- 1School of Life Sciences, Beijing University of Chinese Medicine, Beijing, China
- 2State Key Laboratory of Brain and Cognitive Science, Institute of Biophysics, Chinese Academy of Sciences, Beijing, China
- 3National Laboratory of Biomacromolecules, Institute of Biophysics, Chinese Academy of Sciences, Beijing, China
- 4University of Chinese Academy of Sciences, Beijing, China
Increasing numbers of behavioral observations have shown that many organisms can sense magnetic fields in the environment. However, the underlying mechanisms of magnetosensation remain elusive, and a major problem is the lack of an animal model convenient for detecting magnetic responses, especially in vertebrates. We have previously reported that the physical performance of mice decreased after 1 month of exposure to a hypomagnetic field, which probably includes a light-independent mechanism. In this study, we investigated the effects of a static magnetic field on the locomotion of Xenopus tadpoles (stage 48) using a real-time recording system. Because it is speculated that the photosensing system is related to magnetosensation, we triggered the tadpoles to swim by a 30 s light pulse after a 5 min adaptation in the dark and measured the swimming activities of the tadpoles under static magnetic field exposure in both the dark and bright conditions. Compared to the sham control treatment, the presence of a magnet inhibited the movement of the tadpoles under both bright and dark conditions, as shown by reductions in swimming distance, speed, and counts of path adjustment. Moreover, the directional preference for path adaptation was altered when swimming in the dark. These results suggest that tadpoles in the dark can exert a rapid locomotion response to changes in the environmental magnetic field, providing evidence for an intrinsic, light-independent rapid magnetoresponse pathway in tadpoles. In addition, this rapid vertebrate locomotion assay paradigm will be a practical tool to facilitate further investigation of the mechanisms of biomagnetic effects.
1 Introduction
How organisms perceive and respond to magnetic fields is a fundamental question that remains to be answered. Accumulating behavioral evidence has shown that many organisms can sense environmental magnetic fields. The movement of magnetotactic bacteria and the geomagnetic navigation of insects, fish, and migratory birds [1] show that organisms can use geomagnetic field (GMF) information to coordinate their locomotion. Our recent studies have shown that elimination of the GMF, i.e., the hypomagnetic field (HMF), induces changes in the circadian rhythms of drinking behavior, physical performance, and anxiety-related behavior in mice, accompanied by metabolic changes [2–5], suggesting that the GMF is required for the maintenance of the normal animal behavior. Although most studies have focused on the effects of GMF direction on animal behavior [6–9], accumulating evidence has also been shown that animal behavior can respond to changes in the intensity of the GMF [10–13]. However, the understanding of the mechanism of magnetoresponse in relation to magnetic field intensity is still very limited.
It has been reported that the photosensing proteins, cryptochromes (CRYs), are involved in animal magnetoreception. Ritz et al. indicated that the photoreceptor cryptochrome is part of the magnetoreception system [14]. Gegear et al. provided the first genetic evidence for a CRY-based magneto-sensitive system in Drosophila [15]. Foley et al. used a transgenic approach to demonstrate that human CRY2, which is highly expressed in the retina, can function as a magnetosensor in the magnetoreception system of Drosophila [16]. Wan et al. showed that CRY1 from Drosophila and monarch butterflies plays a key role in magnetosensation in a light-dependent manner [17]. However, because ultraviolet (UV)-A/blue light is required for photosensing CRYs to form magnetically sensitive radical pairs [16–19], GMF driven navigation of animals at night could not be fully explained by the light-dependent mechanism.
It has also been reported that non-photosensing proteins/particles are involved in magnetosensation. Magnetite is a commonly recognized magnetoreceptor that can explain orientation-dependent magneto-sensing [20–25]. Structural proteins and enzymes may also respond to the HMF. We have found that exposure to the HMF disrupts the in vitro assembly of cytoskeletal proteins, tubulin, and actin [26, 27], accelerates the denaturation of Cu, Zn-Superoxide Dismutase (CuZn-SOD) [28], and increases the activity of lactate dehydrogenase (LDH), a key enzyme in glycolysis [5]. Therefore, it is worth investigating whether other light-independent factors besides CRY and CRY-related signaling pathways, are involved in the general response of animal behavior to the environmental magnetic field.
The lack of a simple and robust in vivo magnetosensation model suitable for genetic modification and behavioral testing is a significant limitation to the study of magnetosensation. We have previously monitored mouse behavior in an HMF and found that a 3 days exposure causes anxiety [29], and a 1-month exposure can reduce physical performance [3, 4]. However, these HMF effects are accumulative, require long-term exposure, and do not lend themselves to the detection of rapid intrinsic magneto-sensing behavior. For the behavioral effects observed in other applied magnetic fields, a real-time behavioral recording system for immediate monitoring and analysis under specific magnetic field conditions is lacking [6, 10, 25, 30–35].
The African clawed frog (Xenopus laevis) is a commonly used vertebrate model. Xenopus embryos are a suitable material for functional analysis. They develop rapidly in vitro, are easy to transgene and detect their effect, and it is easy to obtain large numbers of embryos. The small size of the tadpoles also allows simultaneous treatment and behavioral observation of multiple individuals in a limited workspace. We have previously observed that Xenopus embryos in an HMF exhibit abnormal embryonic development [36], indicating that they are good candidates for the examination of the effect of environmental magnetic fields and are suitable for further investigation of the genetic basis of bio-magnetic effects.
In this study, we set up a suitable paradigm for analyzing the locomotion of the Xenopus tadpole to investigate its rapid response to an applied static magnetic field (SMF) under both dark and bright conditions. Because the magnetoresponse in animals is thought to be closely related to the photosensing system, tadpoles at stage 48 with distinct photo-motor sensations were used. Tadpoles were exposed to a medium-intensity (1mT - 1T) SMF generated by commercially available neodymium iron boron (NdFeB) magnets, commonly used in magnet therapy and mechanistic studies in animals [37, 38]. With the light-dependent hypothesis of magnetosensation, magnetic responses should be detectable only under the light condition rather than in the dark. While with the light-independent hypothesis, the magnetoresponse should be observed in both the light and dark conditions. Furthermore, If the signaling of magnetoresponse is synergistic with the photosensing system, the light-triggered response, e.g. photo-motor sensations, would be affected (amplified/weakened) by the magnetic stimulation, i.e., exhibiting magnetoresponse. Using this experimental system, we aimed to characterize the behavioral response of tadpoles to the SMF and evaluate the role of light in the magnetic response of tadpoles. We find a light-independent rapid locomotion response to the static magnetic field, which provide clues to the mechanism of the magnetoresponse with a practical in vivo paradigm for further studies.
2 Material and methods
2.1 Xenopus tadpole preparation
Xenopus tadpoles (stage 48) were used in this study. Xenopus embryos were prepared for in vitro fertilization according to a previously published protocol [39]. Embryos were cultured with 0.1 × MMR solution (10 mM NaCl; 0.2 mM KCl; 0.2 mM CaCl2; 0.1 mM MgCl2; 0.5 mM HEPES, pH 7.4). Progression of development was monitored at a temperature of 14–24°C. Embryonic stages were determined as previously described [40, 41]. All animal experimental procedures were performed according to institutional ethical guidelines (grant No. SYXK2014-30).
2.2 Magnetic field conditions
Tadpoles were tested in 12-well plates containing 3 ml of 0.1 × MMR solution. As shown in Figure 1, six wells around the central zone were selected as test arenas. Two stacks of NdFeB permanent magnets and nickel coins, both wrapped in tin foil, were placed in the two central wells for the SMF (magnetic) and sham treatment, respectively. No objects were placed in the blank GMF control. The environmental magnetic field (MF) on the blank and sham control plates was measured using a 3-axis fluxgate magnetometer constructed by the National Space Science Center (NSSC), of the Chinese Academy of Sciences (CAS). The magnetic properties of the SMF were measured using a Teslameter (HTME tech, HT20, Shanghai, China).
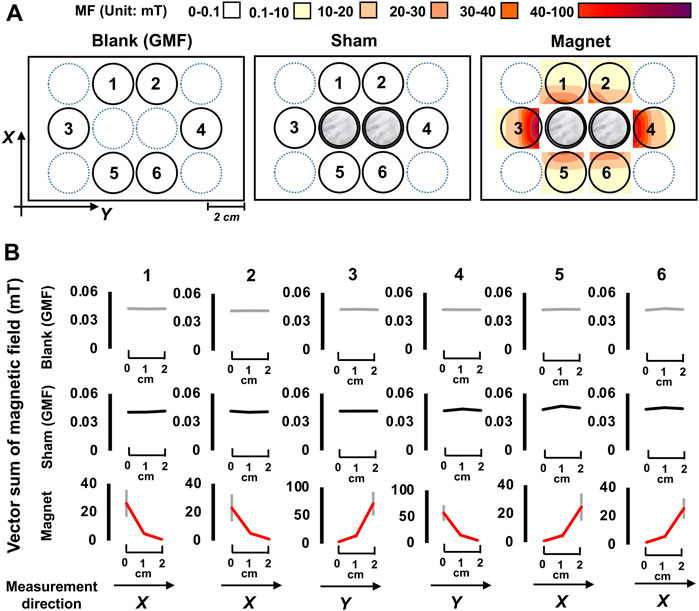
FIGURE 1. The experimental setup and magnetic field conditions. (A)The distributions of the magnetic field (vector sum) at the bottom plane of a 12-well plate for the blank, sham, and magnetic treatments are shown. Tadpoles were placed in wells with Arabic numerals 1 to 6. The central two wells (in gray) were the positions for magnetic (magnets) or sham (nickel coins) treatments. Black arrows indicate direction of the magnetic field. X-axis, South to North; Y-axis, East to West, MF, magnetic field. (B) The distribution of magnetic field vector sum along the X- or Y-axis, data are shown as mean ± standard deviation (SD) of the vector sum in each test well.
In the sham treatment, tin-foil-wrapped nickel coins of the same size and appearance as the tin-foil-wrapped magnets were used to control non-magnetic factors. The presence of the sham nickel stacks had no effect on the MF. The MF intensity in the test arenas under the blank and sham conditions was 0.042 ± 0.001 mT and 0.043 ± 0.005 mT, respectively, corresponding to the GMF level (0.04–0.06 mT). Under the SMF condition, it exhibited a high to low gradient along the central-to-peripheral direction. The gradients were at the same level in arenas 1, 2, 5, and 6 (0.1–30 mT), and became stronger in arenas 3 and 4 (0.1–80 mT) (Figure 1). The directions of the magnet-generated MF in the test arenas were largely downwards and northwest (in contrast to the blank and sham, that were downwards, and northeast).
2.3 Locomotion test
Locomotion of the stage 48 tadpoles was monitored using a Zebralab system (Viewpoint, France) in six test wells of the plate, with one tadpole per well. The temperature was constant at around 25°C. The length of a tadpole is about 15mm, and the width is about 2.5mm, the body size accounts for about 1/10 volume of the test well, so tadpoles have ample space for movement. The tadpole locomotion test lasted a total of 10 min: a 5 min dark adaptation followed by 5 min alternating light (full spectrum of visible light, 900 lx) pulse stimulation with 30 s light on and 30 s off (dark) (Figure 2A). Prior to each test, tadpoles were transferred to the test arenas for a 5–10 min accommodation period. Swimming tracks were recorded with an infrared camera, especially under dark conditions.
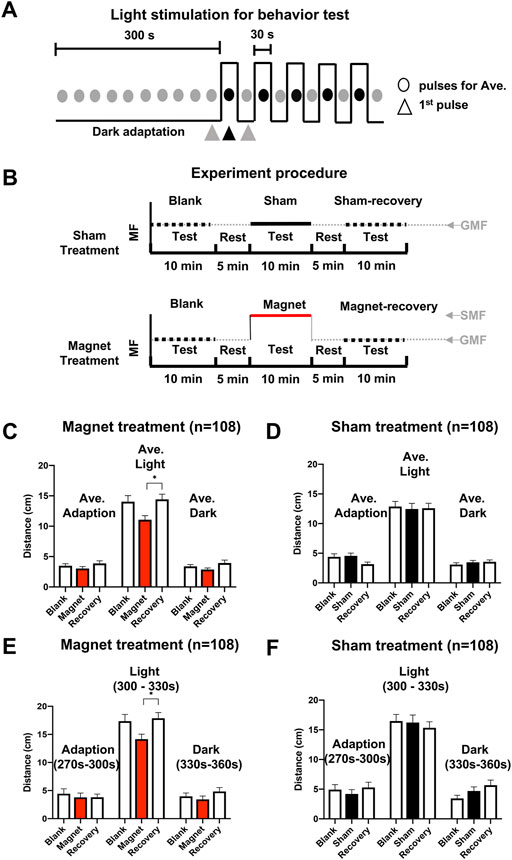
FIGURE 2. SMF-induced immediate and reversible inhibition of tadpole swimming. (A) The light stimulation mode for the behavioral test, which includes a 300 seconds (s) adaption in the dark and 5 pairs of light-dark pulses. Each circle represents 30 s, black and gray represent a stimulation in the light and an adaptation in the dark, respectively. Triangles represent the last 30s of the dark adaptation period (270–300 s) and the first light pulse: the 30 s light stimulation period (300–330 s), and the dark period (330–360 s), respectively, for data analysis. (B) The experimental procedure for sequential tests under blank, sham, or magnetic, and blank (recovery) conditions. The horizontal axis represents test/rest time. The vertical axis represents the relative magnetic field strength of different treatment conditions. The black dotted line represents the relative magnetic field strength without a nickel/magnet during the test. The black and red lines represent the relative magnetic field strength with the presence of a nickel or the magnet during the test, respectively. A “test” means a complete locomotion observation by using the light stimulation mode described in panel (A) (C,D) Effect of the SMF (C) and sham (D) treatments on the average swimming distance of tadpoles. (E,F) Effect of the SMF (E) and sham (F) treatment on the swimming distance of tadpoles in the last 30 s of the dark adaptation period (270–300 s), the 30 s light stimulation period (300–330 s), and the dark period (330–360 s). n is the total number of tadpoles from six experiments, eighteen tadpoles per experiment. Data are expressed as mean ± SEM. The p values were calculated using the Kruskal–Wallis test. *p < 0.05, **p < 0.01, ***p < 0.001.
In the first experimental procedure, a single tadpole was tested sequentially under different conditions, blank-sham or magnet-blank (recovery) (Figure 2B), and the average swimming distance in the dark and light was calculated. Additionally, the swimming distance was calculated in the last 30 s of the dark adaptation period (270–300 s), first 30 s light stimulation period (300–330 s), and dark period (330–360 s). The blank tests before the treatments served as a quality control to ensure that the tadpoles exhibited normal photomotor responses (Supplementary Figure S1).
For the second experiment, the same batch of tadpoles was tested under the sham and magnetic conditions sequentially (Figure 3B). Data from the last 30 s of the dark adaptation period (270–300 s) and the first 30 s of the light stimulation period (300–330 s) were collected for each test.
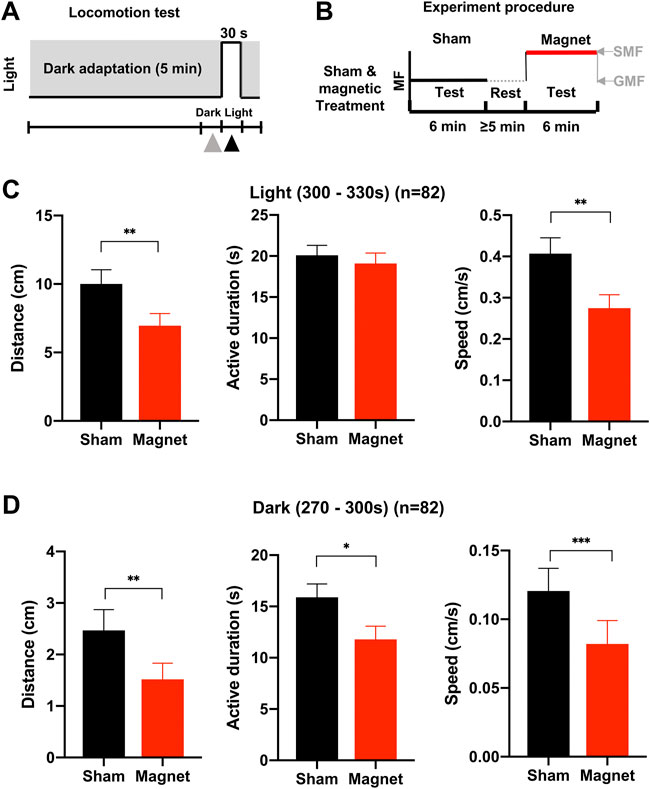
FIGURE 3. The effect of the SMF on the swimming behavior of tadpoles differs between light and dark conditions. (A) The light stimulation mode for the behavioral test. The triangles represent the last 30s of the dark adaptation period (270–300 s) and the first light pulse (the 30 s light stimulation period (300–330 s), respectively, for data analysis. (B) The experimental procedure for sequential tests under sham and magnetic conditions. The horizontal axis represents test/rest time. The vertical axis represents the relative magnetic field strength of different treatment conditions. The black dotted line represents the relative magnetic field strength without a nickel/magnet during the test. The black and red lines represent the relative magnetic field strength with the presence of a nickel and the magnet during the test, respectively. A “test” means a complete locomotion observation by using the light stimulation mode described in panel (A) (C,D) Effects of the SMF treatment on locomotion indexes of tadpoles in the dark (C) or in the light (D). SMF inhibits tadpole swimming distance and speed in the light condition, and the swimming distance, active duration, and speed in the dark. n is the total number of tadpoles from seven experiments, twelve tadpoles per experiment. Data are expressed as mean ± SEM. The p values were calculated using the Wilcoxon matched-pairs signed-rank test. *p < 0.05, **p < 0.01, ***p < 0.001.
2.4 Locomotion indexes
Locomotion indexes for movement intensity and path adaptation were determined using Viewpoint tracking and locomotion analysis software (Viewpoint, France). The intensity indexes include moving distance, speed, and active duration (instantaneous speed ≥0.25 cm/s). Indexes for path adjustment included path angle adjustment and the number of adjustments at each angle.
2.5 Data analysis
All experiments were performed independently at least six times, with 12–18 tadpoles per experiment. Tadpoles showing no large movement during the blank test were excluded. Means were calculated with all tadpoles from the experiments, data are expressed as mean ± standard error of the mean (SEM) unless otherwise stated. The Kruskal–Wallis test was used for the mean comparison of nonparametric data between three or more groups, like the distance in Figures 2C–F. The Wilcoxon matched-pairs signed-rank test was used for comparison of nonparametric data between two groups, such as swimming distance, active duration, speed, and path adjustment (count) in Figure 3 and Figures 4A,B. Two-way analysis of variance (ANOVA) was used to compare data meeting the assumption of normal distribution like the path angle adjustment details in Figures 4F,G. Differences were considered statistically significant at p < 0.05. GraphPad Prism 8 was used for statistical analysis and graphical plots.
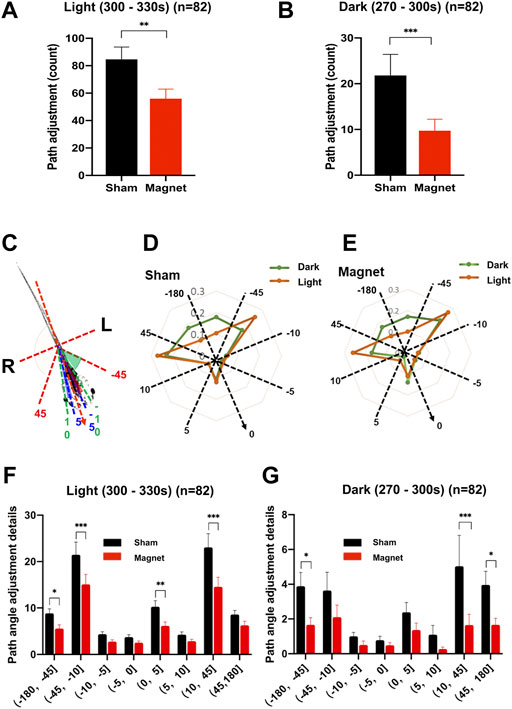
FIGURE 4. The SMF reduced counts of path angle adjustment of swimming tadpoles. (A,B) SMF-induced reduction in total counts of path angle adjustments in light (A) and dark (B). (C) Schematic diagram illustrating the direction and angles adjusted during swimming. The centerline along the body axis from head to tail refers to the previous direction of locomotion. The directional adjustment to the right (R) of the body is determined as positive (+) and to the left (L) as negative (-). (D,E) Path angle adjustment pattern of the tadpoles shows no difference between the sham (D) and the magnetic (E) treatment shown by the spider plot. The dashed lines divided the groups with angle setting of (0°–5°], (5°–10°], (10°–45°], (45°–180°]. The orange and green dot lines show the ratio of adjustment counts in each quadrant compared to the total counts during the light pulse and dark interval, respectively. (F) The SMF inhibits the counts of left sided large-angle (45–180°), both right and left sided medium-angle (10–45°), and right sided direct-angle (0–5°) path adjustments in the light. (G) The SMF inhibits the counts of both right and left sided large-angle (45–180°) and right-sided medium-angle (10–45°) path angle adjustments in the dark. n is the total number of tadpoles from seven experiments, twelve tadpoles per experiment. Data are expressed as mean ± SEM. The p values were calculated using the Wilcoxon matched-pairs signed-rank test (A,B) and the two-way ANOVA (F,G) test. *p < 0.05, **p < 0.01, ***p < 0.001.
3 Results
3.1 The SMF-induced immediate and reversible inhibition of tadpole swimming
To investigate the effect of the SMF condition on the swimming behavior of the tadpoles, we first used an experimental procedure with different tadpoles for the sham and magnetic treatments (Figures 2A,B). As showing in the swimming distance test, the tadpoles, after sham or magnetic treatment (Figures 2C–F), exhibited typical photo-motor responses with immediate more active movement during light stimulation than in darkness, and the distance level could recover when the magnet was removed.
For the tadpoles in the magnetic group, the average swimming distance during the 30 s light pulses showed a decreasing trend during the tests compared to those in the dark adaptation (−21%, p = 0.2184); and when the magnet was removed, the swimming distance recovered to the level of the dark adaptation test before treatment (p = 0.9396). Although tadpole locomotion also exhibited a magnet-induced decreasing trend during testing in both the dark adaptation period (−13%) and the 30s dark pulses (−15%) after the light, the magnet-induced changes in swimming distance were not statistically significant compared to either the blank or recovery conditions (Figure 2C).
In the sham treatment, no significant changes were observed in any of the experimental tests, under either bright or dark conditions (Figure 2D). The results indicate that SMF has an inhibitory effect on the locomotion of tadpoles and that the SMF effect is immediate and reversible.
To further confirm the immediate and reversible SMF effect, we analyzed the distance in the last 30 s of the dark adaptation period (270–300 s), the first 30 s of the light stimulation period (300–330 s), and the dark period (330–360 s) in detail (Figures 2E,F). These results were consistent with those obtained from the average swimming distance. During the first 30 s light pulse, the presence of the SMF tended to decrease the swimming distance of the tadpoles (- 18%), but this was not statistically significant compared to the blank condition (p = 0.0778). However, swimming activity returned to normal when the SMF was removed from the tadpoles (p = 0.0345) (Figure 2E). We suggest that the tadpoles may be more sensitive to recovery from the SMF condition than from the weak GMF condition. During the first 30 s dark pulse, tadpoles in the presence of the magnet also exhibited a weak decreasing tendency (- 15%) in swimming activity, but no statistical difference from that in the blank and recovery condition, consistent with the analysis using mean swimming distance. The sham treatment showed the same negative effect on swimming distance as shown by the mean distance (Figure 2F).
3.2 SMF exerts locomotion reduction both in dark and bright conditions
To demonstrate SMF-induced inhibition of locomotion, we used the second experimental procedure in the subsequent experiments: for the same batch of tadpoles, each tadpole was tested under the “sham” and “magnet” conditions sequentially (Figure 3B), which provides a better investigation of the SMF effect on the swimming behavior by avoiding possible variations due to differences between individual tadpoles. To compare the effect of SMF in more detail, two additional parameters, active duration and swimming speed, were used in the following experiments.
We observed that SMF inhibited the locomotion of tadpoles in a different pattern under dark and bright conditions. Under the bright conditions, tadpoles maintained a similar active swimming duration, but swimming intensity, i.e., distance (−31%, p = 0.0025) and swimming speed (−32%, p = 0.0015), was significantly inhibited by the presence of the magnet (Figure 3C). All three locomotion parameters showed statistical significance decreased during the 30s dark pulse (distance: − 26%, p = 0.0011; active duration: − 26%, p = 0.0155; speed: − 32%, p = 0.0007) (Figure 3D). These results confirm the inhibitory effect of SMF on tadpole locomotion and indicate that the inhibitory pattern of SMF differs between light and dark conditions.
3.3 The SMF weakens the path adjustment behavior and alter the direction preference in dark
The above results showed that the inhibitory effect of the SMF was observed even at low locomotion when the tadpoles rested in the dark, suggesting that the SMF may act on small-scale movement. To test this hypothesis, we examined the effect of the SMF on the fine movement of tadpoles, i.e., their directional adaptation during swimming, using the second experimental procedure (Figures 3A,B).
The total number of path directional adaptations were significantly reduced in both the bright (−34%, p = 0.0015) and dark (−55%, p = 0.0001) conditions under the SMF (Figures 4A,B), consistent with our hypothesis above, and the reduction are greater than that of the three locomotion parameters analyzed above.
When we break down the distribution of the number of directional adaptations by different angular intervals, we find that tadpoles under bright and dark conditions have different habits adapting the path to the angle. Figures 4C–E shows: 1) more large-angle (45–180°) adjustments in darkness; 2) medium-angle (10–45°) adjustments were preferred by the tadpoles in both the bright and dark conditions, and showed more right preference (+, 23.1%) than left preference (-, 16.7%) in darkness; 3) a right-forward small-angle (0–5°) adjustment preference was shown by the tadpoles in both the bright and dark conditions. In addition, further analysis revealed that, although there was no statistical difference, the tadpoles in the sham group showed a right-sided preference in the medium-angle (10–45°) and small-angle (0–5°) adjustments; whereas in the presence of a magnet, the right-sided preference of tadpoles was reversed to the left-sided preference (-, 21.3% vs + 17.9%). This path angle adjustment preference is not evident in bright conditions (Supplementary Figure S2).
In the presence of a magnet, path angle adjustments of tadpoles showed a general downward trend at all angles under both bright and dark conditions (Figures 4F,G). In the light, the SMF had an obvious inhibitory effect on the left-sided large-angle (45–180°) adjustments; for the dominant medium-angle (10–45°) adjustment, the number of angle adjustments were significantly reduced by the SMF exposure in both the left (- 30%, p = 0.0005) and right ranges (37%, p < 0.0001), indicating no left-right preference. The SMF significantly suppressed the small-angle (0–5°) adjustments (- 40%; p = 0.0046), which had a pronounced right-sided preference in the sham group (Figure 4F). In the dark condition, the SMF-exposure significantly reduced the number of large-angle (45–180°) adjustments (left: 57%, p = 0.0268; right: 58%, p = 0.0196), with no left-right preference, and the SMF-exposure significantly reduced only the number of the right-sided medium-angle (10–45°) adjustments (- 67%, p = 0.0046) (Figure 4G). The results confirmed that the fine movement of the tadpoles and path angle adjustment behavior during swimming were inhibited by the SMF.
4 Discussion
In this study, we demonstrated an immediate, reversible, and light-independent magnetic response in Xenopus tadpoles with mature light-sensing ability (St. 48). The effects were demonstrated in a locomotion test at 30s light plus 30 s dark, and the assay was completed with two tests under sham and magnetic conditions. In the presence of the SMF, a sharp decrease in locomotion activity was observed under both dark and light conditions (Figures 2–4), indicating that light is not a critical factor in the tadpoles’ behavioral response to an SMF. The SMF-weakened swimming behavior was restored shortly after the magnet was removed. Tadpole swimming distance of the tadpoles returned to the normal levels almost immediately in the subsequent GMF blank recovery test less than 5 min after SMF exposure (Figure 2). The rapid and reversible behavioral SMF response in Xenopus would be an ideal behavioral phenomenon for further investigation of the neural and molecular mechanisms of biomagnetic effects.
Recent studies have provided evidence supporting the existence of a light-independent magnetic response mechanism in animals. For example, Fukomys mechowii and Heliophobius argenteocinereus can obtain directional information from a magnetic field even in the dark [42]. Myklatun et al. also pointed out that adult fish can orient themselves according to the direction of the magnetic field even in a dark and unfamiliar environment [8]. Our results showed that SMF-induced reductions in key locomotion indexes (distance speed, active duration, and number of path angle adjustments) were recorded even during low-intensity swimming of tadpoles in darkness, and these changes were statistically significant. Overall, by examining the SMF effect on fine movement in detail, we confirmed the inhibitory effect of the SMF in the tadpole under dark conditions, which is consistent with our hypothesis that light-independent SMF-sensation exists in the tadpole. In addition, it has been reported that GMF-perceptive bats rely more heavily on information from light for navigation under bright conditions, such as using sunsets to calibrate a magnetic compass [43]. In other words, under light conditions, animals are less dependent on the magnetic field than on information provided by light, whereas in the dark, when there is no light information, animals are more sensitive to changes in the magnetic field, which is consistent with our experimental results. In commonly used animal models, tadpoles are cheap, easy to obtain, and amenable to genetic manipulation. Therefore, our experimental paradigm will help overcome the lack of animal models suitable for studying the mechanism of instantaneous response to the magnetic field.
Although we observed that light is not a necessary factor for the SMF-effect in the tadpoles, we also found that the SMF-response pattern in the light was different from that in the dark, showing no left-right preference, and was easily detected with a regular parameter (swimming distance). Since the photosensing system would trigger both locomotion and magnetoresponse, light could be a possible factor whose effects mask those of the magnet on directional preference in the light. As the locomotion activity of the tadpoles was much more elevated by light stimulation, it was not easy to detect whether the SMF effect was left-right biased in the light conditions. Interestingly, recent studies have indicated that the strength of the magnetic field has a more important effect on bird navigation than the inclination [44]. Therefore, it will be interesting to explore how the magnetic field strength is perceived in the dark using this model, which will help to unravel the mystery of magnetoreception.
Many reports have shown that most bio-magnetic responses of animals are weak but accumulative, so that a long-term magnetic field exposure would promise the accumulation of sufficient modifications at transcription, expression, or signal transduction levels leading to a detectable bio-magnetic response [45–48]. However, the immediate and reversible response of tadpoles to SMF exposure observed in our experiments suggests that a rapid biological process serves as the basis for the mechanism rather than the time-consuming processes mentioned above. Magnetic fields have been shown to affect energy metabolism. For example, magnetic field exposure can cause changes in ATP content, decreased mitochondrial activity, and changes in the mitochondrial membrane potential [2, 49–53]. Exercise is an energy-consuming process and energy metabolism is a fast and efficient process. Therefore, it is reasonable to study the mechanisms from the perspective of energy metabolism. In addition, magnetic fields have been reported to be related to neural conduction, for example, by affecting the activity of neurons or ion channels [54–57]. Therefore, nerve conduction is a potential target for magnetic fields. Moreover, based on the current experimental evidence, we could not exclude the possibility that CRYs are involved in the SMF-inhibitory effect. It has been shown that type 2 CRYs could also function as magnetosensors even in the dark [58, 59]. It is hypothesized that CRY undergoes a magnetically sensitive radical-pair step in the light, and there are a large number of activated CRY molecules, so magnetic sensitivity in the dark is possible [58, 60]. In the future, it is hoped that this model can be combined with real-time electrical activity detection and other technologies to determine the neural circuitry of biological magnetoreception.
In summary, we have demonstrated a rapid and light-independent SMF response behavior in tadpoles. The SMF significantly reduces the locomotion intensity, and the tadpoles can perceive changes of the magnetic field, even in the dark. In addition, the SMF was able to alter the tadpoles’ movement angle adjustment preference pattern in the dark. Our experimental paradigm provides novel behavioral evidence for the intrinsic light-independent magnetic response and clues to uncover the mechanism of the magnetoresponse with a practical system in vivo for further investigation.
Data availability statement
The original contributions presented in the study are included in the article/Supplementary Material, further inquiries can be directed to the corresponding authors.
Ethics statement
The animal study was reviewed and approved by institutional ethical guidelines (grant No. SYXK2014-30).
Author contributions
YL, RH, and TJ contributed to the study design. WM, HZ, and XW performed the experiments. WM, JR, YL analyzed the data. WM, JR, YL and RH wrote the manuscript. All authors read and approved the final manuscript.
Funding
This study was supported by the National Nature Science Foundation of China (Grant Nos. 31870840 and 31271387) and SS Application project (No. YYWT-0801-EXP-13).
Acknowledgments
We thank MX for technical assistance in frog manipulation.
Conflict of interest
The authors declare that the research was conducted in the absence of any commercial or financial relationships that could be construed as a potential conflict of interest.
Publisher’s note
All claims expressed in this article are solely those of the authors and do not necessarily represent those of their affiliated organizations, or those of the publisher, the editors and the reviewers. Any product that may be evaluated in this article, or claim that may be made by its manufacturer, is not guaranteed or endorsed by the publisher.
Supplementary material
The Supplementary Material for this article can be found online at: https://www.frontiersin.org/articles/10.3389/fphy.2022.995860/full#supplementary-material
References
1. Clites BL, Pierce JT. Identifying cellular and molecular mechanisms for magnetosensation. Annu Rev Neurosci (2017) 40:231–50. doi:10.1146/annurev-neuro-072116-031312
2. Fu JP, Mo WC, Liu Y, He RQ. Decline of cell viability and mitochondrial activity in mouse skeletal muscle cell in a hypomagnetic field. Bioelectromagnetics (2016) 37:212–22. doi:10.1002/bem.21968
3. Hu PD, Mo WC, Fu JP, Liu Y, Rong-Qiao H. Long-term hypogeomagnetic field exposure reduces muscular mitochondrial function and exercise capacity in adult male mice. Prog Biochem Biophys (2020) 4:426–38. doi:10.16476/j.pibb.2020.0047
4. Mo WC, Fu JP, Ding HM, Liu Y, Hua Q, He RQ. Hypomagnetic field alters circadian rhythm and increases algesia in adult male mice. Prog Biochem Biophys (2015) 42:639–46. doi:10.16476/j.pibb.2015.0137
5. Wang G, Fu J, Mo W, Zhang H, Liu Y, He R. Shielded geomagnetic field accelerates glucose consumption in human neuroblastoma cells by promoting anaerobic glycolysis. Biochem Biophysical Res Commun (2022) 601:101–8. doi:10.1016/j.bbrc.2022.01.114
6. Ernst DA, Lohmann KJ. Effect of magnetic pulses on caribbean spiny lobsters: Implications for magnetoreception. J Exp Biol (2016) 219:1827–32. doi:10.1242/jeb.136036
7. Leucht T. Interactions of light and gravity reception with magnetic fields in Xenopus laevis. J Exp Biol (1990) 148:325–34. doi:10.1242/jeb.148.1.325
8. Myklatun A, Lauri A, Eder SHK, Cappetta M, Shcherbakov D, Wurst W, et al. Zebrafish and medaka offer insights into the neurobehavioral correlates of vertebrate magnetoreception. Nat Commun (2018) 9:802. doi:10.1038/s41467-018-03090-6
9. Pereira MC, Guimarães IC, Acosta-Avalos D, Antonialli Junior WF. Can altered magnetic field affect the foraging behaviour of ants? PLoS One (2019) 14:e0225507. doi:10.1371/journal.pone.0225507
10. Ernst DA, Lohmann KJ. Size-dependent avoidance of a strong magnetic anomaly in Caribbean spiny lobsters. J Exp Biol (2018) 221:jeb172205. doi:10.1242/jeb.172205
11. Shuo S, Yumeng YM, Leilei LL, Yanhui YH, Chao C, Hua H, et al. Static magnetic field induces abnormality of glucose metabolism in rats' brain and results in anxiety-like behavior. J Chem Neuroanat (2021) 113:101923. doi:10.1016/j.jchemneu.2021.101923
12. Wan G, Liu R, Li C, He J, Pan W, Sword GA, et al. Change in geomagnetic field intensity alters migration-associated traits in a migratory insect. Biol Lett (2020) 16:20190940. doi:10.1098/rsbl.2019.0940
13. Zhang YC, Wan GJ, Wang WH, Li Y, Yu Y, Zhang YX, et al. Enhancement of the geomagnetic field reduces the phototaxis of rice Brown planthopper Nilaparvata lugens associated with frataxin down‐regulation. Insect Sci (2020) 27:1043–52. doi:10.1111/1744-7917.12714
14. Ritz T, Adem S, Schulten K. A model for photoreceptor-based magnetoreception in birds. Biophysical J (2000) 78:707–18. doi:10.1016/S0006-3495(00)76629-X
15. Gegear RJ, Casselman A, Waddell S, Reppert SM. Cryptochrome mediates light-dependent magnetosensitivity in Drosophila. Nature (2008) 454:1014–8. doi:10.1038/nature07183
16. Foley LE, Gegear RJ, Reppert SM. Human cryptochrome exhibits light-dependent magnetosensitivity. Nat Commun (2011) 2:356. doi:10.1038/ncomms1364
17. Wan G, Hayden AN, Iiams SE, Merlin C. Cryptochrome 1 mediates light-dependent inclination magnetosensing in monarch butterflies. Nat Commun (2021) 12:771. doi:10.1038/s41467-021-21002-z
18. Gegear RJ, Foley LE, Casselman A, Reppert SM. Animal cryptochromes mediate magnetoreception by an unconventional photochemical mechanism. Nature (2010) 463:804–7. doi:10.1038/nature08719
19. Giachello CN, Scrutton NS, Jones AR, Baines RA. Magnetic fields modulate blue-light-dependent regulation of neuronal firing by cryptochrome. J Neurosci (2016) 36:10742–9. doi:10.1523/JNEUROSCI.2140-16.2016
20. Acosta-Avalos D, Esquivel DM, Wajnberg E, Lins de Barros HG, Oliveira PS, Leal I. Seasonal patterns in the orientation system of the migratory ant Pachycondyla marginata. Naturwissenschaften (2001) 88:343–6. doi:10.1007/s001140100245
22. Irwin WP, Lohmann KJ. Disruption of magnetic orientation in hatchling loggerhead sea turtles by pulsed magnetic fields. J Comp Physiol A (2005) 191:475–80. doi:10.1007/s00359-005-0609-9
23. Kirschvink JL, Gould JL. Biogenic magnetite as a basis for magnetic field detection in animals. Biosystems (1981) 13:181–201. doi:10.1016/0303-2647(81)90060-5
24. Walker MM, Quinn TP, Kirschvink JL, Groot C. Production of single-domain magnetite throughout life by sockeye salmon, Oncorhynchus nerka. J Exp Biol (1988) 140:51–63. doi:10.1242/jeb.140.1.51
25. Wiltschko W, Wiltschko R. Magnetic orientation and magnetoreception in birds and other animals. J Comp Physiol A (2005) 191:675–93. doi:10.1007/s00359-005-0627-7
26. Mo WC, Zhang ZJ, Wang DL, Liu Y, Bartlett PF, He RQ. Shielding of the geomagnetic field alters actin assembly and inhibits cell motility in human neuroblastoma cells. Sci Rep (2016) 6:22624. doi:10.1038/srep22624
27. Wang DL, Wang XS, Xiao R, Liu Y, He RQ. Tubulin assembly is disordered in a hypogeomagnetic field. Biochem Biophysical Res Commun (2008) 376:363–8. doi:10.1016/j.bbrc.2008.08.156
28. Zhang HT, Zhang ZJ, Mo WC, Hu PD, Ding HM, Liu Y, et al. Shielding of the geomagnetic field reduces hydrogen peroxide production in human neuroblastoma cell and inhibits the activity of CuZn superoxide dismutase. Protein Cell (2017) 8:527–37. doi:10.1007/s13238-017-0403-9
29. Ding HM, Wang X, Mo WC, Qin LL, Wong S, Fu JP, et al. Hypomagnetic fields cause anxiety in adult male mice. Bioelectromagnetics (2019) 40:27–32. doi:10.1002/bem.22155
30. Kiss B, Gyires K, Kellermayer M, László JF. Lateral gradients significantly enhance static magnetic field-induced inhibition of pain responses in mice-a double blind experimental study. Bioelectromagnetics (2013) 34:385–96. doi:10.1002/bem.21781
31. Nishimura T, Tada H, Fukushima M. Correlation between the lunar phase and tail-lifting behavior of lizards (Pogona vitticeps) exposed to an extremely low-frequency electromagnetic field. Animals (2019) 9:208. doi:10.3390/ani9050208
32. Osipova EA, Pavlova VV, Nepomnyashchikh VA, Krylov VV. Influence of magnetic field on zebrafish activity and orientation in a plus maze. Behav Process (2016) 122:80–6. doi:10.1016/j.beproc.2015.11.009
33. Romanovskij AV, Pesnya DS, Izvekov EI, Krylov VV, Nepomnyashchikh VA. The behavior of male Danio rerio Hamilton after exposure of fish embryos to a simulated geomagnetic storm. Biophysics (2014) 59:935–9. doi:10.1134/S0006350914060190
34. Vácha M, Půžová T, Kvíćalová M. Radio frequency magnetic fields disrupt magnetoreception in American cockroach. J Exp Biol (2009) 212:3473–7. doi:10.1242/jeb.028670
35. Xu J, Pan W, Zhang Y, Li Y, Wan G, Chen F, et al. Behavioral evidence for a magnetic sense in the oriental armyworm,Mythimna separata. Biol Open (2017) 6:340–7. doi:10.1242/bio.022954
36. Mo WC, Liu Y, Cooper HM, He RQ. Altered development of Xenopus embryos in a hypogeomagnetic field. Bioelectromagnetics (2012) 33:238–46. doi:10.1002/bem.20699
37. Ghodbane S, Lahbib A, Sakly M, Abdelmelek H. Bioeffects of static magnetic fields: Oxidative stress, genotoxic effects, and cancer studies. Biomed Res Int (20132013) 2013:1–12. doi:10.1155/2013/602987
38. Tasić T, Lozić M, Glumac S, Stanković M, Milovanovich I, Djordjevich DM, et al. Static magnetic field on behavior, hematological parameters and organ damage in spontaneously hypertensive rats. Ecotoxicology Environ Saf (2021) 207:111085. doi:10.1016/j.ecoenv.2020.111085
39. Sive HLGR, Harland RM. Early development of Xenopus laevis. 5th ed. New York: Cold Spring Harbor Laboratory Press (1997).
40. Bowes JB, Snyder KA, Segerdell E, Jarabek CJ, Azam K, Zorn AM, et al. Xenbase: Gene expression and improved integration. Nucleic Acids Res (2010) 38:D607–D612. doi:10.1093/nar/gkp953
41. Nieuwkoop PD, Faber J. Normal table of Xenopus laevis (daudin). New York: Garland Publishing (1994). p. 252.
42. Oliveriusová L, Němec P, Králová Z, Sedláček F. Magnetic compass orientation in two strictly subterranean rodents: Learned or species-specific innate directional preference? J Exp Biol (2012) 215:3649–54. doi:10.1242/jeb.069625
43. Holland RA, Thorup K, Vonhof MJ, Cochran WW, Wikelski M. Bat orientation using Earth's magnetic field. Nature (2006) 444:702. doi:10.1038/444702a
44. Zein B, Long JA, Safi K, Kölzsch A, Benitez-Paez F, Wikelski M, et al. Simulating geomagnetic bird navigation using novel high-resolution geomagnetic data. Ecol Inform (2022) 69:101689. doi:10.1016/j.ecoinf.2022.101689
45. Zhang B, Wang L, Zhan A, Wang M, Tian L, Guo W, et al. Long-term exposure to a hypomagnetic field attenuates adult hippocampal neurogenesis and cognition. Nat Commun (2021) 12:1174. doi:10.1038/s41467-021-21468-x
46. Fitak RR, Wheeler BR, Ernst DA, Lohmann KJ, Johnsen S. Candidate genes mediating magnetoreception in rainbow trout ( Oncorhynchus mykiss ). Biol Lett (2017) 13:20170142. doi:10.1098/rsbl.2017.0142
47. Ernst DA, Fitak RR, Schmidt M, Derby CD, Johnsen S, Lohmann KJ. Pulse magnetization elicits differential gene expression in the central nervous system of the Caribbean spiny lobster, Panulirus argus. J Comp Physiol A (2020) 206:725–42. doi:10.1007/s00359-020-01433-7
48. Fan Z, Hu P, Xiang L, Liu Y, He R, Lu T. A static magnetic field inhibits the migration and telomerase function of mouse breast cancer cells. Biomed Res Int (2020) 2020:1–9. doi:10.1155/2020/7472618
49. Calabrò E, Magazù S, Currò M, Ientile R. The inverse relation between mitochondrial transmembrane potential and proteins α-helix in neuronal-like cells under static magnetic field and the role of VDAC. Electromagn Biol Med (2020) 39:176–82. doi:10.1080/15368378.2020.1737808
50. Gurhan H, Bruzon R, Kandala S, Greenebaum B, Barnes F. Effects induced by a weak static magnetic field of different intensities on HT‐1080 fibrosarcoma cells. Bioelectromagnetics (2021) 42:212–23. doi:10.1002/bem.22332
51. Wang D, Wang Z, Zhang L, Li Z, Tian X, Fang J, et al. Cellular ATP levels are affected by moderate and strong static magnetic fields. Bioelectromagnetics (2018) 39:352–60. doi:10.1002/bem.22122
52. Gorczynska E, Galka G, Wegrzynowicz R, Mikosza H. Effect of magnetic field on the process of cell respiration in mitochondria of rats. Physiol Chem Phys Med NMR (1986) 18:61–9.
53. Zhao G, Chen S, Wang L, Zhao Y, Wang J, Wang X, et al. Cellular ATP content was decreased by a homogeneous 8.5 T static magnetic field exposure: Role of reactive oxygen species. Bioelectromagnetics (2011) 32:94–101. doi:10.1002/bem.20617
54. Nikolic L, Bataveljic D, Andjus PR, Nedeljkovic M, Todorovic D, Janac B. Changes in the expression and current of the Na+/K+ pump in the snail nervous system after exposure to static magnetic field. J Exp Biol (2013) 216:3531–41. doi:10.1242/jeb.085332
55. Nikolić L, Todorović N, Zakrzewska J, Stanić M, Rauš S, Kalauzi A, et al. Involvement of Na+/K+pump in fine modulation of bursting activity of the snail Br neuron by 10 mT static magnetic field. J Comp Physiol A (2012) 198:525–40. doi:10.1007/s00359-012-0727-0
56. Vidal-Gadea A, Ward K, Beron C, Ghorashian N, Gokce S, Russell J, et al. Magnetosensitive neurons mediate geomagnetic orientation in Caenorhabditis elegans. Elife (2015) 4:e07493. doi:10.7554/eLife.07493
57. Kavaliers M, Ossenkopp KP. Magnetic fields and stress: Day-night differences. Prog Neuro-Psychopharmacology Biol Psychiatry (1987) 11:279–86. doi:10.1016/0278-5846(87)90071-6
58. Netušil R, Tomanová K, Chodáková L, Chvalová D, Doležel D, Ritz T, et al. Cryptochrome-dependent magnetoreception in a heteropteran insect continues even after 24 h in darkness. J Exp Biol (2021) 224:jeb243000. doi:10.1242/jeb.243000
59. Bazalova O, Kvicalova M, Valkova T, Slaby P, Bartos P, Netusil R, et al. Cryptochrome 2 mediates directional magnetoreception in cockroaches. Proc Natl Acad Sci U.S.A (2016) 113:1660–5. doi:10.1073/pnas.1518622113
Keywords: magnetoresponse, locomotion, light independent, xenopus tadpole, magnetic field, path adjustment, direction preference
Citation: Ren J, Mo W, Zhang H, He R, Wang X, Jiang T and Liu Y (2022) The light-independent locomotion response to a static magnetic field in Xenopus tadpoles. Front. Phys. 10:995860. doi: 10.3389/fphy.2022.995860
Received: 16 July 2022; Accepted: 01 September 2022;
Published: 30 September 2022.
Edited by:
Guijun Wan, Nanjing Agricultural University, ChinaReviewed by:
Xin Zhang, Hefei Institutes of Physical Science (CAS), ChinaPoornachandra Sekhar Burada, Indian Institute of Technology Kharagpur, India
Copyright © 2022 Ren, Mo, Zhang, He, Wang, Jiang and Liu. This is an open-access article distributed under the terms of the Creative Commons Attribution License (CC BY). The use, distribution or reproduction in other forums is permitted, provided the original author(s) and the copyright owner(s) are credited and that the original publication in this journal is cited, in accordance with accepted academic practice. No use, distribution or reproduction is permitted which does not comply with these terms.
*Correspondence: Rongqiao He, aGVycUBpYnAuYWMuY24=; Ying Liu, eWluZ2xpdUBidWNtLmVkdS5jbg==
†These authors share first authorship