- 1Functional Ceramics and Smart Materials Lab, Department of Physics, Manipal University Jaipur, Jaipur, India
- 2Department of Physics, Cluster University of Jammu, Jammu, India
- 3Inter-University Accelerator Centre, New Delhi, India
In present communication, a new Aurivillius family compound K2Bi4Ti4WO18 was synthesized, and the impact of swift heavy ion (SHI), Ni+11 irradiation on its surface and dielectric properties has been studied in detail. The phase formation in this complex oxide, and crystallization to B2cb symmetry was confirmed by the X-ray diffraction. However, post irradiation the XRD, SEM and AFM studies shows the surface amorphization, in agreement with the theoretical calculations. Furthermore, the effect of irradiation was also observed in the bulk dielectric properties as the system transform to a phase with negative dielectric constant above 350 K in the radio frequencies. This transition is in correlation with significant change in other dielectric parameters such enhancement in AC conductivity, a helical Nyquist plot and multiple dielectric relaxations. This conspicuous changes in the dielectric response post irradiation is attributed to the SHI induced defect formation, modification of energy barriers and their consequences on the electronic structure. Thus, current study suggests that the dielectric properties of Aurivillius K2Bi4Ti4WO18 could be tailored by ion irradiation and opens a new possibility of tuning functional properties.
1 Introduction
The electric permittivity ϵ, and the magnetic permeability μ, are the fundamental quantities which determine the interaction of electromagnetic waves with matter[1-3]. These indices also dictate many physical and optical properties of materials. Depending on ϵ and μ, the field-matter interaction induces magnetization and polarization in magnetic materials and dielectric materials respectively. Both polarization and magnetization also produce their own fields, and the interaction of these fields with the external fields, result in a large spectrum of dielectric and magnetic phenomena. In the present work we’ll limit our discussion to dielectric systems, which is equally applicable to magnetic systems due to the correlation as derived by Maxwell’s equations [4, 5]. The dielectric constant for a linear, homogeneous and isotropic dielectric medium is given by ϵr = 1 + χ, where χ is electric susceptibility. This χ is further related to the polarization, P = (ϵ0)χE, where ϵ0 is permittivity of free space and E, is the electric field intensity; indicating a dependence of dielectric constant on polarization. These macroscopic properties (ϵr, P) in a non-polar dielectric are linked to the microscopic quantity (polarizability) by the famous Clausius-Mossotti relation
This idea, although allowed by fundamental electrodynamics principles, remain obscure for more than 30 years as it was not observed in any natural material. Based on the idea of a split ring resonator proposed by [7, 8] were able to experimentally demonstrate the idea of negative indexed systems. The composite system with a periodic array of interspaced conducting non-magnetic split ring resonators and continuous wires exhibits negative effective permittivity and permeability at microwave frequencies. In the recent past many such ‘composite’ systems were devised to show the negative indices. The systems with either of indices negative at a certain frequency, although rare, are also reported and do not violate the fundamental physical laws [9, 10]. Thus, materials with negative index (ϵ < 0, μ < 0) are of considerable scientific interest due to rich physical phenomena and large possibilities of novel photonic and electronic devices.
In present work a comparative study of Aurivillius K2Bi4Ti4WO18, before and after Ni+11 irradiation is reported in detail. The Aurivillius family compounds have attracted a flurry of research activities in the last few decades. These complex oxides possesses excellent dielectric and ferroelectric properties even at high temperatures and thus have many potential applications such as high-temperature actuators, sensors, energy harvesters, multi-layered ceramic capacitors, etc [11-17]. The Aurivillius family also known as bismuth layered ferroelectrics (BLFs) is represented by
2 Experimental section
For synthesis of polycrystalline K2Bi4Ti4WO18 (KBTW) solid-state reaction technique was employed. The stoichiometric amount of high purity (Sigma-Aldrich) K2C O 3, Bi2 O 3, Ti O 2, and W O 6 were taken as precursors. The powder mixture was mixed well using mortar and pestle and then ball milled for 6 h, followed by intermediate calcination at 950°C for 8 h. The calcined powder mixed with PVA binder was then pressed into a pellet (12 mm dia, and 1 mm thick), in a uni-axial press. The pellets were then sintered at 1,100 for 2 hours.These pellets were uniformly exposed to Ni+11 of 150 M eV energy at fluence of 5 × 1012 ions/cm2. The SHI irradiation was carried out at UD Pelletron Accelerator at Inter University Accelerator Centre (IUAC), New Delhi. The room temperature XRD measurement of pristine (unirradiated) and irradiated samples was performed using BRUKER D-8 X-ray diffractometer with Cu − Kα radiation of 1.54 Å. The SEM imaging was performed with a JEOL make JSM-7610F Plus FESEM. The AFM was performed using Veeco Instruments Inc. made a Multi-Mode SPM system with a Nanoscope IIIa controller. The temperature (100K–420K) dependent dielectric measurement was performed using an Agilent LCR meter in a frequency range of 10Hz-2 MHz. (Model No E4980A) with Lakeshore temperature controller (Model no-340). The initial calculation of expected radiation damage was performed using Stopping and Range of Ions in Matter (SRIM) software.
2.1 Stopping and range of ions in matter (SRIM) simulation
The depth profile of 150 MeV Ni+11 ions in KBTW crystal was theoretically simulated by SRIM software [41]. Figures 1A, B shows the longitudinal and cross-sectional view respectively post normal irradiation which suggests a nearly homogeneous irradiation effect. The energetic ions while penetrating predominantly loses its energy either via collision with nuclei (Sn) or with the atomic electrons (Se). The energy loss by these two processes in KBTW is summarized in Figure 1C. It is evident that the inelastic interaction with electron (Se) dominates initially upto a depth of 12μ m with a initial maximum value of 1.2 keV/Å. Thereafter, the nuclear interactions start increasing with a maximum at 0.025 keV/Å for penetration depth of 18 μ m. Thus, based on thermal spike model the top layer from surface up to 12μ m can be considered as electron loss layer and from 12μ m to 19μ m as nuclear loss layer. Thus the type of defects and imperfections created by ion track will vary from surface to penetration 17 μm with a straggle of 15 μm.
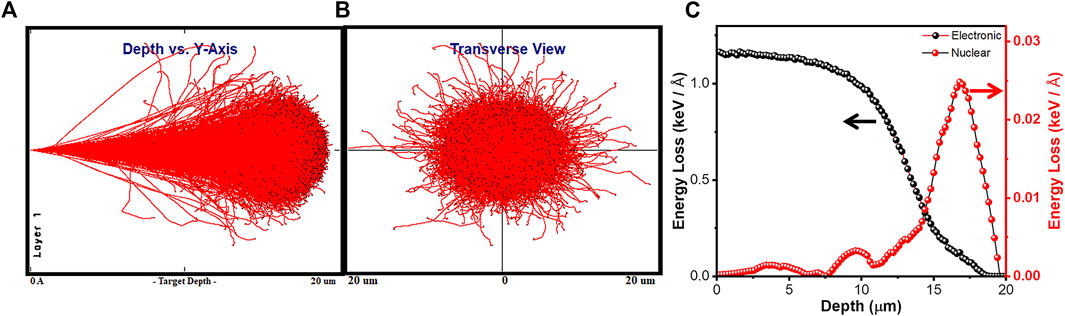
FIGURE 1. The longitudinal (A) and cross sectional (B) profile of KBTW ceramic pellet when irradiated by Ni+11 ions. (C) The nuclear and electron energy loss profile as a function of penetration depth from sample surface.
3 Results and discussion
3.1 Structural and surface studies
The Le-Bail analysis of the pristine sample is represented in Figure 2A. As evident from the Le-Bail fitting, all the observed peaks belongs to the orthorhombic B2cb space group-symmetry with a = 5.6085 (12), b = 5.38146), and c = 49.4763) Å, respectively. The structure parameters clearly indicate that crystal structure of KBTW is similar to a typical Aurivillius structures with 5-layered perovskite stacking sandwich between Bi2 O 2 layers. A detailed structural analysis is in communication and hence not discussed in this article. The penetration of ions (20 μm) is much larger than the interaction depth of x-rays in solids and thus explains the observed amorphous nature in irradiated ceramic (Figure 2B, bottom curve). This result is agreement with the SRIM calculations [42, 43].
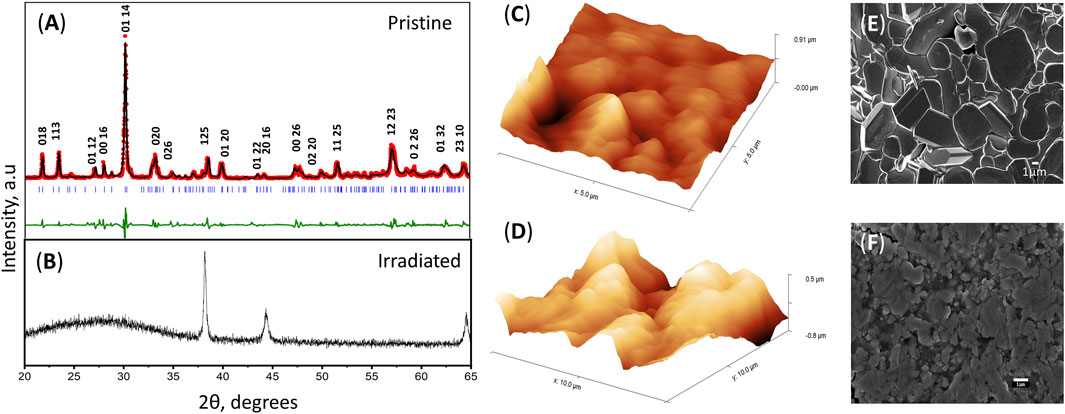
FIGURE 2. (A) The x-ray diffractogram of polycrystalline K2Bi4Ti4WO18. The bottom pattern (B) shows the amorphization post irradiation. The AFM images also depict an expected increase in the surface roughness after irradiation (D), when compared before irradiation (C) (E) The electron micrograph shows a grain distribution typical of bismuth layered structure. (F) The irradiation leads to surface amorphization with no clear crystallites.
The tapping mode AFM imaging was carried out to examine the surface effects and is shown in Figures 2C, D for pristine and irradiated sample respectively. The roughness profile for both the samples was measured with the aid of inbuilt processing software. The root mean square roughness of the sample shows nearly 10 times increase post treatment. The sample also shows formation of deep trenches, cracks and grain damage. A visible change is colour towards dark shade can be understood by localized heating caused while loosing of ion energy to the lattice. This result is consistent with other published works in functional ceramics [44-46].
To characterize the microstructural changes, the secondary electron imaging was performed. The unirradiated KBTW ceramic shows a plate like grain morphology, typical of bismuth layered structures Figure 2E. The grain distribution in sintered sample has an average crystallite size of 0.36 μ m. The changes induced by Ni+11 ions in its irradiated counterpart is clearly visible in electron micrograph, Figure 2F. The crystallites are fragmented into smaller grains and the agglomeration to form clusters is also observed. Due to diffused grain boundaries, it is challenging to measure the grain size after irradiation. This irradiation damage can be understood by the distribution of kinetic energy of the ions to the lattice. If the energy imparted is greater than the binding energy, the excessive energy can rise the local temperature resulting in localize melting, resulting in the observed amorphization of the surface [47, 48]. A more information on defect kinetics and grain dynamics during ion-beam exposure, can be gained by in situ measurements [49]. Thus, the combination of XRD, AFM and SEM provides more comprehensive information of surface morphology, and internal damage structure.
3.2 Broadband dielectric study
The temperature (100K–420 K) and frequency (10 Hz–2 MHz) dependence of the real part of the dielectric constant (ϵ′) for KBTW ceramics before and after irradiation is shown in Figure 3. The ϵ′ was observed to show a low frequency dispersion for KBTW with large values at low frequency (Figure 3B) and decrease monotonically with increase in frequency. The pristine KBTW follows a non-linear modified Debye equation. This equation indicate the presence of multiple relaxation mechanism due to surface boundaries effects, usually dominating at lower frequencies, and also termed Maxwell -Wagner (MW) polarization [5]; [50-52]. The ϵ′ was also observed to show a temperature dependence, and increases with temperature. However, no dielectric phase transition was observed for the pristine KBTW in the performed experimental range of temperature. This frequency and temperature dependence is in agreement with conventional dielectric systems [53-55]. As mentioned in the introduction the dielectric constant is related to the total polarizability by Clausius-Mossotti relation. Different polarization mechanisms such electronic, ionic, orientational, space charge and hopping creates dipoles and add to give total polarizability of the system [5]. The dielectric behavior for irradiated sample can be divided into two parts below and above 350 K. Below the transition temperature, the dielectric constant was observed to show an appreciable decrease in dielectric constant. A comparative change post irradiation at high frequency (2 MHz) is summarized in Table 1. This decrease in dielectric constant is attributed to the introduction of defects by irradiation which provides additional inertia to dipole relaxations.
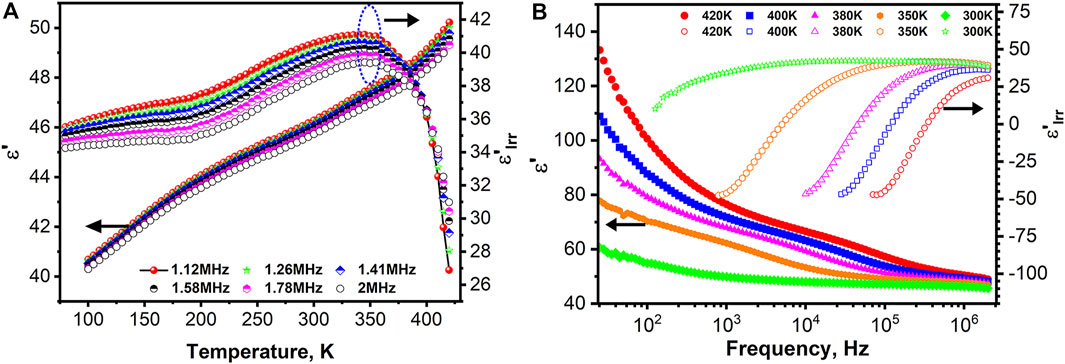
FIGURE 3. (A)The temperature dependent dielectric response for pristine (left y-axis) and irradiated (right y-axis) K2Bi4Ti4WO18. (B) The frequency dispersion of real part of dielectric constant at various temperature, shows a transformation to negative values above 350 K.
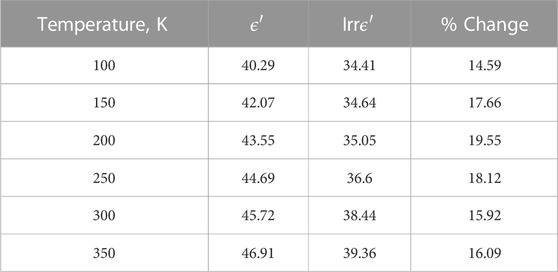
TABLE 1. The comparative dielectric values for pre- and post-irradiated samples at discrete temperatures and a fix frequency of 2 MHz.
Notably, the irradiated sample shows an anomalous change with increase in temperature near 350 K indicating a phase transition. This drop in dielectric constant (Irr ϵ′) was observed to show a frequency dependence as well. Interestingly, in irradiated KBTW the dielectric constant further drops to negative value above critical temperature (350 K) at radio frequencies. This anomalous transformation to negative dielectric constant state via SHI irradiation is not reported in literature. In presence of an external electric excitation all the conventional polarization mechanisms (electronic, ionic, orientational, hopping, space charge and spontaneous) aligns in the direction of field. All the induced as well as preexisting dipoles add up to give a positive value of effective dielectric constant. The negative value suggests that the net polarization opposes the external field. In present case the transition was observed above TC, indicating the thermally activated mechanism. This could be understood as either increase in the value of depolarizing.
Field (which opposes the applied field) or creation of new dipolar mechanism induced by SHI plausibly by trapping of charge carriers. A similar response was observed in polycrystalline PrMn O 3, wherein negative value is explained by modified Drude model which allows the formation of electron-electron pair [56].
Modulus Study: The frequency dependence of imaginary part of the modulus for temperatures above transition temperature shows frequency dependence (Figure 4A). The broad dispersive peak representing a dielectric relaxation is shown by solid symbols for pristine K2Bi4Ti4WO18. The peak maxima (ωmax) shift towards higher frequency with increase in temperature showing presence of a glassy phase. The irradiated ceramic also exhibit a similar dispersive peak with higher value of M″ and a small change in ωmax. Surprisingly, in addition to a broad dispersive peak several dispersive peaks were observed (Figure 4B). The irradiated ceramic also exhibit a similar dispersive peak with higher value of M″ and a small change inωmax. Surprisingly, in addition to a broad dispersive peak several dispersive peaks were observed. The temperature dependence of frequency maximum (ωmax) follows an Arrhenius relaxation given by
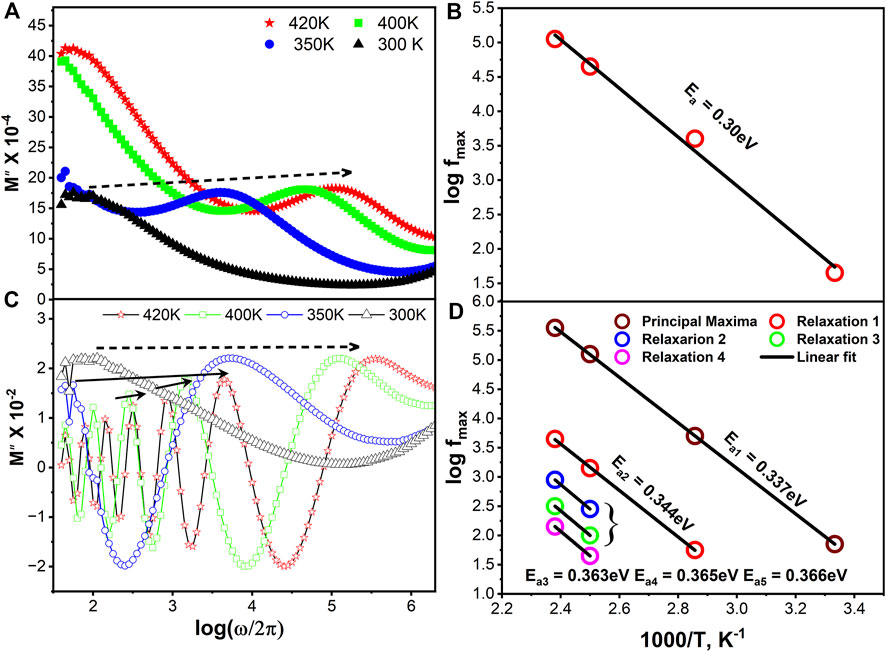
FIGURE 4. The frequency dispersion of imaginary part of modulus at discrete temperatures for pristine (A) (shown by solid symbols) and irradiated (C) (shown by hollow symbols) K2Bi4Ti4WO18. The activation energy profile for pristine (B) and irradiated (D) KBTW shows activation energies corresponding to different relaxations.
AC Conductivity Study: The frequency dependence of the real part of the ac conductivity, σ′ at different temperatures above transition temperature is shown in Figure 5A. The conductivity was observed to increase with temperature, indicating a thermally activated charge transport in these ceramic. However, a significant enhancement (7 times) in conductivity was also observed post irradiation. This SHI induced enhancement can be understood by introduction of charge carriers by metallic Ni+11 ions. These values are in agreement with the dielectric response. However, no anomalous change in the conductivity (which is related to ϵ″) above the transition temperature suggest the dielectric response is intrinsic in nature. The real part of the ac conductivity,σ′ was observed to follow the universal response proposed by Jonscher: σ(ω, T) = σdc + Aωn, where ω is angular frequency, T is temperature, σdc is dc conductivity, and A is a constant, and n is a temperature dependent exponent [59]. A deviation from this ‘universal’ response can be seen in the (inset of 5 A)) irradiated samples. The energy imparted by SHIs’ is extremely large and can cause disruption in terms of breaking of bonds, introduction of defects, strain and vacancies. This result in formation of new barrier potentials which when subjected to ac fields display overall increase in macroscopic conductivity and also manifest itself in terms of observed multiple dielectric relaxations in modulus study.
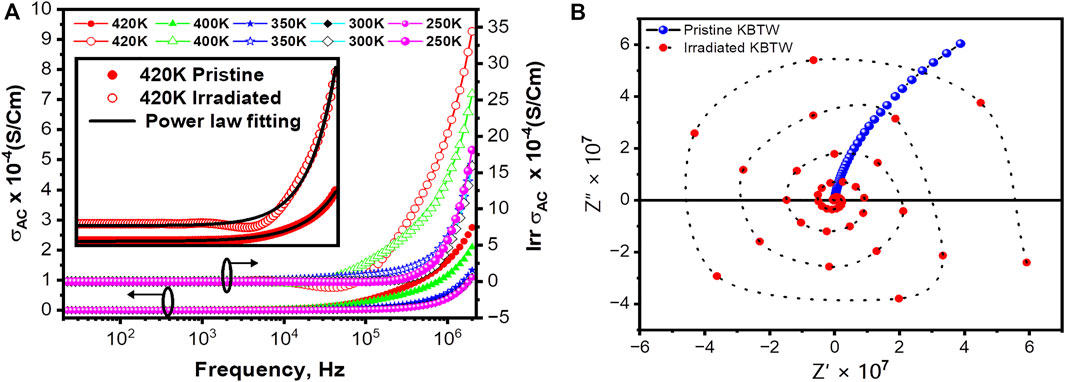
FIGURE 5. (A) The frequency dependence of conductivity for pristine and irradiated KBTW. The inset shows the deviation from Johncher’s law post irradiation. (B) The Comparative Nyquist plot in case of pristine and irradiated KBTW. The irradiated sample shows a spiral curve suggesting strong inductive effects at lower frequency.
Nyquist Plot analysis: The Nyquist representation in complex impedance plane for pristine and irradiated Aurivillius at 400 K is shown in Figure 5B. The pristine sample shows a portion of semi-circle which can further modeled to calculate the impedance contribution by grain and grain boundaries. In present case this circuit can be seen as combination of one parallel R-C circuit in series with R. This R and R-C represents the grain and grain boundary contributions respectively. However, the electro-chemical impedance for irradiated sample exhibits an unconventional spiral curve. The divergence from true semicircle is reported in many complex systems [60, 61]; but the observation of the helical Nyquist plot is a rare phenomenon. A similar curve is observed in impedance analysis of electrodes in Lithium-ion batteries and is termed as inductive loop [62]. This behavior is usually attributed to the solid - electrolyte interphase and a constant phase element is introduced to understand this behavior [63]. As seen in electron micro-graph, the irradiation has fragmented the grains and distorted the grain boundaries due to high energy transfer. Although not completely understood the combinations of various effects (both surface and bulk) could be responsible for the observed inductive behaviour.
3.3 Discussion
Materials with negative dielectric constant, although allowed by principle of electrodynamics, are not found in nature. Same is true for dia-electric materials, the only counterpart of magnetic phenomena which is missing in the electric order. Nevertheless, these systems have been pursued by the scientific community for long as they exhibit a variety of unusual properties which could revolutionize the existing electronic and photonic technologies. In search of such systems, the first breakthrough was achieved in an artificial structure consisting of a periodic array of copper (non-magnetic) resonators [7]. The interaction of such a composite system with oscillating fields is observed to be analogous to that of neutral plasma. And below the plasma frequency (microwave regime) the system exhibits negative indices. The negative relative permittivity was also observed in Perovskite PrMn O 3, urea-coated nano-particles, insulating polymers, Modified Rh800 dye, and array of gold nano-rods [56]; [64-66]. In present work the effective dielectric constant was observed to achieve negative values for the irradiated K2Bi4Ti4WO18 above 350 K. In addition the sample exhibits some unique features such as: the helical Nyquist plot, and multiple dipole relaxations. The seven fold rise in conductivity shows an increase in charge carriers due to impingement of Ni+11 in the insulating matrix of ceramic. This anomalous response observed in bulk properties is clearly due to interplay of many complex dipolar mechanisms. The SHI distorts the homogeneity of the ceramic and is capable of creating defects, charge carriers, dipoles, and shows a plasma like surface effect. As the temperature reaches above a critical value a transition to negative dielectric phase is observed plausibly due to activation of these dipoles.
4 Conclusion
In conclusion, the phase pure KBTW was prepared by solid state reaction technique. The structural study shows the formation of SHI induced amorphous structure by disintegration of crystallites and introduction of defects. The irradiated complex oxide shows an anomalous dielectric response such as: deviation of conductivity from Jonscher’s behaviour, a helical Nyquist plot and transition to negative dielectric constant state above 350 K. The ceramic is modified post irradiation due to absorption of kinetic energy of ion both via electronic and nuclear interactions. These interactions along with impingement of ions create defects, imperfections, and charge separation with modified energy barrier configuration. These dipoles when subjected to external field result in an unconventional macroscopic dielectric response. The phase transition to a phase with negative dielectric constant is attributed to the thermal-induced de-trapping of dipoles.
Data availability statement
The original contributions presented in the study are included in the article/supplementary material, further inquiries can be directed to the corresponding author.
Author contributions
RN and SR conceived the work while VS and AK carried out all the experimental work including synthesis of samples. IS helped in design or work and experimental support. RM and SK has provided dielectric and XRD characterization. VK, RN, and SR wrote the manuscript. All authors have discussed all results and the manuscript.
Funding
One author (SSR) acknowledges the financial support of Science and Engineering Research Board, India (ECR/2017/002691).
Acknowledgments
The authors acknowledge the Science and Engineering Research Board (SERB), Govt. of India for financial support under sanctioned project no. ECR/2017/002691. The authors also acknowledge the Sophisticated Analytical Instrument Facility (SAIF), Manipal University Jaipur for the FESEM analysis, the User Facility at IUAC, Delhi for pelletron accelerator facility, AFM, the X-ray diffraction measurements and dielectric measurements.
Conflict of interest
The authors declare that the research was conducted in the absence of any commercial or financial relationships that could be construed as a potential conflict of interest.
Publisher’s note
All claims expressed in this article are solely those of the authors and do not necessarily represent those of their affiliated organizations, or those of the publisher, the editors and the reviewers. Any product that may be evaluated in this article, or claim that may be made by its manufacturer, is not guaranteed or endorsed by the publisher.
References
1. Maxwell JC. Viii. a dynamical theory of the electromagnetic field. United Kingdom: Philosophical transactions of the Royal Society of London (1865).
2. Griffiths DJ. Introduction to electrodynamics. 4 edn. Cambridge: Cambridge University Press (2017). doi:10.1017/9781108333511
3. Rees WG. Interaction of electromagnetic radiation with matter. Cambridge, United Kingdom: Cambridge University Press (2001). doi:10.1017/CBO9780511812903.004
4. Kao KC. 1 - introduction. In: KC Kao, editor. Dielectric phenomena in solids. San Diego: Academic Press (2004). p. 1–39. doi:10.1016/B978-012396561-5/50011-6
5. Kao KC. 2 - electric polarization and relaxation. In: KC Kao, editor. Dielectric phenomena in solids. San Diego: Academic Press (2004). p. 41–114. doi:10.1016/B978-012396561-5/50012-8
6. Viktor GV. The electrodynamics of substances with simultaneously negative values of ϵ and m. Soviet Phys Uspekhi (1968) 10:509–14.
7. Porto J, Garcia-Vidal F, Pendry J. Transmission resonances on metallic gratings with very narrow slits. Phys Rev Lett (1999) 83:2845–8. doi:10.1103/physrevlett.83.2845
8. Smith DR, Padilla WJ, Vier D, Nemat-Nasser SC, Schultz S. Composite medium with simultaneously negative permeability and permittivity. Phys Rev Lett (2000) 84:4184–7. doi:10.1103/physrevlett.84.4184
9. Shelby RA, Smith DR, Schultz S. Experimental verification of a negative index of refraction. Science (2001) 292:77–9. doi:10.1126/science.1058847
10. Smith DR, Padilla WJ, Vier DC, Nemat-Nasser SC, Schultz S. Composite medium with simultaneously negative permeability and permittivity. Phys Rev Lett (2000) 84:4184–7. doi:10.1103/PhysRevLett.84.4184
11. Wei H, Wang H, Xia Y, Cui D, Shi Y, Dong M, et al. An overview of lead-free piezoelectric materials and devices. J Mater Chem C (2018) 6:12446–67. doi:10.1039/C8TC04515A
12. Hussain F, Khesro A, Lu Z, Wang G, Wang D. Lead free multilayer piezoelectric actuators by economically new approach. Front Mater (2020) 7. doi:10.3389/fmats.2020.00087
13. Maurya D, Peddigari M, Kang M-G, Geng LD, Sharpes N, Annapureddy V, et al. Lead-free piezoelectric materials and composites for high power density energy harvesting. J Mater Res (2018) 33:2235–63. doi:10.1557/jmr.2018.172
14. Do N-B, Lee H-B, Yoon C-H, Kang J-K, Lee J-S, Kim I-W. Effect of ta-substitution on the ferroelectric and piezoelectric properties of bi 0.5/(na 0.82 k 0.18) 0.5 tio 3 ceramics. Trans Electr Electron Mater (2011) 12:64–7. doi:10.4313/teem.2011.12.2.64
15. Wu J. Perovskite lead-free piezoelectric ceramics. J Appl Phys (2020) 127:190901. doi:10.1063/5.0006261
16. Takenaka T, Komura KKK, Sakata KSK. Possibility of new mixed bismuth layer-structured ferroelectrics. Jpn J Appl Phys (1996) 35:5080. doi:10.1143/JJAP.35.5080
17. Sun H, Wu Y, Xie X, Lu Y, Yao T, Zhong J, et al. Ferroelectric, magnetic, and optical properties of aurivillius compound bi5feti2.5co0.5o15. J Materiomics (2018) 4:353–9. doi:10.1016/j.jmat.2018.09.005
18. Sharma VK, Nathawat R, Rathore SS. Dielectric properties correlation with microstructure in abi4ti4o15 (a = sr, ba) bismuth layered ferroelectrics. Mater Adv (2022) 3:4890–8. doi:10.1039/D2MA00333C
19. Sharma VK, Nathawat R, Chakinala N, Chakinala AGG, Rathore SS. Enhanced photocatalytic properties in metal modified bismuth layered ferroelectric ceramics, srbi4ti4o15. SSRN Electron J (2022) 2022. doi:10.2139/ssrn.4233197
20. Bell AJ, Deubzer O. Lead-free piezoelectrics—The environmental and regulatory issues. MRS Bull (2018) 43:581–7. doi:10.1557/mrs.2018.154
21. Koruza J, Bell AJ, Frömling T, Webber KG, Wang K, Rödel J. Requirements for the transfer of lead-free piezoceramics into application. J Materiomics (2018) 4:13–26. doi:10.1016/j.jmat.2018.02.001
22. Zhang J, Xu D, Tong L, Qi H, Zhang D, Wang C. Surface layer and its effect on dielectric properties of sic ceramics. J Alloys Comp (2018) 734:16–21. doi:10.1016/j.jallcom.2017.10.218
23. Beier CW, Cuevas MA, Brutchey RL. Effect of surface modification on the dielectric properties of batio3 nanocrystals. Langmuir (2010) 26:5067–71. doi:10.1021/la9035419
24. Hirvonen JK, Clayton CR. Materials modification by ion implantation. Boston, MA: Springer US (1983). p. 323–83. doi:10.1007/978-1-4613-3733-1_12
25. McHargue CJ. The mechanical properties of ion implanted ceramics-a review. Defect and Diffusion Forum (1988) 57:359–80.
26. Picraux ST. Ion implantation in metals. Annu Rev Mater Sci (1984) 14:335–72. doi:10.1146/annurev.ms.14.080184.002003
27. Page T, Bull S. Ion implantation in ceramics. In: Reference module in materials science and materials engineering. Amsterdam, Netherlands: Elsevier (2016). doi:10.1016/B978-0-12-803581-8.03725-5
28. Clark J, Pollock J. Ion implantation and fracture toughness of ceramics. Australia: Fourth Australian conference on nuclear techniques of analysis: Proceedings (1985).
29. Popok V. Ion implantation of polymers: Formation of nanoparticulate materials. Rev Adv Mater Sci (2012) 30:1–26.
30. Loh I, Oliver R, Sioshansi P. Conducting polymers by ion implantation. Nucl Instr Methods Phys Res Section B: Beam Interactions Mater Atoms (1988) 34:337–46. doi:10.1016/0168-583x(88)90054-7
31. Rautray TR, Narayanan R, Kwon T-Y, Kim K-H. Surface modification of titanium and titanium alloys by ion implantation. J Biomed Mater Res B: Appl Biomater (2010) 93:581–91. doi:10.1002/jbm.b.31596
32. Müller-Steinhagen H, Zhao Q. Investigation of low fouling surface alloys made by ion implantation technology. Chem Eng Sci (1997) 52:3321–32. doi:10.1016/s0009-2509(97)00162-0
33. Höchbauer T, Misra A, Hattar K, Hoagland R. Influence of interfaces on the storage of ion-implanted he in multilayered metallic composites. J Appl Phys (2005) 98:123516. doi:10.1063/1.2149168
34. Magruder R, Yang L, Haglund R, White C, Yang L, Dorsinville R, et al. Optical properties of gold nanocluster composites formed by deep ion implantation in silica. Appl Phys Lett (1993) 62:1730–2. doi:10.1063/1.109588
35. Madauß L, Ochedowski O, Lebius H, Ban-d’Etat B, Naylor CH, Johnson AC, et al. Defect engineering of single-and few-layer mos2 by swift heavy ion irradiation. 2D Mater (2016) 4:015034. doi:10.1088/2053-1583/4/1/015034
36. Zhu Y, Cai Z, Budhani R, Suenaga M, Welch D. Structures and effects of radiation damage in cuprate superconductors irradiated with several-hundred-mev heavy ions. Phys Rev B (1993) 48:6436–50. doi:10.1103/physrevb.48.6436
37. Douillard L, Duraud J. Swift heavy ion amorphization of quartz—A comparative study of the particle amorphization mechanism of quartz. Nucl Instr Methods Phys Res Section B: Beam Interactions Mater Atoms (1996) 107:212–7. doi:10.1016/0168-583x(95)01044-0
38. Costantini J-M, Beuneu F. Point defects induced in yttria-stabilized zirconia by electron and swift heavy ion irradiations. J Phys Condensed Matter (2011) 23:115902. doi:10.1088/0953-8984/23/11/115902
39. Dufour C, Audouard A, Beuneu F, Dural J, Girard J, Hairie A, et al. A high-resistivity phase induced by swift heavy-ion irradiation of bi: A probe for thermal spike damage? J Phys Condensed Matter (1993) 5:4573–84. doi:10.1088/0953-8984/5/26/027
40. Singh Solanki R, Patel JP, Rath C, Kulriya PK, Avasthi DK, Pandey D. Swift heavy ion irradiation induced structural phase transitions in batio3: An in-situ x-ray diffraction study (2017). arXiv e-printsarXiv–1703.
41. Ziegler JF, Ziegler M, Biersack J. Srim – The stopping and range of ions in matter (2010). Nucl Instr Methods Phys Res Section B: Beam Interactions Mater Atoms (2010) 268:1818–23. doi:10.1016/j.nimb.2010.02.091
42. Park S, Lang M, Tracy CL, Zhang J, Zhang F, Trautmann C, et al. Swift heavy ion irradiation-induced amorphization of la2ti2o7. Nucl Instr Methods Phys Res Section B: Beam Interactions Mater Atoms (2014) 326:145–9. doi:10.1016/j.nimb.2013.10.088
43. Lang M, Zhang F, Li W, Severin D, Bender M, Klaumünzer S, et al. Swift heavy ion-induced amorphization of cazro3 perovskite. Nucl Instr Methods Phys Res Section B: Beam Interactions Mater Atoms (2012) 286:271–6. doi:10.1016/j.nimb.2011.12.028
44. Pandian M, Krishnaprasanth A, Palanisamy M, Bangaru G, Meena R, Dong C-L, et al. Effects of heavy ion irradiation on the thermoelectric properties of in2 (te1-xsex) 3 thin films. Nanomaterials (2022) 12:3782. doi:10.3390/nano12213782
45. Sinduja M, Amirthapandian S, Magudapathy P, Masarat A, Krishnan R, Srivastava S, et al. Role of ion irradiation induced defects in thermoelectric transport properties of bi2te3 thin films. Thin Solid Films (2021) 734:138830. doi:10.1016/j.tsf.2021.138830
46. Gadani K, Rathod K, Shrimali V, Rajyaguru B, Udeshi B, Vadgama V, et al. Effect of 200 mev ag+ 15 ion irradiation on structural, microstructural and dielectric properties of y0- 95sr0-05mno3 manganite films. Solid State Commun (2020) 318:113975. doi:10.1016/j.ssc.2020.113975
47. Kalita P, Ghosh S, Gutierrez G, Rajput P, Grover V, Sattonnay G, et al. Grain size effect on the radiation damage tolerance of cubic zirconia against simultaneous low and high energy heavy ions: Nano triumphs bulk. Scientific Rep (2021) 11:10886–10. doi:10.1038/s41598-021-90214-6
48. Sharma M, Gaur A, Quamara JK. Effect of 80 mev o6+ ion irradiation on structural, morphological, dielectric, and ferroelectric properties of (1-x) pvdf/(x) batio3 nanocomposites. Ionics (2020) 26:471–81. doi:10.1007/s11581-019-03163-6
49. Niu T, Rayaprolu S, Shang Z, Sun T, Fan C, Zhang Y, et al. In situ study on heavy ion irradiation induced microstructure evolution in single crystal cu with nanovoids at elevated temperature. Mater Today Commun (2022) 33:104418. doi:10.1016/j.mtcomm.2022.104418
50. Salame PH, Prakash O, Kulkarni AR. Colossal dielectric constant and extremely low loss in t-type la2cuo4- δ ceramics. Ceramics Int (2016) 42:13207–14. doi:10.1016/j.ceramint.2016.05.113
51. Rathore SS, Nathawat R, Vitta S. A ‘mixed’dielectric response in langasite ba 3 nbfe 3 si 2 o 14. Phys Chem Chem Phys (2021) 23:554–62. doi:10.1039/d0cp04965d
52. Sharma VK, Nathawat R, Rathore SS. Structural, thermal and dielectric studies of mixed spinel cofe2o4. Mater Today Proc (2021) 46:2193–6. doi:10.1016/j.matpr.2021.03.153
53. Rayssi C, Kossi SE, Dhahri J, Khirouni K. Frequency and temperature-dependence of dielectric permittivity and electric modulus studies of the solid solution Ca0.85Er0.1Ti1−xCo4x/3O3 (0 ≤ x ≤ 0.1). Rsc Adv (2018) 8:17139–50. doi:10.1039/c8ra00794b
54. Narang SB, Kaur D, Pubby K. Frequency and temperature dependence of dielectric and electric properties of Ba2-xSm4+2x/3Ti8O24 with structural analysis. Mater Science-Poland (2015) 33:268–77. doi:10.1515/msp-2015-0034
55. Santhosh Kumar T, Pamu D. Temperature dependent dielectric properties of ba (zn 1/3 ta 2/3) o 3 ceramics. XVI Natl Semin Ferroelectrics Dielectrics (Nsfd-xvi) (2011) 1372:20–3.
56. Balu SK, Shanker NP, Manikandan M, Aparnadevi N, Mukilraj T, Manimuthu P, et al. Crossover to negative dielectric constant in perovskite prmno3. physica status solidi (a) (2020) 217:2000230. doi:10.1002/pssa.202000230
57. Rathore SS, Vitta S. Effect of divalent ba cation substitution with sr on coupled ‘multiglass’ state in the magnetoelectric multiferroic compound ba3nbfe3si2o14. Scientific Rep (2015) 5:9751–11. doi:10.1038/srep09751
58. Park Y, Knowles KM. Glass-like behaviour in the relaxor ferroelectric 0.6 pb (-0.4 pb. J Phys D: Appl Phys (1999) 32:504–12. doi:10.1088/0022-3727/32/4/020
60. Magar HS, Hassan RY, Mulchandani A. Electrochemical impedance spectroscopy (eis): Principles, construction, and biosensing applications. Sensors (2021) 21:6578. doi:10.3390/s21196578
61. Allison A, Andreas H. Minimizing the nyquist-plot semi-circle of pseudocapacitive manganese oxides through modification of the oxide-substrate interface resistance. J Power Sourc (2019) 426:93–6. doi:10.1016/j.jpowsour.2019.04.029
62. Itagaki M, Taya A, Watanabe K, Noda K. Deviations of capacitive and inductive loops in the electrochemical impedance of a dissolving iron electrode. Anal Sci (2002) 18:641–4. doi:10.2116/analsci.18.641
63. Jorcin J-B, Orazem ME, Pébère N, Tribollet B. Cpe analysis by local electrochemical impedance spectroscopy. Electrochimica Acta (2006) 51:1473–9. doi:10.1016/j.electacta.2005.02.128
64. Yan H, Zhao C, Wang K, Deng L, Ma M, Xu G. Negative dielectric constant manifested by static electricity. Appl Phys Lett (2013) 102:062904. doi:10.1063/1.4792064
65. Shalaev VM, Cai W, Chettiar UK, Yuan H-K, Sarychev AK, Drachev VP, et al. Negative index of refraction in optical metamaterials. Opt Lett (2005) 30:3356–8. doi:10.1364/OL.30.003356
Keywords: swift heavy ion irradiation, impedance spectroscopy, phase transition, aurivillius ceramic oxides, negative index
Citation: Sharma VK, Kumawat AK, Rathore SS, Sulania I, Meena RC, Kedia SK and Nathawat DR (2023) The SHI irradiation induced transition to negative dielectric constant phase in K2Bi4Ti4WO18. Front. Phys. 11:1127118. doi: 10.3389/fphy.2023.1127118
Received: 19 December 2022; Accepted: 23 January 2023;
Published: 24 February 2023.
Edited by:
Surender Kumar Sharma, Federal University of Maranhão, BrazilReviewed by:
Jose Martin Yañez Limon, National Polytechnic Institute, MexicoLipeng Xin, Xi’an Jiaotong University, China
Copyright © 2023 Sharma, Kumawat , Rathore , Sulania , Meena , Kedia and Nathawat. This is an open-access article distributed under the terms of the Creative Commons Attribution License (CC BY). The use, distribution or reproduction in other forums is permitted, provided the original author(s) and the copyright owner(s) are credited and that the original publication in this journal is cited, in accordance with accepted academic practice. No use, distribution or reproduction is permitted which does not comply with these terms.
*Correspondence: Dr. Rashi Nathawat, cmFzaGkubmF0aGF3YXRAZ21haWwuY29t