- 1Department of Applied Physics, Nanjing University of Science and Technology, Nanjing, China
- 2Key Laboratory of Computational Physics, Institute of Applied Physics and Computational Mathematics, Beijing, China
- 3HEDPS, Center for Applied Physics and Technology, Peking University, Beijing, China
- 4Laboratoire de Chimie Physique–Mati`ere et Rayonnement, CNRS, Sorbonne Universités, Paris, France
In our last study [J. Phys. B At. Mol. Opt. Phys. 54, 125,102 (2021).], we reported the ab initio calculation of the full-dimensional potential energy surfaces of water molecule including 9 A’ and 9 A” states in Cs symmetry. In this study, we performed additional non-adiabatic semi-classical studies based on the potential energy surfaces. Our simulation successfully repeated the near picosecond lifetime of the
1 Introduction
With the development of vacuum ultra-violet free electron laser (VUV-FEL) light sources combined with time-sliced velocity-map imaging (TSVMI) [1] and time-resolved photo-electron spectroscopy (TRPES) technique [2], dynamical studies for the photo-chemistry process in molecules from highly excited electronic states have been performing for several years before [1, 3, 4]. Among small molecules, water had been extensively studied as an ideal polyatomic system. Interesting phenomena have been found for the photodissociation dynamics of highly-excited states of water, including the hot rotation of OH fragments in ground and excited states at special incident photon wavelengths [4, 5] the long-lived lifetime of
Despite the extensive experimental studies of the photodissociation of water molecule in highly-excited states, corresponding theoretical studies are relatively scarce due to the lack of the corresponding potential energy surfaces (PES). On the other hand, most theoretical studies focus on specific fragment quantum distributions such as the rovibronic [8] or the fine structures of OH radical [9], and few studies consist of all three channels of H + OH, H2+O and H + H + O. In our latest work [10], we obtained the full dimensional potential energy surfaces with the combined multi configurational self-consistent field and multi reference single and double excitation configuration interaction method (MCSCF + MRDCI), nearly 99,000 geometries are considered which include all the three mentioned channels.
If we do not aim at studying quantum effects such as interference, energy resonant, or geometric phase, a semi-classical simulation of the nuclear motions is good enough to describe the reaction behavior with a lower computational cost. In this paper, based on our full-dimensional PESs, the photodissociation of water molecule with respect to the photon energy of 9–12 eV are studied with a semi-classical method, and the non-adiabatic transition is included using a Landau-Zener type approach near conical-intersections or avoid-crossings. It should be noted that, in the present study, only the states of
2 Theoretical method
The semi-classical simulation is performed by solving the Newton equations with the Verlet algorithm:
Here,
During the simulation, such probability is compared with a pseudorandom number ξ within a uniform interval of [0,1]. If
to ensure the energy conservation. The summation is over all nuclear with mass
The initial geometry samplings are based on a Wigner distribution [16, 17] near the equilibrium geometry of the ground state
3 Results and discussion
3.1 The lifetime of state and
We obtain the lifetime of state
The state
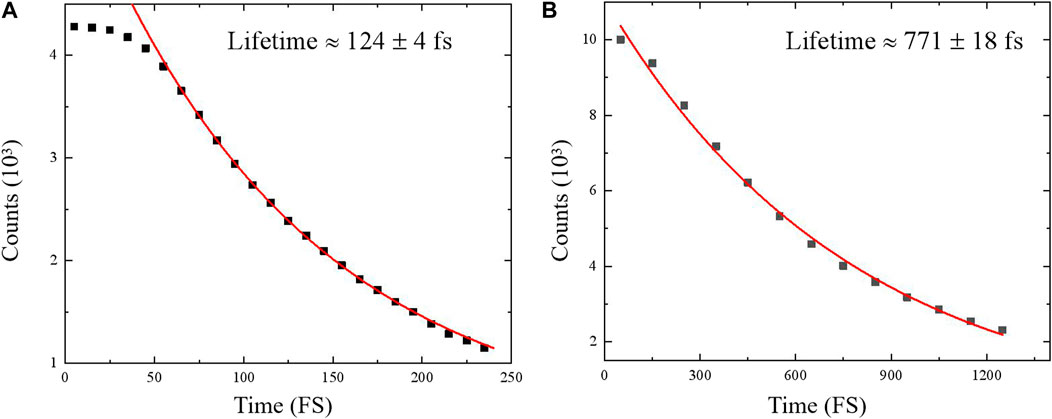
FIGURE 1. Number of undissociated trajectories versus simulation time of state
The lifetime of the time-resolved photo-electron spectroscopy (TRPES). They suggested a weak non-adiabatic traisition from
3.2 The channel ratio with respect to the incident photon energy
In previous theoretical studies, researchers mainly focus on part of the dissociation channels. e.g., for H + OH dissociation channel, Jiang etal [20] studied the rotational and vibrational distributions of the OH fragment, Zhou etal [8] studied the effect of spin-orbit couplings on the rotational distributions of OH fragment. For H2+O channel, the only theoretical study was performed by van Harrevelt etal [21], in which the rotational and vibrational distributions of H2 were obtained, a 10% ratio for the H2+O channel was found which was in good accordance with earlier experimental results t [22]. For the three body channels, no specific theoretical studies are published. In a recent study, Chang etal [7] found a quite large ratio of the three-body channel at short wavelengths (near 100 nm, 12.34 eV).
Here we perform a simulation containing all three channels with the full-dimensional PESs obtained in our last work [10]. A key point is the determination of the initial surface. In the present study, we perform the simulation from all the excited
Here
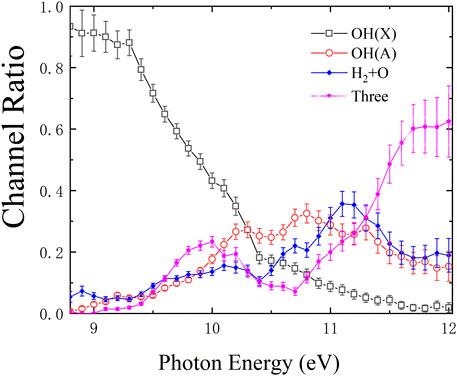
FIGURE 2. Branching ratio versus photon energy. OH(X) and OH(A) correspond to the H + OH channel with the OH fragment on ground and first excited states, respectively. “Three” correspond to the H + H + O channel. The error bars are determined from the statistical error of the number of the trajectories of each channel.
As shown in Figure 2, at low photon energy (near 9 eV), most trajectories lead to the H + OH(X) channel. As the photon energy increases the ratio of H + OH(X) channel reduces rapidly and the ratios of other channels rise. H + OH(A) channel corresponds to the dissociation on
3.3 The H2+O channel
In recent experimental work of Chang etal [3], the photo dissociation of water at wavelength ranging from 102.67 to 112.81 nm (10.99—12.08 eV), corresponding to the H2+O channel, was studied. The H2+O (1S) channel was observed and the vibrationally excited H2 molecule was mostly populated. This is surprising because the H2+O (1S) channels correspond to the fourth
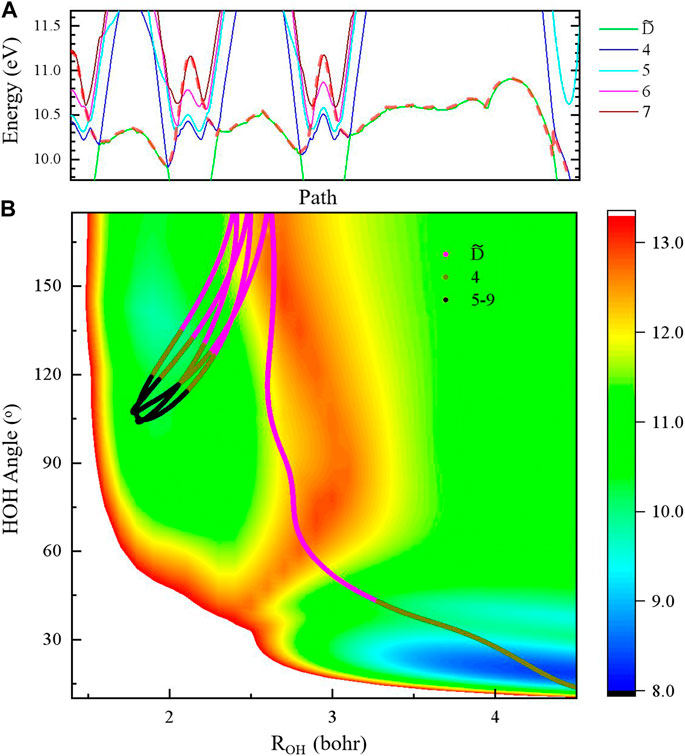
FIGURE 3. Reaction path corresponding to H2+O (1S) channel. In panel (A), the adiabatic potential energies within the
4 Conclusion
By performing semi-classical simulation with Landau-Zener surface hopping approximation, the photo-dissociation dynamics of water molecule in high-lying states are studied. The lifetimes of
Data availability statement
The original contributions presented in the study are included in the article/Supplementary Material, further inquiries can be directed to the corresponding author.
Author contributions
Conceptualization: YW, RL methodology: YP, NS investigation: YP, XH, visualization: YP, NS, XH supervision: YW, JW, RL writing—original draft: YP, NS writing—review and editing: YW, JW, RL.
Acknowledgments
Grants from the NSFC(Nos. 11934004,11904028) are acknowledged. We thank Dr K. Luo, Dr C. Yu., Dr J. W. Gao and Dr C. Z. Gao for helpful discussions. NS thanks the Institut de Chimie du CNRS for financial support.
Conflict of interest
The authors declare that the research was conducted in the absence of any commercial or financial relationships that could be construed as a potential conflict of interest.
Publisher’s note
All claims expressed in this article are solely those of the authors and do not necessarily represent those of their affiliated organizations, or those of the publisher, the editors and the reviewers. Any product that may be evaluated in this article, or claim that may be made by its manufacturer, is not guaranteed or endorsed by the publisher.
References
1. Chang Y, Yu S, Li Q, Yu Y, Wang H, Su S, et al. Tunable VUV photochemistry using vacuum ultraviolet free electron laser combined with H-atom Rydberg tagging time-of-flight spectroscopy. Rev Sci Instrum (2018) 89, 063113. doi:10.1063/1.5017757
2. He Z, Yang D, Chen Z, Yuan K, Dai D, Wu G, et al. An accidental resonance mediated predissociation pathway of water molecules excited to the electronic C̃ state. Phys Chem Chem Phys (2017) 19:29795–800. doi:10.1039/c7cp06286a
3. Chang Y, An F, Chen Z, Luo Z, Zhao Y, Hu X, et al. Vibrationally excited molecular hydrogen production from the water photochemistry. Nat Commun (2021) 12:6303. doi:10.1038/s41467-021-26599-9
4. Chang Y, Yu Y, Wang H, Hu X, Li Q, Yang J, et al. Hydroxyl super rotors from vacuum ultraviolet photodissociation of water. Nat Commun (2019) 10:1250. doi:10.1038/s41467-019-09176-z
5. Chang Y, An F, Li Q, Luo Z, Che L, Yang J, et al. Electronically excited OH super-rotors from water photodissociation by using vacuum ultraviolet free-electron laser pulses. J Phys Chem Lett (2020) 11:7617–23. doi:10.1021/acs.jpclett.0c02320
6. Yang D, Min Y, Chen Z, He Z, Chen Z, Yuan K, et al. Ultrafast dynamics of water molecules excited to electronic F̃ states: A time-resolved photoelectron spectroscopy study. Chin J. Chem. Phys. (2019) 32:53–8. doi:10.1063/1674-0068/cjcp1811243
7. Chang Y, Yu Y, An F, Luo Z, Quan D, Zhang X, et al. Three body photodissociation of the water molecule and its implications for prebiotic oxygen production. Nat Commun (2021) 12:2476. doi:10.1038/s41467-021-22824-7
8. Jiang B, Xie D, Guo H. State-to-state photodissociation dynamics of triatomic molecules: H2O in the B band. J Chem Phys (2012) 136:034302. doi:10.1063/1.3676725
9. Zhou L, Xie D, Sun Z, Guo H. Product fine-structure resolved photodissociation dynamics: The A band of H2O. J Chem Phys (2014) 140, 024310. doi:10.1063/1.4861230
10. Peng Y, Hu X, Wu Y, Wang J, Lu R, Sisourat N. Photodissociation dynamics of water molecule at short photon wavelengths: Full dimensional potential energy surface of rydberg states. J Phys B: Mol Opt Phys (2021) 54:125102. doi:10.1088/1361-6455/ac01ab
11. Belyaev AK, Lebedev OV. Nonadiabatic nuclear dynamics of atomic collisions based on branching classical trajectories. Phys Rev A (2011) 84, 014701. doi:10.1103/physreva.84.014701
12. Belyaev AK, Lasser C, Trigila G. Landau–Zener type surface hopping algorithms. J Chem Phys (2014) 140:224108. doi:10.1063/1.4882073
13. Kube S, Lasser C, Weber M. Monte Carlo sampling of Wigner functions and surface hopping quantum dynamics. J Comput Phys (2009) 228:1947–62. doi:10.1016/j.jcp.2008.11.016
14. Lasser C, Swart T. Single switch surface hopping for a model of pyrazine. J Chem Phys (2008) 129, 034302. doi:10.1063/1.2954019
15. Fermanian Kammerer C, Lasser C. Single switch surface hopping for molecular dynamics with transitions. J Chem Phys (2008) 128:144102. doi:10.1063/1.2888549
17. Dahl JP, Springborg M. The Morse oscillator in position space, momentum space, and phase space. J Chem Phys (1988) 88:4535–47. doi:10.1063/1.453761
18. Steinkellner O, Noack F, Ritze H-H, Radloff W, Hertel IV. Ultrafast predissociation dynamics of water molecules excited to the electronic C̃ and D̃ states. J Chem Phys (2004) 121:1765–70. doi:10.1063/1.1760732
19. Yuan K, Cheng L, Cheng Y, Guo Q, Dai D, Yang X. Two-photon photodissociation dynamics of H2O via the D̃ electronic state. J Chem Phys (2009) 131, 074301. doi:10.1063/1.3168398
20. Lin G-S-M, Zhou L, Xie D. Theoretical study of the state-to-state photodissociation dynamics of the vibrationally excited water molecule in the B band. J Phys Chem A (2014) 118:9220–7. doi:10.1021/jp503062s
21. van Harrevelt R, van Hemert MC. Quantum mechanical calculations for the H2O + hν → O(1D) + H2 photodissociation process. J Phys Chem A (2008) 112:3002–9. doi:10.1021/jp711857w
22. Slanger TG, Black G. Photodissociative channels at 1216 Å for H2O, NH3, and CH4. J Chem Phys (1982) 77:2432–7. doi:10.1063/1.444111
Keywords: photodissociation, semi-classical, surface-hopping, potential energy surface, non-adiabatic dynamics
Citation: Peng Y, Hu X, Wu Y, Wang J, Lu R and Sisourat N (2023) Photodissociation of water molecule at short photon wavelengths: Dynamical studies. Front. Phys. 11:1098119. doi: 10.3389/fphy.2023.1098119
Received: 14 November 2022; Accepted: 21 February 2023;
Published: 10 March 2023.
Edited by:
Libin Fu, Graduate School of China Academy of Engineering Physics, ChinaReviewed by:
Vinodkumar P. C., Sardar Patel University, IndiaVaibhav Prabhudesai, Tata Institute of Fundamental Research, India
Meishan Wang, Ludong University, China
Copyright © 2023 Peng, Hu, Wu, Wang, Lu and Sisourat. This is an open-access article distributed under the terms of the Creative Commons Attribution License (CC BY). The use, distribution or reproduction in other forums is permitted, provided the original author(s) and the copyright owner(s) are credited and that the original publication in this journal is cited, in accordance with accepted academic practice. No use, distribution or reproduction is permitted which does not comply with these terms.
*Correspondence: Yong Wu, d3VfeW9uZ0BpYXBjbS5hYy5jbg==; Ruifeng Lu, cmZsdUBuanVzdC5lZHUuY24=