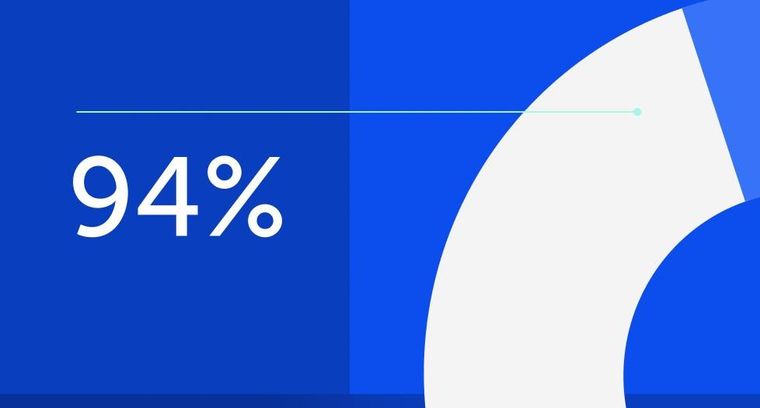
94% of researchers rate our articles as excellent or good
Learn more about the work of our research integrity team to safeguard the quality of each article we publish.
Find out more
EDITORIAL article
Front. Phys., 24 January 2024
Sec. Medical Physics and Imaging
Volume 11 - 2023 | https://doi.org/10.3389/fphy.2023.1356574
This article is part of the Research TopicNovel MRI BiomarkersView all 5 articles
Editorial on the Research Topic
Novel MRI biomarkers
Magnetic Resonance Imaging (MRI) has been available for over 40 years and has made great strides in the diagnosis of numerous pathologies. The advancements in MRI methods, pulse sequences, electronics, radiofrequency (RF) coils, and the improved magnetic fields and gradients, have continuously broadened and deepened the applications of MRI and make it the primary imaging assessment tool for many diseases and an important part in management decisions. MRI biomarkers are specific and measurable characteristics obtained from MRI scans, which can include structural, functional, molecular, or quantitative information, offering important insights into biological processes, diseases, or conditions within the body.
The goal of this Research Topic “Novel MRI Biomarkers” was to provide a platform for researchers to share findings and exchange ideas. The articles featured in this Research Topic contributed unique perspectives and innovative approaches to their fields. Here we summarize these articles in this editorial.
T1ρ, the spin lattice relaxation time in the rotating frame, has been used extensively to probe the relatively slow macromolecular processes, making it a practical tool for gaining information about water spin dynamics and interactions with endogenous macromolecules [1–3]. Studies have indicated that at high static fields (3.0T and beyond), chemical exchange significantly contributes to T1ρ relaxation [4–6]. Therefore, at higher static fields, T1ρ may improve its capability to investigate the content of labile protons associated with macromolecules. The study presented by Liu Y et al. conducted the T1ρ imaging in human brain at ultra-high field (5.0T) and compared the results with 3.0T. They found that there was no significant difference in T1ρ values between 3.0T and 5.0T, but the signal-to-noise ratio (SNR) was significantly improved at 5.0T, indicating the benefits of using 5.0T in investigating neurological disorders. It is worth noting that T1ρ imaging at higher field strengths is prone to image artifacts arising from field inhomogeneities. There have been significant efforts previously to address this Research Topic [7–10]; another Research Topic at higher fields is the high specific absorption rate (SAR) that potentially prolongs the imaging time. The study showed that both issues were manageable at ultra-high field 5.0T.
Chemical exchange-sensitive MRI sequences, such as chemical exchange saturation transfer (CEST) or chemical exchange-sensitive spin-lock (CESL), are MRI techniques used to detect and visualize certain molecules or compounds in biological tissues based on their chemical exchange properties [11–14]. Both CEST and CESL techniques have been used in studying biological systems and which can offer insights into various physiological and pathological conditions. In both CEST and CESL, there is a time delay between the irradiation preparation and the imaging acquisition, during which the T1-relaxation can reduce the chemical exchange contrast and affect the quantification of such methods. The conventional correction method requires a separate T1 map scan to compensate for the T1-relaxation effect [15], but this approach increases the total imaging time. In the paper by Chung and Jin a formula was derived from theoretical analysis to compensate for the T1-relaxation effect. This proposed method involves post-acquisition correction and holds potential for application in other scenarios, such as multi-slice T1-weighted imaging or diffusion-weighted imaging.
Multi-band interleaved EPI (echo-planar imaging) involves the simultaneous excitation of multiple slices in an MRI sequence, allowing for the rapid acquisition of multiple slices in a single imaging volume. This technique improves the speed of image acquisition, enabling faster whole-brain coverage and higher temporal resolution compared to traditional methods. Two-dimensional single-shot EPI (2D-ssEPI) is the typical MRI method used in diffusion and functional MRI because of its rapid acquisition. However, EPI suffers from Nyquist ghost artifacts caused by gradient delay associated with alternating readout polarity [16]. In the study by Liu et al., a robust 2D Nyquist ghost correction method for multi-band interleaved EPI, without the need for a reference scan and iterative calculation, was proposed. This method demonstrates promise in enhancing multiple imaging biomarkers including DWI, DTI, or multi-shot EPI.
Dixon imaging with multi-echo Stack-of-star radial k-space trajectories and golden angle ordering shows promise in fat quantification, specifically the estimation of proton density fat fraction (PDFF). However, imperfections in the gradient chain, such as eddy currents and system delays, might influence radial imaging and distort the estimation of fat fraction. In the work by Zöllner et al., a retrospective trajectory correction method was proposed. This method, based on a simple gradient modulation transfer function (GMTF) measurement, aims to predict and correct k-space trajectory errors induced by the gradient chain. The results indicated that the GMTF-based k-space trajectory correction is a rapid alternative to mitigate PDFF quantitation errors caused by the gradient system. The authors validated this method using 3D radial multi-echo gradient-echo acquisitions.
Although a limited number of articles have been received in this Research Topic, each one offers significant insights into technical improvements addressing important Research Topic in clinical application or has important clinical implication. The future of MRI biomarkers holds immense potential. Through ongoing research and technological innovations, we can anticipate the development of even more sophisticated biomarkers that provide unprecedented levels of insight into disease processes. Moreover, the integration of artificial intelligence and machine learning algorithms is poised to further enhance the utility of MRI biomarkers. These technologies will automate data analysis, reduce diagnostic errors, and enable the creation of predictive models for disease progression and treatment response.
PW: Writing–original draft, Writing–review and editing. WC: Writing–original draft, Writing–review and editing. JG: Writing–original draft, Writing–review and editing.
The author(s) declare that no financial support was received for the research, authorship, and/or publication of this article.
We would like to thank all the authors and reviewers who have participated in this Research Topic.
The authors declare that the research was conducted in the absence of any commercial or financial relationships that could be construed as a potential conflict of interest.
All claims expressed in this article are solely those of the authors and do not necessarily represent those of their affiliated organizations, or those of the publisher, the editors and the reviewers. Any product that may be evaluated in this article, or claim that may be made by its manufacturer, is not guaranteed or endorsed by the publisher.
1. Duvvuri U, Goldberg AD, Kranz JK, Hoang L, Reddy R, Wehrli FW, et al. Water magnetic relaxation dispersion in biological systems: the contribution of proton exchange and implications for the noninvasive detection of cartilage degradation. Proc Natl Acad Sci U S A (2001) 98(22):12479–84. doi:10.1073/pnas.221471898
2. Borthakur A, Wheaton AJ, Gougoutas AJ, Akella SV, Regatte RR, Charagundla SR, et al. In vivo measurement of T1rho dispersion in the human brain at 1.5 tesla. J Magn Reson Imaging (2004) 19(4):403–9. doi:10.1002/jmri.20016
3. Wang YX, Griffith JF, Leung JC, Yuan J. Age related reduction of T1rho and T2 magnetic resonance relaxation times of lumbar intervertebral disc. Quant Imaging Med Surg (2014) 4(4):259–64. doi:10.3978/j.issn.2223-4292.2014.07.14
4. Cobb JG, Xie J, Gore JC. Contributions of chemical and diffusive exchange to T1ρ dispersion. Magn Reson Med (2013) 69(5):1357–66. doi:10.1002/mrm.24379
5. Spear JT, Gore JC. New insights into rotating frame relaxation at high field. NMR Biomed (2016) 29(9):1258–73. doi:10.1002/nbm.3490
6. Wang P, Block J, Gore JC. Chemical exchange in knee cartilage assessed by R1ρ (1/T1ρ) dispersion at 3T. Magn Reson Imaging (2015) 33(1):38–42. doi:10.1016/j.mri.2014.07.008
7. Chen W. Errors in quantitative T1rho imaging and the correction methods. Quant Imaging Med Surg (2015) 5(4):583–91. doi:10.3978/j.issn.2223-4292.2015.08.05
8. Chen W. Artifacts correction for T1rho imaging with constant amplitude spin-lock. J Magn Reson (2017) 274:13–23. doi:10.1016/j.jmr.2016.11.002
9. Witschey WR, Borthakur A, Elliott MA, Mellon E, Niyogi S, Wallman DJ, et al. Artifacts in T1 rho-weighted imaging: compensation for B(1) and B(0) field imperfections. J Magn Reson (2007) 186(1):75–85. doi:10.1016/j.jmr.2007.01.015
10. Pang Y. A self-compensated spin-locking scheme for quantitative R1ρ dispersion MR imaging in ordered tissues. Magn Reson Imaging (2022) 94:112–8. doi:10.1016/j.mri.2022.09.007
11. Chung JJ, Jin T, Lee JH, Kim SG. Chemical exchange saturation transfer imaging of phosphocreatine in the muscle. Magn Reson Med (2019) 81(6):3476–87. doi:10.1002/mrm.27655
12. van Zijl PC, Yadav NN. Chemical exchange saturation transfer (CEST): what is in a name and what isn't? Magn Reson Med (2011);65(4):927–48. doi:10.1002/mrm.22761
13. Jin T, Wang P, Zong X, Kim SG. MR imaging of the amide-proton transfer effect and the pH-insensitive nuclear overhauser effect at 9.4 T. Magn Reson Med (2013) 69(3):760–70. doi:10.1002/mrm.24315
14. Jiang B, Jin T, Blu T, Chen W. Probing chemical exchange using quantitative spin-lock R1ρ asymmetry imaging with adiabatic RF pulses. Magn Reson Med (2019) 82(5):1767–81. doi:10.1002/mrm.27868
15. Sun PZ, Murata Y, Lu J, Wang X, Lo EH, Sorensen AG. Relaxation-compensated fast multislice amide proton transfer (APT) imaging of acute ischemic stroke. Magn Reson Med (2008) 59(5):1175–82. doi:10.1002/mrm.21591
Keywords: magnetic resoance imaging (MRI), imaging biomakers, T1rho imaging, chemical exchange saturation transfer (CEST), mDixon MR, chemical exchange-sensitive spin-lock (CESL)
Citation: Wang P, Chen W and Gao J (2024) Editorial: Novel MRI biomarkers. Front. Phys. 11:1356574. doi: 10.3389/fphy.2023.1356574
Received: 15 December 2023; Accepted: 19 December 2023;
Published: 24 January 2024.
Edited and reviewed by:
Ewald V. Moser, Medical University of Vienna, AustriaCopyright © 2024 Wang, Chen and Gao. This is an open-access article distributed under the terms of the Creative Commons Attribution License (CC BY). The use, distribution or reproduction in other forums is permitted, provided the original author(s) and the copyright owner(s) are credited and that the original publication in this journal is cited, in accordance with accepted academic practice. No use, distribution or reproduction is permitted which does not comply with these terms.
*Correspondence: Ping Wang, cGluZy53YW5nQGJhcnJvd25ldXJvLm9yZw==; Weitian Chen, d3RjaGVuQGN1aGsuZWR1Lmhr; Jiahong Gao, amdhb0Bwa3UuZWR1LmNu
Disclaimer: All claims expressed in this article are solely those of the authors and do not necessarily represent those of their affiliated organizations, or those of the publisher, the editors and the reviewers. Any product that may be evaluated in this article or claim that may be made by its manufacturer is not guaranteed or endorsed by the publisher.
Research integrity at Frontiers
Learn more about the work of our research integrity team to safeguard the quality of each article we publish.