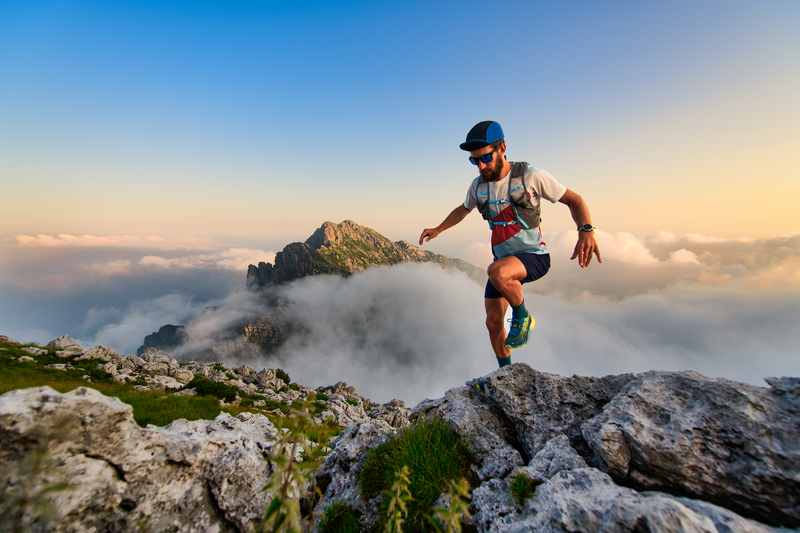
95% of researchers rate our articles as excellent or good
Learn more about the work of our research integrity team to safeguard the quality of each article we publish.
Find out more
REVIEW article
Front. Phys. , 15 September 2023
Sec. Nuclear Physics
Volume 11 - 2023 | https://doi.org/10.3389/fphy.2023.1242668
This article is part of the Research Topic Clustering in Light Nuclei: Current Research, New Aspects, Challenges and Perspectives View all 7 articles
The structure of the unbound nucleus 13Be is important for understanding the Borromean, two-neutron halo nucleus 14Be. The experimental studies conducted over the last four decades are reviewed in the context of the beryllium chain of isotopes and some significant theoretical studies. The focus of this paper is the comparison of new data from a 12Be(d,p) reaction in inverse kinematics, which was analyzed using Geant4 simulations and a Bayesian fitting procedure, with previous measurements. Two possible scenarios to explain the strength below 1 MeV above the neutron separation energy were proposed in that study: a single p-wave resonance or a mixture of an s-wave virtual state with a weaker p- or d-wave resonance. Comparisons of recent invariant mass and the (d,p) experiments show good agreement between the transfer measurement and the two most recent high-energy nucleon removal measurements.
With just four protons, the particle-bound members of the beryllium chain of isotopes stretch from 7Be (N/Z = 0.75) on the proton-rich side of stability to 14Be (N/Z = 2.5), the two-neutron halo on the neutron-rich side. Adding a single neutron to 7Be results in the 8Be system of two α particles which is unbound by only 92 keV. Adding a second neutron produces 9Be, the only beryllium isotope that is stable against β decay. This stability is a product of two phenomena that occur across the beryllium chain of isotopes, molecular structures [1]; [2]; [3], and core excitation [4]. The molecular structure is closely connected to the Borromean nature of 9Be; the three-body system of α − α − n is bound despite the two-body subsystems, α − n and α − α, being unbound. The delocalized neutron in 9Be can be viewed as being exchanged between α particles [5]. Analogous to atomic molecules, the neutron in the ground state of 9Be is well understood as being in a π-type orbital, whereas the first excited state may be better described with a neutron in the σ-type orbital. These molecular orbits are intimately related to the prolate nature of the two-α cluster structure [6].
The cluster structure appears to weaken in 10Be, as evidenced by the reduced size of its charge radius [7]; [8]. The two-α plus two-neutron structure is more apparent in excited states closer to the α and neutron separation energies, such as the isomeric second 0+ state at Ex = 6.179 MeV, which has a small γ branch to the more compact first 2+ state [5].
Adding another neutron makes the archetypal one-neutron halo nucleus 11Be. A 16% core-excited component in the ground state of 11Be was required to reproduce the results from the 11Be(p,d)10Be reaction [9]. This is less than that of 9Be, where the core-excited component was calculated as approximately half of the ground-state wave function [4]. Additionally, dynamical core excitation needs to be included in the calculations of both transfer [10] and breakup [11] reactions.
The parity inversion in 11Be, where the 1/2+ ground state decreases 320 keV below the only other bound state with Jπ = 1/2−, along with larger collectivity in 10Be, led to the questioning about the robustness of the N = 8 shell closure at 12Be. Using a three-body model with core excitation, Nunes et al. were able to show an increased sphericity in the core 10Be within 12Be compared to that observed in 11Be [12]. This in turn led to greater mixing between p- and sd-shell valence neutron states and a melting of the N = 8 shell closure. The coupling of a d-wave neutron with the excited 2+ 10Be core severely restricts the formation of a halo in 12Be [13]. Notably, 12Be is not Borromean, as the n-10Be system is bound but is still well described by three-body models.
The N = 10 isotope 14Be presents the heaviest particle-stable beryllium isotope. The naive shell model would predict a d5/2-dominated ground-state wave function for 14Be. However, with the level inversion seen in the other neutron-rich beryllium isotopes, some low-lying s1/2 strength is expected. With two neutrons in the halo and with significant ℓ > 0 components of the wave function, the halo of 14Be is much more contained than that of 11Be, despite being closer to the drip line. It would seem natural to study 14Be in a three-body model, with 12Be as the core and two valence neutrons [14]; [15]. Thompson and Zhukov found that adding an s-wave virtual state below the well-known
Beyond the neutron drip line, 15Be has been observed to decay to 12Be through unbound states in 14Be [18]. The last isotope to be observed is 16Be, which is bound with respect to one neutron emission, but unbound to the emission of two neutrons [19]. The two neutrons from the decay were observed in a small emission angle.
Theoretical studies of 13Be have used the shell model [20] or a potential model [21], the Nilsson model [22], microscopic cluster models [23], antisymmetrized molecular dynamics [24], and relativistic mean-field theory [25]. As shown in Table 1, with the exception of the earliest work, the calculations agree on the existence of a 5/2+ resonance between 2 and 2.5 MeV above the neutron threshold. However, the location of the ground state relative to the neutron threshold is disputed, as is the parity of the ground state. There have also been reaction theory studies of, and comparing to, experimental data, for example, the work of Bonaccorso [26] and references therein. Casal et al. [27] used a transfer to the continuum model including deformation in the 12Be + n potential, following the prescription in Thompson et al. [28], to interpret the data obtained from Corsi et al. [29]. This work indicates a p-wave resonance at between 0.4 and 0.5 MeV above the threshold.
TABLE 1. Energy (and Jπ assignments) of low-lying states in 13Be according to a selection of theoretical studies.
There have been many experiments on 13Be since the first discovery of a resonance [31] at 1.8 MeV above the neutron threshold. Some of the experimental results from the last four decades are shown in Table 2. The reactions used to probe the structure of halo nuclei can be broadly divided into missing mass and invariant mass techniques. Transfer reactions, where excitation energies are found from the reaction Q-values, fall into the missing mass category. Knockout, breakup, and Coulomb dissociation, where the final state is reconstructed from two or more fragments, represent invariant mass techniques. In the case of 13Be, the fragments are 12Be and a neutron. The early measurements, e.g., [31]; [30]; [32]; and [33], mostly populated 13Be through multinucleon transfer reactions. An exception is the 12Be(d,p) experiment performed in [34] at RIKEN. At a beam energy of 55 AMeV, the conditions are not well matched to observe low ℓ transfer, and the carbon in the target largely masked any structure below 2 MeV. Indeed, none of the experiments before the fragmentation experiment at the National Superconducting Cyclotron Laboratory [35] revealed any structure below the
TABLE 2. Previous studies of the low-lying structure of 13Be, up to approximately 2.5 MeV above the neutron threshold.
Most of the more recent experiments used invariant mass techniques at energies between 40 and 400 AMeV. Of these, all except one employed nucleon removal, either a neutron from 14Be [37]; [38]; [39]; and [29] or a proton from 14B [36]; [40]; and [42]. In [41], a nucleon exchange reaction was used to populate unbound states in 13Be from a 13B beam. Spectra from invariant mass methods contain information relating to the initial state of the target (the beam in an inverse kinematics reaction) and reflect final state interactions between outgoing fragments. There can also be complications relating to the reaction mechanism, which can be diffractive or absorptive.
The most recent experiment, [44]; [43], used a 12Be beam from ISAC-II and the solid deuterium target and detector system IRIS [45] to perform a one-neutron transfer reaction. The advantages of using the (d,p) reaction at low energy (Ebeam = 9.5 AMeV) are that the energy and angular momentum-matching conditions are ideal for populating low-ℓ, near-threshold states. The non-Gaussian experimental response caused by increasingly poor resolution for lower-energy protons exiting the solid deuterium target necessitated an analysis technique that included both simulation and Bayesian fitting methods, as demonstrated in [46]. The initial analysis included the
A comparison of the transfer measurement (for the case assuming a single state below 2 MeV) with some recent invariant mass measurement results is shown in Figure 1. Discrepancies clearly persist in the low-lying structure of 13Be, even between recent measurements. The
FIGURE 1. Comparison of the low-lying structure in 13Be according to recent invariant mass measurements [38]; [40]; [42]; [29] and a recent transfer reaction measurement [43]. Only the case with a pure p-wave is shown for [43], as the position of the waves in the mixtures could not be resolved. Red, blue, and green lines depict s, p, and d waves, respectively. Red-dashed lines show the threshold. Gray-shaded region shows the presence of a virtual state.
The mixed case in [43] is not shown in Figure 1 as the ordering of the s- and p-wave strength cannot be extracted from the data, only the relative intensities. The lowest χ2/NDF was found for a mixture of
To make a more robust comparison between the recent invariant-mass measurements and the transfer reaction, the resonance parameters extracted from [38], [29], [40], and [42] were used as inputs for the Geant4 [47] simulation, as shown in Figure 2. The centroid energies and widths from the analyses were used, and the relative intensities of the resonances and virtual states were fitted as free parameters. A relatively poor fit to the data from the 12Be(d,p) reaction experiment was produced from the simulations using resonance parameters from [38] (χ2/NDF = 5.51). Using the parameters from [40] provided a better fit (χ2/NDF = 3.47), and those from [39] (not shown) provided a fit with χ2/NDF = 3.18. The parameters that provided the best fit from the literature were those from [29] (χ2/NDF = 2.62), closely followed by those from [42] (χ2/NDF = 2.76). These can all be compared to the Bayesian fit of the data with a single p-wave resonance along with the well-known d-wave resonance of approximately 2 MeV. This fit allowed the locations and widths of the two resonances to vary along with the intensities. The parameters shown in Table 2 resulted in a χ2/NDF of 2.02. The single p wave below the 2 MeV
FIGURE 2. Data fitted with Geant4 simulations with energy and widths obtained from (A) [38], (B) [29], (C) [40], and (D) [42]. Amplitudes of the states were used from the angular distributions. Global fit is shown as the red line, and the background is denoted as black dots. Lowest-lying strength is shown as a blue-dashed line irrespective of its nature. Higher-lying states are depicted as solid brown and green dot–dashed lines.
The beryllium chain of isotopes displays various clustering phenomena including molecular structures and one- and two-neutron halos in 11Be and 14Be, respectively. The isotope 13Be is an unbound subsystem of the Borromean nucleus 14Be. Its structure has been investigated experimentally for 40 years using both missing mass and invariant mass techniques. However, with the exception of the 2 MeV
KJ was the spokesperson for the new 12Be + 2H experiment and wrote the first draft of this manuscript. JK analyzed the data and produced the figures from the new 12Be + 2H experiment. RK was the co-spokesperson for the new 12Be + 2H experiment and was instrumental in the design and running of the experiment. All authors contributed to the article and approved the submitted version.
This research was supported by the U.S. Department of Energy, the Office of Science, the Office of Nuclear Physics under contract Nos DE-FG02-96ER40963 (UTK). The authors are grateful for the support from the NSERC, Canada Foundation for Innovation and Nova Scotia Research and Innovation Trust, and RCNP, the grant-in-aid program of the Japanese government. TRIUMF is supported by a contribution from the National Research Council, Canada.
The authors would like to acknowledge the collaborators on the TRIUMF 12Be + 2H experiment and the beam delivery team for providing the 12Be beam, and DE-AC05-00OR22725 (ORNL), and the U. S. National Science Foundation under Award Numbers PHY-1404218 (Rutgers) and PHY-2011890 (Notre Dame). This work was supported by the National Research Foundation of Korea (NRF) grant funded by the Korean government (MSIT) grant nos 2020R1A2C1005981 and 2016R1A5A1013277. This work was partially supported by STFC grant no. ST/L005743/1 (Surrey).
The authors declare that the research was conducted in the absence of any commercial or financial relationships that could be construed as a potential conflict of interest.
All claims expressed in this article are solely those of the authors and do not necessarily represent those of their affiliated organizations, or those of the publisher, the editors, and the reviewers. Any product that may be evaluated in this article, or claim that may be made by its manufacturer, is not guaranteed or endorsed by the publisher.
1. von Oertzen W. Two-center molecular states in 9B, 9Be, 10Be, and 10B. Z Phys (1996) 354:37–43. doi:10.1007/s002180050010
2. von Oertzen W. Dimers based on the α + α potential and chain states of carbon isotopes. Z Phys (1997) 357:355–65. doi:10.1007/s002180050255
3. Seya M, Kohno M, Nagata S. Nuclear binding mechanism and structure of neutron-rich Be and B isotopes by molecular-orbital model. Prog Th Phys (1981) 65:204–23. doi:10.1143/PTP.65.204
4. Parfenova Y, Leclercq-Willain C. Hyperfine anomaly in be isotopes in the cluster model and the neutron spatial distribution. Phys Rev C (2005) 72:024312. doi:10.1103/PhysRevC.72.024312
5. Freer M, Horiuchi H, Kanada-En’yo Y, Lee D, Meißner U-G. Microscopic clustering in light nuclei. Rev Mod Phys (2018) 90:035004. doi:10.1103/RevModPhys.90.035004
6. Canavan R, Freer M. Demonstration of the universality of molecular structures in prolate deformed nuclei. J Phys G (2020) 47:095102. doi:10.1088/1361-6471/ab96b3
7. Nörtershäuser W, Tiedemann D, Žáková M, Andjelkovic Z, Blaum K, Bissell ML, et al. Nuclear charge radii of 7,9,10Be and the one-neutron halo nucleus 11Be. Phys Rev Lett (2009) 102:062503. doi:10.1103/PhysRevLett.102.062503
8. Krieger A, Blaum K, Bissell ML, Frömmgen N, Geppert C, Hammen M, et al. Nuclear charge radius of 12Be. Phys Rev Lett (2012) 108:142501. doi:10.1103/PhysRevLett.108.142501
9. Fortier S, Pita S, Winfield J, Catford W, Orr N, de Wiele V, et al. Core excitation in 11Begs via the p(11Be,10Be)d reaction. Phys Lett (1999) B461:22–7. doi:10.1016/S0370-2693(99)00825-4
10. Deltuva A, Ross A, Norvaišas E, Nunes FM. Role of core excitation in (d, p) transfer reactions. Phys Rev C (2016) 94:044613. doi:10.1103/PhysRevC.94.044613
11. Moro AM, Crespo R. Core excitation effects in the breakup of the one-neutron halo nucleus 11be on a proton target. Phys Rev C (2012) 85:054613. doi:10.1103/PhysRevC.85.054613
12. Nunes FM, Thompson IJ, Tostevin JA. Core excitation in 12Be. Nucl Phys (2002) A703:593–602. doi:10.1016/S0375-9474(01)01667-0
13. Nunes FM. Valence pairing, core deformation and the development of two-neutron halos. Nucl Phys (2005) A757:349–59. doi:10.1016/j.nuclphysa.2005.04.005
14. Descouvemont P. Halo structure of 14Be in a microscopic 12Be+n+n cluster model. Phys Rev C (1995) 52:704–10. doi:10.1103/PhysRevC.52.704
15. Thompson IJ, Zhukov MV. Structure and reactions of the 12,14Be nuclei. Phys Rev C (1996) 53:708–14. doi:10.1103/PhysRevC.53.708
16. Labiche M, Marqués FM, Sorlin O, Vinh Mau N. Structure of 13Be and 14Be. Phys Rev C (1999) 60:027303. doi:10.1103/PhysRevC.60.027303
17. Vinh Mau N, Pacheco JC. Structure of the 11Li nucleus. Nucl Phys (1996) A607:163–77. doi:10.1016/0375-9474(96)00246-1
18. Spyrou A, Smith JK, Baumann T, Brown BA, Brown J, Christian G, et al. Search for the 15be ground state. Phys Rev C (2011) 84:044309. doi:10.1103/PhysRevC.84.044309
19. Spyrou A, Kohley Z, Baumann T, Bazin D, Brown BA, Christian G, et al. First observation of ground state dineutron decay: 16Be. Phys Rev Lett (2012) 108:102501. doi:10.1103/PhysRevLett.108.102501
20. Poppelier N, Wood L, Glaudemans P. Properties of exotic p-shell nuclei. Phys Lett B (1985) 157:120–2. doi:10.1016/0370-2693(85)91529-1
21. Fortune HT. Update on energies and widths in 13Be. Phys Rev C (2019) 99:014304. doi:10.1103/PhysRevC.99.014304
22. Macchiavelli AO, Crawford HL, Campbell CM, Clark RM, Cromaz M, Fallon P, et al. Analysis of spectroscopic factors in 11Be and 12Be in the Nilsson strong-coupling limit. Phys Rev C (2018) 97:011302. doi:10.1103/PhysRevC.97.011302
23. Descouvemont P. Evidence for particle stability of 13Be in a microscopic cluster model. Phys Lett B (1994) 331:271–5. doi:10.1016/0370-2693(94)91050-2
24. Kanada-En’yo Y. Breaking of N=8 magicity in 13Be. Phys Rev C (2012) 85:044320. doi:10.1103/PhysRevC.85.044320
25. Ren Z, Chen B, Ma Z, Xu G. Level inversion of N=9 isotones in the relativistic mean-field theory. Z Phys A (1997) 357:137–41. doi:10.1007/s002180050228
26. Bonaccorso A. Unbound nuclei studied by projectile fragmentation. Physica Scripta (2012) 2012:014008. doi:10.1088/0031-8949/2012/t150/014008
27. Casal J, Gómez-Ramos M, Moro A, Corsi A. Exploring continuum structures in reactions with three-body nuclei. J Phys Conf Ser (2020) 1643, 012075. doi:10.1088/1742-6596/1643/1/012075
28. Thompson I, Nunes F, Danilin B. FaCE: A tool for three body faddeev calculations with core excitation. Comp Phys Commun (2004) 161:87–107. doi:10.1016/j.cpc.2004.03.007
29. Corsi A, Kubota Y, Casal J, Gómez-Ramos M, Moro A, Authelet G, et al. Structure of 13Be probed via quasi-free scattering. Phys Lett B (2019) 797:134843. doi:10.1016/j.physletb.2019.134843
30. Ostrowski AN, Bohlen HG, Demyanova AS, Gebauer B, Kalpakchieva R, Langner C, et al. Mass spectroscopy of 13Be. Z Phys (1992) A343:489–90. doi:10.1007/BF01289828
31. Aleksandrov DV, Ganza EA, Glukhov YA. Observation of the isotope 13Be in the reaction 14C(7Li, 8B). Sov J Nucl Phys (1983) 37:474–5.
32. von Oertzen W, Bohlen H, Gebauer B, von Lucke-Petsch M, Ostrowski A, Seyfert C, et al. Nuclear structure studies of very neutron-rich isotopes of 7−−10He, 9−11Li and 12−14Be via two-body reactions. Nucl Phys A (1995) 588:c129–34. Proceedings of the Fifth International Symposium on Physics of Unstable Nuclei. doi:10.1016/0375-9474(95)00111-D
33. Belozyorov A, Kalpakchieva R, Penionzhkevich Y, Dlouhý Z, Piskor S, Vincour J, et al. Spectroscopy of 13Be. Nucl Phys A (1998) 636:419–26. doi:10.1016/S0375-9474(98)00217-6
34. Korsheninnikov A, Nikolskii E, Kobayashi T, Aleksandrov D, Fujimaki M, Kumagai H, et al. Spectroscopy of 12Be and 13Be using a 12Be radioactive beam. Phys Lett B (1995) 343:53–8. doi:10.1016/0370-2693(94)01435-F
35. Thoennessen M, Yokoyama S, Hansen PG. First evidence for low lying s-wave strength in 13Be. Phys Rev C (2000) 63:014308. doi:10.1103/PhysRevC.63.014308
36. Lecouey JL. Experimental studies of unbound neutron-rich nuclei. Few-Body Syst (2004) 34:21–6. doi:10.1007/s00601-004-0029-3
37. Simon H, Meister M, Aumann T, Borge M, Chulkov L, Pramanik UD, et al. Systematic investigation of the drip-line nuclei 11Li and 14Be and their unbound subsystems 10Li and 13Be. Nucl Phys A (2007) 791:267–302. doi:10.1016/j.nuclphysa.2007.04.021
38. Kondo Y, Nakamura T, Satou Y, Matsumoto T, Aoi N, Endo N, et al. Low-lying intruder state of the unbound nucleus 13Be. Phys Lett B (2010) 690:245–9. doi:10.1016/j.physletb.2010.05.031
39. Aksyutina Y, Aumann T, Boretzky K, Borge MJG, Caesar C, Chatillon A, et al. Structure of the unbound nucleus 13Be: One-neutron knockout reaction data from 14Be analyzed in a holistic approach. Phys Rev C (2013) 87:064316. doi:10.1103/PhysRevC.87.064316
40. Randisi G, Leprince A, Al Falou H, Orr NA, Marqués FM, Achouri NL, et al. Structure of 13Be probed via secondary-beam reactions. Phys Rev C (2014) 89:034320. doi:10.1103/PhysRevC.89.034320
41. Marks BR, DeYoung PA, Smith JK, Baumann T, Brown J, Frank N, et al. Population of 13Be in a nucleon exchange reaction. Phys Rev C (2015) 92:054320. doi:10.1103/PhysRevC.92.054320
42. Ribeiro G, Nácher E, Tengblad O, Díaz Fernández P, Aksyutina Y, Alvarez-Pol H, et al. Structure of 13Be studied in proton knockout from 14B. Phys Rev C (2018) 98:024603. doi:10.1103/PhysRevC.98.024603
44. Kovoor JM. Study of one-nucleon transfer reactions. Phd dissertation. Knoxville: University of Tennessee (2022).
45. Kanungo R. Iris: The ISAC charged particle reaction spectroscopy facility for reaccelerated high-energy ISOL beams. Hyperfine Interactions (2014) 225:235–40. doi:10.1007/s10751-013-0904-8
46. Hooker J, Kovoor J, Jones K, Kanungo R, Alcorta M, Allen J, et al. Use of Bayesian Optimization to understand the structure of nuclei. Nucl Instrum Methods B (2022) 512:6–11. doi:10.1016/j.nimb.2021.11.014
Keywords: direct reactions, clustering, beryllium, 13Be, 12Be
Citation: Jones KL, Kovoor J and Kanungo R (2023) Status of experimental knowledge on the unbound nucleus 13Be. Front. Phys. 11:1242668. doi: 10.3389/fphy.2023.1242668
Received: 19 June 2023; Accepted: 28 August 2023;
Published: 15 September 2023.
Edited by:
Valdir Guimaraes, University of São Paulo, BrazilReviewed by:
Zilong Chang, Indiana University, United StatesCopyright © 2023 Jones, Kovoor and Kanungo. This is an open-access article distributed under the terms of the Creative Commons Attribution License (CC BY). The use, distribution or reproduction in other forums is permitted, provided the original author(s) and the copyright owner(s) are credited and that the original publication in this journal is cited, in accordance with accepted academic practice. No use, distribution or reproduction is permitted which does not comply with these terms.
*Correspondence: K. L. Jones, a2dyenl3YWNAdXRrLmVkdQ==
Disclaimer: All claims expressed in this article are solely those of the authors and do not necessarily represent those of their affiliated organizations, or those of the publisher, the editors and the reviewers. Any product that may be evaluated in this article or claim that may be made by its manufacturer is not guaranteed or endorsed by the publisher.
Research integrity at Frontiers
Learn more about the work of our research integrity team to safeguard the quality of each article we publish.