- 1Ceyear Technologies Co., Ltd., Qingdao, China
- 2Science and Technology on Electronic Test & Measurement Laboratory, Qingdao, China
- 3School of Electronic Engineering, Xidian University, Xi’an, China
- 4School of Information Science and Engineering, Shandong University, Qingdao, China
A high side-mode suppression ratio external-cavity wavelength-swept laser system with a wide mode-hopping free tuning range is developed. The proposed wavelength-swept laser is based on a single-angled facet gain chip in a classical Littman-Metcalf external-cavity configuration using a blazed diffraction grating as the feedback element. The output power, side-mode suppression ratio, and tunable range of the external-cavity wavelength-swept laser system are experimentally measured. Wide mode-hopping-free continuous wavelength tuning range about 100 nm in CL-band with a side-mode suppression ratio of more than 65.64 dB are realized. An output optical power of more than 14.12 dBm over the entire tunable region can also be achieved. The proposed external-cavity wavelength-swept laser system allows simultaneously high side-mode suppression ratio and no mode-hopping tunable radiation, thus opening a door for practical applications such as optical modulation schemes.
1 Introduction
The highly controllable emission properties of wavelength-swept lasing sources are prior selected semiconductor lasers for a vast array of applications in fields including high-resolution spectroscopy [1,2], laser cooling [3], coherent optical orthogonal frequency division multiplexing (CO-OFDM) modulation schemes [4], space strontium optical clock [5–7], and optical frequency-domain reflectometry (OFDR) and so on [8–12]. Across these fields, the wavelength-swept lasing sources with higher side-mode suppression ratios (SMSRs) and increased mode-hopping free (MHF) tuning ranges continue to enable new applications. Therefore, it is critical to building a continuous wavelength-swept laser system with high SMSR.
Several types of wavelength-swept lasers, such as external-cavity semiconductor lasers (ECSLs), dye lasers, distributed Bragg reflector lasers, tunable solid-state lasers, vertical-cavity surface-emitting lasers, distributed feedback lasers, and optical non-linear frequency conversion-based lasers have been developed in the last 50 years [13–21]. Compared to other types of wavelength-swept laser, ECSL has the prestigious characteristics of a large tuning range, high signal-to-source spontaneous emission ratio, narrow linewidth, and low cost, which is one of the most versatile measuring tools [22–24]. An external-cavity diode laser system with a maximum SMSR up to 50 dB at 1550 nm has been demonstrated by K. Fedorova et al., with a 173 nm tunable range of the proposed wavelength-swept laser system [25]. Using an InGaAsP/InP multiple quantum well (MQW) gain chip, an external-cavity diode laser configuration with a spectral range of 1320–1562 nm was achieved, where the SMSR covers the 17 dB–42 dB range [26]. Yuan et al. showed an extended-cavity InAs/InP quantum-dot (QD) laser operating at pulsed injection current with a maximum SMSR of around 20 dB, where the reported wavelength-swept laser system had a tuning range of 190 nm [27]. A high SMSR external-cavity laser scheme based on traditional Littman design was demonstrated by Gong et al., with a maximum MHF range of 78 GHz [28]. A narrow linewidth external-cavity laser scheme was exhibited operating in C-band (1528.77–1568.36 nm in vacuum) by Zhang et al., with an output power of around 13 dBm and with a SMSR around 55 dB [29]. Another C/L-band wavelength-tunable external cavity laser was demonstrated by K. Kasai et al., with a spectral range of 1530–1570 nm or 1570–1610 nm without mode hopping and with a SMSR around 46 dB or 49 dB [30]. Recently, Wang et al. fabricated an ultra-high SMSR ECSL with a wide tunable range around 1550 nm, where a maximum wavelength tuning range of 209.9 nm was obtained, and the SMSR reached 65 dB [31]. Although many attempts focused on the widely tunable external-cavity laser configuration, this may not be the critical issue in many practical applications (typically in telecommunications) where the higher SMSR and the continuous tuning characteristics with MHF need to be paid more attention to.
In this work, based on an antireflection-coated single-angled facet gain chip in a Littman-Metcalf external cavity, a high-SMSR wavelength-swept laser with a CL-band (1520–1620 nm) MHF tuning range is experimentally demonstrated. By adjusting the length of the external cavity applied to the proposed configuration, a single mode tuning range of 100 nm without mode-hopping is obtained. The output power of the wavelength-swept laser is around 15.84 dBm with an injected current of 410 mA at the emitting wavelength of 1550 nm, and output power of more than 14.12 dBm over the entire tuning range is achieved. Meanwhile, the SMSR is more than 65.64 dB through the tuning range. The advantage of this proposed external-cavity wavelength-swept laser is that the lasing wavelength can be tuned finely in a range of C-band and L-band without mode-hoping, thus opening a door for practical application such as coherent optical modulation schemes.
2 Configuration of the wavelength-swept laser
A schematic drawing of the proposed wavelength-swept laser setup is shown in Figure 1. The customized single-angled facet gain chip is housed in a heat-sink with Peltier elements (or called thermoelectric cooler) attached to it is coated with an anti-reflection (0.005%) film on its angled facet and a reflectivity (10%) film on its normal facet. The temperature and the injection current of the single-angled facet gain chip are controlled with a homemade laser diode driver. The running temperature of the gain chip is typically stabilized at 25.0°C. The cavity length of the gain chip is 900 μm. A molded aspherical lens (NA = 0.55) with a focal length of 4.51 mm (Edmund, 87155) is applied to collimate the output continuous wave (CW) light from the gain chip. A gold-coated 900 grooves/mm blazed diffraction grating (Newport Richardson, 33025FL01-155R), with an average diffraction efficiency of 75%, is attached to the tilting face of the homemade mount. The collimated output beam is incident on the surface of the blazed diffraction grating. Depending on the incident angle of the light coming from the gain chip, a particular wavelength will be retroreflected from the gold-coated cavity mirror back into the gain chip to form the resonator. Tuning of the resonating wavelength is realized by rotating the gold-coated cavity mirror driven by a piezoelectric stack actuator with respect to the incident beam. The output of the lasing wavelength from the gain chip to the fiber is collected using a molded aspherical lens, an isolator and a single mode fiber pigtailed collimator, the coupling efficiency is higher than 50%. To measure the output lasing parameters, the output is picked out by an optical switch (OS) with low insertion loss. One channel of the OS is directed to the 3-dB optical coupler (OC), one port of the OC is directed into an optical spectrum analyzer (Yokogawa, AQ6370D) with a resolution of 0.02 nm for measuring the spectral changes, the other port is monitored by a wavelength meter (Yokogawa, AQ6151B), which is adapted to record the MHF traces over the entire tuning range. The other channel of the OS is connected to the calibrated optical power meter (Thorlabs, PM100D) to measure the output power of the lasing wavelength. It is worth noting that the overall external-cavity length from the gain chip output facet to the tuning mirror front facet is around 50 mm. The corresponding longitude mode space of the proposed wavelength-swept laser is equal to 23.1 pm (3 GHz) operating at 1520 nm, which will decide the maximum MHF tuning range theoretically.
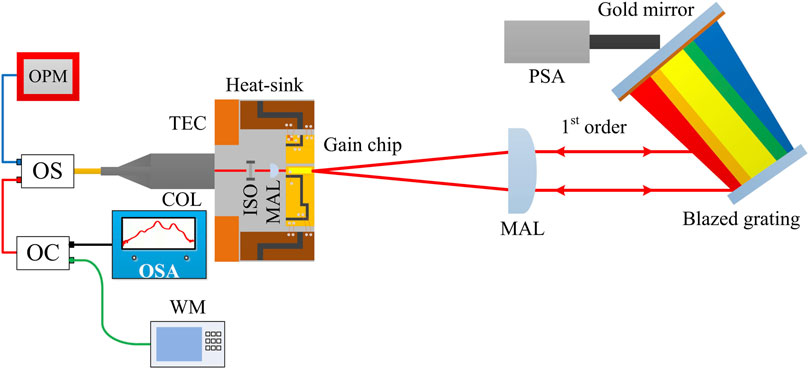
FIGURE 1. Schematic drawing of the proposed wavelength-swept laser setup built in the Littman-Metcalf configuration. OPM, optical power meter; OS, optical switch; TEC, thermoelectric cooler; MAL, molded aspherical lens; PSA, piezoelectric stack actuator; OC, optical coupler; OSA, optical spectrum analyzer; WM: wavelength meter; COL, collimator; ISO, isolator.
3 Characterization and discussion
First, the typical P-I curves of the single-angled facet gain chip with and without an external cavity (namely, free-running) are measured and highlighted in Figures 2A, B, respectively. As can be seen in Figure 2A, since the single-angled facet gain chip is coated with the anti-reflection film, the maximum output power is around 0.14 mW at an injection current of 410 mA. The inset shown in Figure 2A show the gain spectra of the free-running single angled facet gain chip measured using the OSA with resolution of 0.02 nm. The results show the central wavelength is around 1510 nm. When the injection current is increased, the central wavelength slightly decreases along with an obvious increase in the spectra power at two edges of the gain spectra. In fact, by increasing the injection current, the output power at the edges of the full tuning range (1520 nm–1620 nm) of the external-cavity wavelength-swept laser can also be obviously increased.
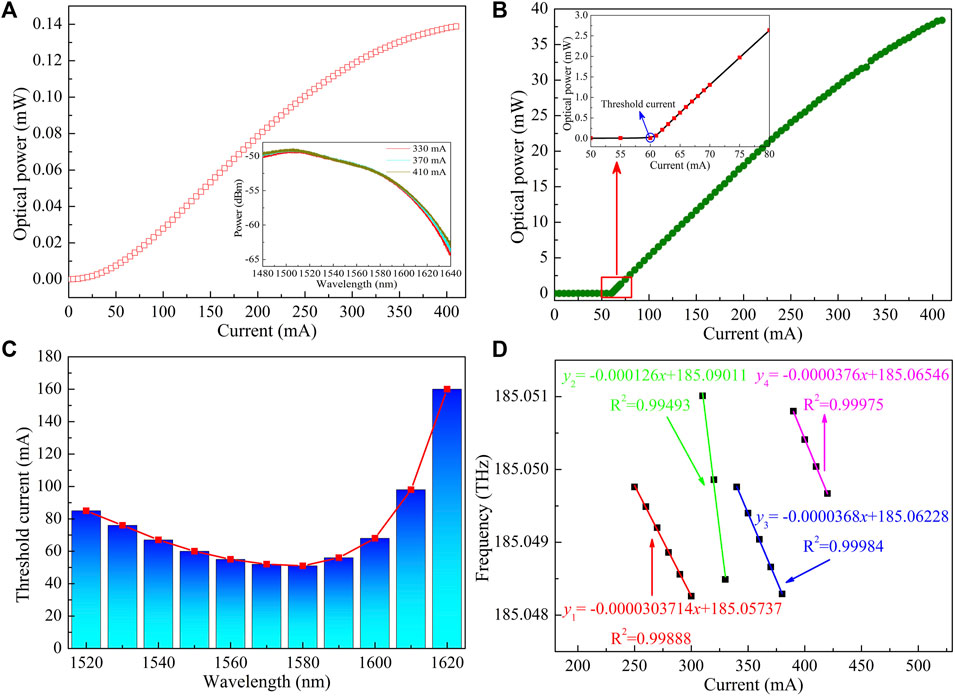
FIGURE 2. (A) Optical output power versus the injection current for the free-running single angled facet gain chip. Inset: gain spectra of the gain chip at different injection currents. (B) Measurement of the output power characteristics of the external-cavity wavelength-swept laser as a function of the injection current operating 1550 nm at a fixed temperature of 25.0°C. (C) Threshold current of the proposed external-cavity wavelength-swept laser at different lasing wavelength. (D) Measurement lasing frequency shift as a function of the injection current in the proposed external-cavity configuration.
After introducing optical feedback to the gain chip, as shown in Figure 2B, the threshold current of the proposed external-cavity wavelength-swept laser is around 60 mA, and the slope efficiency is about 0.11 mW/mA. Meanwhile, an output power of 15.84 dBm is achieved with an injection current of around 410 mA which is currently limited by the homemade laser diode driver. The output power of the gain chip based external cavity laser is 274.3 times as large as the output power of the freely running gain chip. The corresponding experimental result indicates that the external-cavity wavelength-swept laser of the presented configuration runs in a strong optical feedback condition and completes optimum alignment.
The threshold current performance of the presented external-cavity wavelength-swept laser system is characterized by measuring the output optical power at different lasing wavelengths with the same calibrated optical power meter. The results for the threshold current as a function of lasing wavelength are plotted in Figure 2C, demonstrating a maximum threshold current of 160 mA. It can be seen that the threshold current of the proposed wavelength-swept laser system decreases at an intermediate tuning range (1560–1580 nm) and increases significantly when the lasing wavelength is changed toward the short wavelength (1520 nm) or long wavelength (1610 nm). Practically, except for the wavelength-dependent loss (i.e., the diffraction efficiency of the grating) of the cavity, this threshold current performance mainly comes from the spectral gain properties of the single-angled facet gain chip. To achieve a large range of wavelength tunable output, the working current therefore should be set above the maximum threshold current displayed in Figure 2C. Then, the effect of injection current (more than the maximum threshold current) on the tuning characteristics of the presented laser configuration output frequency (wavelength) is investigated. The injection current is increased from 250 mA to 420 mA with 10 mA/step. It can be observed in Figure 2D that, as mentioned in other ECTLs [5,32,33], the proposed external-cavity wavelength-swept laser also has a mode-hopping free current interval. The lasing frequency as a function of the injection current at a controlled laser temperature of 25.0°C is shown in Figure 2D, where linear regression is conducted to achieve the frequency tuning rate of the injection current, respectively, the mode-hopping is observed at an injection current of 300 mA–310, 330–340, and 390–400 mA. From this, the frequency tuning rate of the injection current can be determined to be approximately 0.0304 GHz/mA, 0.126 GHz/mA, 0.0368 GHz/mA and 0.0376 GHz/mA, respectively. Therefore, it is critical to choose a perfect working point to avoid this mode-hopping interval when the external-cavity wavelength-swept laser is working.
Subsequently, the basic tunability of the proposed external-cavity wavelength-swept laser system is characterized by an injection current of 410 mA. Figure 3A shows the measured spectra of the wavelength-swept laser. The resonance wavelength is increased from 1519.98 nm to 1619.98 nm with about 10 nm/step. It can be seen in Figure 3A that the tunable range is 100 nm. Meanwhile, as shown in Figure 3A, it is also easy to find that the wavelength-swept laser operates in a single-mode running state, and the SMSR is no less than 65.64 dB. The output power of the proposed external-cavity laser configuration at different resonance wavelengths is measured and the experimental results are depicted in Figure 3B. The output power of the external-cavity laser configuration changes as the wavelength-swept laser is tuned. The maximum output power is 16.26 dBm operating at 1582.07 nm, and the minimum output power is 14.12 dBm operating at 1620.35 nm. Obviously, the proposed external-cavity wavelength-swept laser system exhibits an output power of more than 14.12 dBm over the entire tunable range when the injected current is 410 mA. A plot of the lasing wavelength as a function of the micro-step applied to the physical length of the external cavity is shown in Figure 3C, where linear regression is performed to obtain the MHF performance of the external-cavity wavelength-swept laser. The physical length of the external cavity is increased from 46.89 mm to 50 mm with 2048 micro-step (1.328 μm)/step. In fact, when the wavelength is tuned, the external-cavity wavelength-swept laser will experience a mode-hopping if the change of resonating wavelength decided by the change of the physical length of the external cavity mismatch with the change of wavelength decided by the first-order reflections from the blazed diffraction grating. As shown in Figure 3C, it is believed that the change in the physical length of the external cavity does match the changing wavelength fed back into the single-angled facet gain chip, the mode-hopping is suppressed as the external-cavity wavelength-swept laser is continuously tuned over 100 nm. Similar experimental results are obtained and highlighted in Figure 3D. The maximum change of the adjacent wavelength subtracts (43.07 pm) is no more than two mode spacing, which indicates an MHF tunable output is able to be achieved [32].
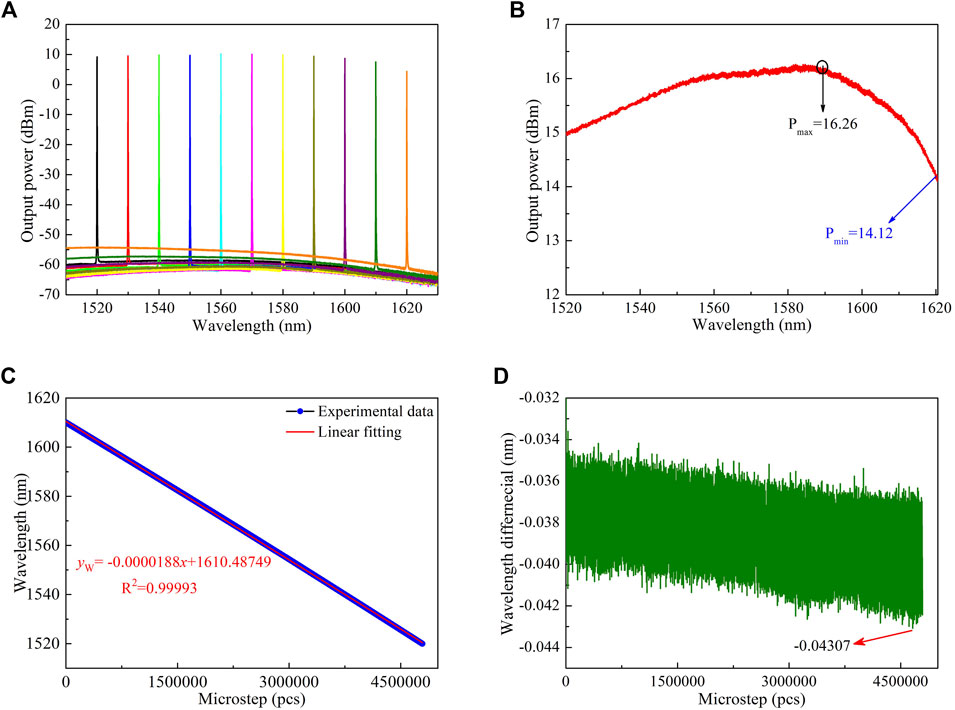
FIGURE 3. (A) The superimposed optical spectra at different lasing wavelengths. (B) Output power of the proposed external-cavity wavelength-swept laser. (C) The relationship between the lasing wavelength and the physical length of the wavelength-swept laser cavity. (D) The mode-hopping free performance of the proposed external-cavity wavelength-swept laser.
The SMSR of the proposed external-cavity wavelength-swept laser system at different lasing wavelengths is investigated and the corresponding results are shown in Figure 4A. With the blazed diffraction grating, the maximum output SMSR is around 71.26 dB operating at 1560 nm. Based on the classical grating equation, a large incident angle (80°) is beneficial to improve the blazed diffraction grating resolution. And the spontaneous emission noise can be suppressed with a large incident angle. Therefore, a higher SMSR can be obtained in the proposed external-cavity wavelength-swept laser configuration. Furthermore, a better SMSR is obtained at an intermediate tuning range, which is results from the match between the external-cavity lasing mode and the wavelength fed back to the internal chip as the first-order reflections from the blazed diffraction grating is rotated. However, in the longer lasing region, the edge of the material gain will occur, which will cause the growth in the spontaneous emission noise, meaning that the SMSR will decrease. Note that the mode-hopping-free tuning range is 1.69 times as large as the results in Ref. 33, while the SMSR in the full tuning range stay at a high level.
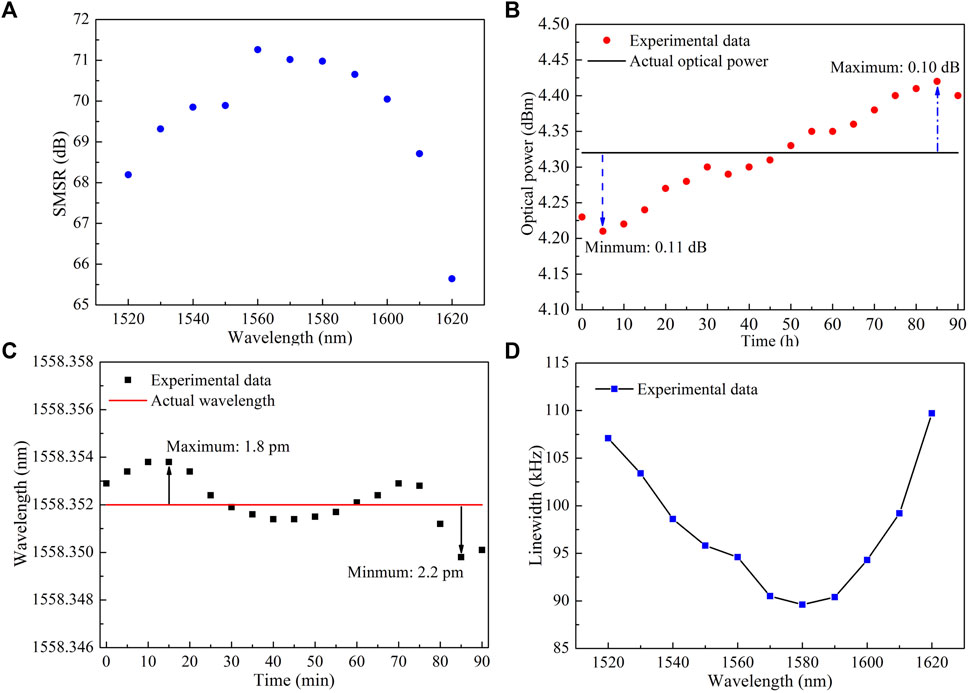
FIGURE 4. (A) Plot of the side-mode suppression ratio (SMSR) shown as the blue circles as a function of the lasing wavelength. (B) and (C) Output power and wavelength stability at wavelength 1558.352 nm with injection current of 410 mA, respectively. A variable optical attenuator is used to avoid the optical damage of the optical wavelength meter and the power meter. (D) Linewidth performance of the proposed system measured using the traditional DSH method with a total length of 24.5 km single-mode fiber and an 80 MHz fiber-coupled AOM. The injection current is 410 mA.
After warmup of 30 min, we monitored the stability of the wavelength and output power as shown Figures 4B, C, respectively, the duration is 90 min with 5 min step. As shown in the figure, the power deviation from the setting output power is less than ±0.11 dB and the wavelength deviation from the setting wavelength is less than 2.2 pm. The results show a good stability of the proposed external-cavity wavelength-swept laser system.
The laser linewidth performance is shown in Figure 4D, which is measured using the traditional delayed self-heterodyne (DSH) arrangement with a total length of 24.5 km single-mode fiber (Corning, SMF28e) and an 80 MHz fiber coupled acousto optic modulator (AOM). The linewidth of the laser output is less than 110 kHz within the full tuning range, the minimum linewidth is 90 kHz at the wavelength of 1580 nm. The linewidth at the central part of the full tuning range is narrower than the results in the two edges, which is most likely due to larger diffraction angles induced extra wavelength-dependent coupling loss at the edge of the full tuning range. The linewidth performance is similar to the results reported in Ref. [32].
4 Summary
In summary, a wide mode-hopping free external-cavity wavelength-swept laser with a high side-mode suppression ratio is designed, which is based on a traditional Littman-Metcalf configuration. Meanwhile, the tuning characteristics and side-mode suppression ratio of the proposed external-cavity diode laser are investigated experimentally. The external-cavity wavelength-swept laser can be continuously tuned over a range of 100 nm in the CL-band, with a maximum side-mode suppression ratio of 71.26 dB and an output optical power of more than 14.12 dBm. With the help of this designed external-cavity wavelength-swept laser, it has the potential applications for higher rate (e.g., 1 Tbit/s) coherent optical orthogonal frequency division multiplexing schemes. Future work shall focus on the optimization of the blazed grating diffraction efficiency to further suppress the spontaneous emission noise.
Data availability statement
The raw data supporting the conclusion of this article will be made available by the authors, without undue reservation.
Author contributions
Conceptualization, LS, SQ, and LH; methodology, LS, LH, AZ, and SQ; software, PL, JJ, TQ, and ZZ; validation, YZ, ZL, and BY; formal analysis, SQ and JH; investigation, YW and LS; resources, LH, ZL, AZ, and LS; data curation, SQ; writing—original draft preparation, LS and SQ; writing—review and editing, LH and LS; visualization, LS; supervision, LH; project administration, LS and LH; funding acquisition, LS, SQ, and LH. All authors have read and agreed to the published version of the manuscript.
Funding
This research was funded in part by National Key Research and Development Plan (2022YFF0705900); In part by National Natural Science Foundation of China (61605034); In part by Qingdao Postdoctoral Applied Research Project (20266153, 21290111); In part by Special Support for Postdoctoral Creative Funding in Shandong Province (202103076); And in part by Science and Technology on Electronic Test and Measurement Laboratory Foundation (ZF332).
Acknowledgments
Thanks to Hui Jin and Qiang Han for their support during the experiments.
Conflict of interest
Authors SQ, LS, LH, AZ, YW, ZL, JJ, ZZ, BY, PL, JH, YZ, and TQ were employed by Ceyear Technologies Co., Ltd.
Publisher’s note
All claims expressed in this article are solely those of the authors and do not necessarily represent those of their affiliated organizations, or those of the publisher, the editors and the reviewers. Any product that may be evaluated in this article, or claim that may be made by its manufacturer, is not guaranteed or endorsed by the publisher.
References
1. Robins N, Lance A, Close J, Sheng L, Liu J, Zhou S, et al. Piezo-locking a diode laser with saturated absorption spectroscopy. Appl Opt (2008) 47(28):5163–6. doi:10.1364/AO.47.005163
2. Doringsho K, Ernsting I, Rinkle R, Schiller S, Wicht A. Low-noise, tunable diode laser for ultra-high-resolution spectroscopy. Opt Lett (2007) 32(413):2876–8. doi:10.1364/OL.32.002876
3. Chi M, Jensen O, Petersen P. Tuning range and output power optimization of an external-cavity GaN diode laser at 455 nm. Appl Opt (2016) 55(9):2263–9. doi:10.1364/AO.55.002263
4. Ma Y, Yang Q, Tang Y, Chen S, Shieh W. 1-Tb/s single-channel coherent optical OFDM transmission over 600-km SSMF fiber with subwavelength bandwidth access. Opt Express (2009) 17(28):9421–7. doi:10.1364/OE.17.009421
5. Zhang L, Liu T, Chen L, Xu G, Jiang C, Liu J, et al. Development of an interference filter-stabilized external-cavity diode laser for space applications. Photonics (2020) 7(12):7010012. doi:10.3390/photonics7010012
6. Schioppo M, Brown R, McGrew W, Hinkley N, Fasano R, Beloy K, et al. Ultrastable optical clock with two cold-atom ensembles. Nat Photon (2017) 11(48):48–52. doi:10.1038/NPHOTON.2016.231
7. Yang X, Yin Y, Li X, Sheng L, Liu J, Zhou S, et al. External cavity diode laser as a stable-frequency light source for application in laser cooling of molecules. Chin Opt Lett (2016) 14(7):071403. doi:10.3788/COL201614.071403
8. Xu P, Yu X, Chen Z, Sheng L, Liu J, Zhou S, et al. Distributed refractive index sensing based on bending-induced multimodal interference and Rayleigh backscattering spectrum. Opt Express (2021) 29(14):21530–8. doi:10.1364/OE.430637
9. Zhao Z, Bai Z, Jin D, Qi Y, Ding J, Yan B, et al. Narrow laser-linewidth measurement using short delay self-heterodyne interferometry. Opt Express (2022) 30(17):30600–10. doi:10.1364/OE.455028
10. Sheng L, Huang L, Yan J, Qiao S, Zhang A, Jin H, et al. Distributed multi-parameter sensing based on the Brillouin scattering effect in orbital angular momentum guiding fiber. Opt Continuum (2022) 1(1):133–42. doi:10.1364/OPTCON.446952
11. Zhao Z, Bai Z, Jin D, Chen X, Qi Y, Ding J, et al. The influence of noise floor on the measurement of laser linewidth using short-delay-length self-heterodyne/homodyne techniques. Micromachines (2022) 13(8):1311. doi:10.3390/mi13081311
12. Qiao S, Sheng L, Song P, Zhang A, Guo H, Li P, et al. Measurement of distributed refractive index through Rayleigh backscattering spectra detection using a U-bent common fiber. In: Proc. SPIE 11907 Sixteenth National Conference on Laser Technology and Optoelectronics, 11907 (2021). p. 1190704. doi:10.1117/12.2600768
13. Mroziewicz B. External cavity wavelength tunable semiconductor lasers-a review. Opto-Electronics Rev (2008) 16:347–66. doi:10.2478/s11772-008-0045-9
14. Shank C, Bjorkholm J, Kogelnik H. Tunable distributed-feedback dye laser. Appl Phys Lett (1971) 18(9):395–6. doi:10.1063/1.1653714
15. Cai Y, Gao F, Chen H, Yang X, Bai Z, Qi Y, et al. Continuous-wave diamond laser with a tunable wavelength in orange–red wavelength band. Opt Commun (2023) 528:128985. doi:10.1016/j.optcom.2022.128985
16. Bai Z, Zhao Z, Tian M, Jin D, Pang Y, Li S, et al. A comprehensive review on the development and applications of narrow-linewidth lasers. Microwave Opt Tech Lett (2022) 64(12):2244–55. doi:10.1002/mop.33046
17. Iga K. Forty years of vertical-cavity surface-emitting laser: Invention and innovation. Jpn J Appl Phys (2018) 57(8S2):08PA01. doi:10.7567/JJAP.57.08PA01
18. Gmachl C, Straub A, Colombelli R, Capasso F, Sivco DL, Sergent A, et al. Single-mode, tunable distributed-feedback and multiple-wavelength quantum cascade lasers. IEEE J Quan Elect (2002) 38(6):569–81. doi:10.1109/JQE.2002.1005407
19. Niu Y, Yan X, Chen J, Ma Y, Zhou Y, Chen H, et al. Research progress on periodically poled lithium niobate for nonlinear frequency conversion. Infrared Phys Tech (2022) 125:104243. doi:10.1016/j.infrared.2022.104243
20. Jin D, Bai Z, Lu Z, Fan R, Zhao Z, Yang X, et al. 22.5-W narrow-linewidth diamond Brillouin laser at 1064 nm. Opt Lett (2022) 47(20):5360–3. doi:10.1364/OL.471447
21. Bai Z, Zhao C, Gao J, Chen Y, Li S, Li Y, et al. Optical parametric oscillator with adjustable pulse width based on KTiOAsO4. Opt Mater (2023) 136:113506. doi:10.1016/j.optmat.2023.113506
22. Komljenovic T, Srinivasan S, Norberg E, Davenport M, Fish G, Bowers J. Widely tunable narrow-linewidth monolithically integrated external-cavity semiconductor lasers. IEEE J Selected Top Quan Elect (2015) 21(6):214–22. doi:10.1109/JSTQE.2015.2422752
23. Li X, Shi J, Wei L, Ding K, Ma Y, Sun K, et al. Research progress of wide tunable Bragg grating external cavity semiconductor lasers. Materials (2022) 15(22):8256. doi:10.3390/ma15228256
24. Cui Q, Lei Y, Chen Y, Qiu C, Wang Y, Zhang D, et al. Advances in wide-tuning and narrow-linewidth external-cavity diode lasers. Sci China Inf Sci (2022) 65(8):181401. doi:10.1007/s11432-021-3454-7
25. Fedorova K, Cataluna M, Kudryashov I, Khalfin V, Rafailov E. Broadly tunable InGaAsP-InP strained multi-quantum-well external cavity diode laser. IEEE Photon Technol Lett (2010) 22(413):1205–7. doi:10.1109/LPT.2010.2051661
26. Bagley M, Wyatt R, Elton D, Wicks H, Spurdens P, Seltzer C, et al. 242 nm continuous tuning from a GRIN-SC-MQW-BH InGaAsP laser in an extended cavity. Electron Lett (1990) 26(4):267–9. doi:10.1049/el:19900178
27. Yuan H, Gao F, Yang T. Ultra-broadband tunable single- and double-mode InAs/InP quantum dot external-cavity laser emitting around 1.65μm. Opt Lett (2018) 43(413):3025–8. doi:10.1364/OL.43.003025
28. Gong H, Liu Z, Zhou Y, Zhang W. Extending the mode-hop-free tuning range of an external-cavity diode laser by synchronous tuning with mode matching. Appl Opt (2014) 53(33):7878–84. doi:10.1364/AO.53.007878
29. Zhang D, Zhao J, Yang Q, Liu W, Fu Y, Li C, et al. Compact MEMS external cavity tunable laser with ultra-narrow linewidth for coherent detection. Opt Express (2012) 20(18):19670–82. doi:10.1364/OE.20.019670
30. Kasai K, Nakazawa M, Tomomatsu Y, Endo T. 1.5 μm, mode-hop-free full C-band wavelength tunable laser diode with a linewidth of 8 kHz and a RIN of -130 dB/Hz and its extension to the L-band. Opt Express (2017) 25(18):22113–24. doi:10.1364/OE.25.022113
31. Wang Y, Wu H, Chen C, Zhou Y, Wang Y, Liang L, et al. An ultra-high-SMSR external-cavity diode laser with a wide tunable range around 1550 nm. Appl Sci (2019) 9:4390. doi:10.3390/app9204390
32. Zhang A, Qiao S, Sheng L, Huang L, Liu Z, Ju J, et al. Study on external cavity diode laser with a wide mode-hopping free tuning range. Front Phys (2022) 10:1093179. doi:10.3389/fphy.2022.1093179
Keywords: side-mode suppression ratio, mode-hopping free, wavelength-swept laser, Littman-Metcalf, narrow linewidth
Citation: Qiao S, Sheng L, Huang L, Zhang A, Wei Y, Liu Z, Ju J, Zhang Z, Yin B, Li P, Han J, Zhang Y and Qu T (2023) High-SMSR wavelength-swept laser with a CL-band mode-hopping free tuning range. Front. Phys. 11:1155266. doi: 10.3389/fphy.2023.1155266
Received: 31 January 2023; Accepted: 14 February 2023;
Published: 23 February 2023.
Edited by:
Bofeng Zhu, Nanyang Technological University, SingaporeCopyright © 2023 Qiao, Sheng, Huang, Zhang, Wei, Liu, Ju, Zhang, Yin, Li, Han, Zhang and Qu. This is an open-access article distributed under the terms of the Creative Commons Attribution License (CC BY). The use, distribution or reproduction in other forums is permitted, provided the original author(s) and the copyright owner(s) are credited and that the original publication in this journal is cited, in accordance with accepted academic practice. No use, distribution or reproduction is permitted which does not comply with these terms.
*Correspondence: Liwen Sheng, c2hlbmdsaXdlbjIwMDhAMTYzLmNvbQ==; Lin Huang, aHVhbmdsaW5AY2V5ZWFyLmNvbQ==