- 1Department of Surgery, College of Medicine, The Pennsylvania State University, Hershey, PA, United States
- 2Department of Biomedical Engineering, College of Medicine, The Pennsylvania State University, Hershey, PA, United States
Biomaterial associated microbial infection and blood thrombosis are two of the barriers that inhibit the successful use of implantable medical devices in modern healthcare. Modification of surface topography is a promising approach to combat microbial infection and thrombosis without altering bulk material properties necessary for device function and without contributing to bacterial antibiotic resistance. Similarly, the use of other antimicrobial techniques such as grafting poly (ethylene glycol) (PEG) and nitric oxide (NO) release also improve the biocompatibility of biomaterials. In this review, we discuss the development of surface texturing techniques utilizing ordered submicron-size pillars for controlling bacterial adhesion and biofilm formation, and we present combinatorial approaches utilizing surface texturing in combination with poly (ethylene glycol) (PEG) grafting and NO release to improve the biocompatibility of biomaterials. The manuscript also discusses efforts towards understanding the molecular mechanisms of bacterial adhesion responses to the surface texturing and NO releasing biomaterials, focusing on experimental aspects of the approach.
1 Introduction
Medical devices are ubiquitous in modern health care. Devices ranging in complexity from simple catheters to valves, stents, and grafts, and even to highly complex artificial organs such as in-dwelling pumps, extra-corporeal membrane oxygenators and cardiac replacement devices such as ventricular assist devices and artificial hearts all require materials that come into significant contact with biological systems as an essential technology for these devices. Biomaterials exhibiting sufficient biocompatibility including hemocompatibility for both short- and long-term implantation remain a limiting factor in the development of advanced versions of these devices. After decades of focused research and literally thousands of publications, two important device associated complications, blood thrombosis [1–3] and microbial infection [4,5], remain significant barriers to the implementation of blood-contacting medical devices. Device associated thrombosis is initiated by both activation of the blood plasma coagulation cascade as device surfaces come into contact with blood and activate the zymogens of the coagulation cascade, and also from platelet adhesion/activation to plasma proteins adsorbed on the device surfaces. Infection is initiated by pathogenic bacterial adhesion to adsorbed proteins followed subsequently by biofilm formation and maturation [6–8]. Both of these important biological reactions are related to the materials used in these devices, but more importantly, they are specifically influenced by the surface properties of materials. The development of new biomaterials possessing multifunctional surfaces with anti-thrombotic and anti-microbial properties is going to be necessary for the continued development and application of biomaterials in advanced implantable medical devices.
While the bulk material properties such as strength and flexibility will usually determine the suitability of the material for an application in medical devices, the physical and/or chemical properties of the material surface are paramount to the successful function of many biomedical devices and are largely determinant of the biocompatibility. Surface engineering and modification becomes an important strategy to allow medical-device designers to retain desirable bulk properties while improving the biocompatibility [9,10]. There are many methods of surface modifications ranging from chemical [11,12] and physical [13,14] modifications of the original surface, and to the use of coatings as a biological-contacting layer [15,16]. Physical modification results in a change in the topography or morphology of the surface with little to no change in the chemistry, while chemical treatment results in surface functionalization, oxidization, or ion infusion, for example [17]. The goal of these approaches is to modify the material surface properties known to influence biocompatibility such as surface topography, water wettability (surface energy), surface chemistry, surface charge, surface chemical patterns, and roughness, in order to create a chemical and/or physical environment that offers a favorable response to hard or soft tissue while simultaneously minimizing adverse responses such as pathogenic infections or thrombosis while contacting with blood, thereby improving the success of these biomaterials in medical applications.
Surface topographical modification is an attractive research field in improvement of biological responses to implanted devices with unique cell-protein-surface interactions [18]. When a medical device is implanted into the body, proteins from blood, interstitial fluid, or other fluid sources will immediately adsorb on material surfaces and form a layer that influences the subsequent cell interactions. Surface topography such as grooves and ridges or pits and pillars can have a dramatic effect on both the plasma protein adsorption and the subsequent cell-surface interactions [19,20]. Numerous experimental studies have shown that macro, micro, and even nanoscale features mediate the adherence, proliferation, and migration of cells [21–25]. Surface topography also influences bacterial adhesion responses [26,27] and platelet adhesion and activation [28,29], and simulation studies of pillars embedded in a hydrogel matrix demonstrate a reduction in bacterial adhesion [30]. Since cells must compete with bacteria for surface space in many environments, the creation of bacteria-repellent surfaces using topography becomes highly important for tissue engineering. A large number of studies have been conducted to investigate how micron- and nano-scale topographies affect bacterial adhesion and biofilm formation, and to explore the possibility of promoting host tissue growth while inhibiting bacterial adhesion [26,27,31–33]. Although certain features were found to promote bacterial attachment and biofilm formation, the most successful features were identified as having an antifouling function. These features ranged from nano- to micro-scale, and could be either well-defined or have a relatively random size and distribution, they may have been designed or inspired from natural antifouling surfaces or been engineered as a novel surface structure, and may have been tested on polymeric and metallic materials, yet all these materials have in common that they can show significant control of bacterial adhesion and biofilm formation. Surface topographies can also show reductions in platelet adhesion and activation, providing a feasible approach for reducing the thrombogenicity of biomaterials [29,34]. Because this technique generates multifunctional surfaces, topographic modification has come to the fore in the area of antimicrobial and anti-biofouling materials [35–38], and is a promising approach to design implant surfaces for improved biocompatibility of medical devices [14,17,39].
To date, there is no material or technique that has proven ideal for controlling microbial infection or thrombosis on implanted medical devices. While surface topography modification has shown significant antimicrobial and antithrombotic effects and has been extensively studied, this use of a single approach still has its limitations. For example, the presence of small number of surface defects following fabrication of physical surface modification such as missed or collapsed structures may lead to local adhesion that can amplify leading to formation of a platelet mass or biofilm. Furthermore, the adsorption of biological components on topographic surfaces during long term exposure may lead to a loss in surface effectiveness, resulting in biofouling or thrombosis. A combination of two or more different strategies is a practical approach to achieve maximum biological performances of biomaterials, both in controlling microbial infections [40,41] and inhibiting potential for thrombotic events [42], by balancing the pros and cons of each of the strategies. The combination of surface topography modification with other techniques may overcome the shortcomings of each individual approach and provide potentially additive or even synergetic effects on increasing the antimicrobial and/or antithrombotic performances of biomaterials. In this review, we will discuss recent developments in surface texturing techniques and combinatorial approaches of surface techniques with other strategies such as grafting of poly (ethylene glycol) (PEG) and nitric oxide (NO) release to improve biocompatibility of implanted biomaterials.
2 Surface texturing
2.1 Basic theories of surface topography influences on bacterial adhesion responses to surface
In general, surface topography modification falls into two categories of patterns depending on the size of features, microscale and nanoscale, with these having different mechanisms in controlling bacterial adhesion and biofilm formation. Some of these surfaces were inspired from naturally existing antifouling surfaces such as plant leaves [43], shark skin [44], and insect wings [45]. Normally, microscale topographies do not have direct bactericidal effects, but may inhibit bacterial adhesion through influencing bacteria-material interactions, while some nanoscale topographies were created to inhibit biofilms by killing bacteria through mechanical forces [46]. Surface topography modification alters surface properties including roughness, surface energy (surface wettability), and hydrodynamic forces at the interface which influence bacterial adhesion, and by influencing initial bacterial adhesion they limit the subsequent biofilm formation. Bacteria can use a variety of mechanisms to colonize terrains with diverse surface topographies. Understanding how surface topography influences bacteria responses is essential for rational design of antifouling materials. Recent reviews on how bacterial cells respond to micro- and nano-scale topography provide a good summary of the most relevant work in this field [47,48].
Microscale patterns have been shown to influence bacterial adhesion behavior of different strains on various material surfaces including polymeric and metallic materials. Topographic features such as pattern size, shape, and distribution play an important role in bacterial attachment and biofilm formation [26]. It is a common observation that bacterial adhesion is reduced as the size of the pattern is smaller, especially when the size is reduced below a micron, as the bacterial cells are unable to access the underlying surface between features and the overall interaction area between cell and surface is reduced [49,50]. Bacterial cells can also actively sense the microscale surface topography and choose their position to settle during adhesion. There is evidence to show that cells can sense the conditions and will maximize contact area with the surfaces. This results in a specific alignment of the cells depending on the arrangement of the topographical details [31]. The edge areas of features are often the locations where bacteria prefer localization [50]. Bacterial structures such as flagella, type-IV pili, and envelope proteins [51–53] have been found important in sensing and responding the physical properties of a surface.
The nanostructure of insect wings has inspired many studies and designs for controlling bacterial adhesion and biofilm formation. The first example of a nanostructure that kills bacteria was reported on the surface of clanger cicada (Psaltoda claripennis) wings where Pseudomonas aeruginosa cells were found not to be repelled by the topography, but instead the nanopillar arrays present on the wing surface penetrated the bacteria, resulting in cell death [54]. Further studies showed that nanopillars on cicada wings effectively kill Gram-negative cells, but Gram-positive cells remain resistant due to their thick cell wall, suggesting that the cell mechanical properties, in particular cell rigidity, are important factors in determining bacterial resistance/sensitivity to the bactericidal nature of the wing surface [55,56]. More nano-topographic surfaces designed to mimic the nanostructure of insect wing were created on a variety materials and found to have strong bactericidal effects, e.g., vertically aligned carbon nanotubes [57], black silicon [58], and gold [59]. The discovery of insect wing nanostructures and the development of new biomimicking antibacterial nanostructured surfaces represents a new generation of biomaterials that can kill bacteria on contact based solely on physical surface structure [46].
Modification of surface topography can alter surface wettability, resulting in significant effect on bacterial adhesion. Two models, the Wenzel and Cassie–Baxter states, have been developed to describe wetting of a topographically modified surface. In Wenzel state, the surface topography enhances both hydrophobicity and hydrophilicity depending on the nature of the corresponding flat surface and always amplifies the intrinsic wettability of a surface, while in Cassie–Baxter state, air is entrapped within the features of the topography and the sessile water drop is supported by a composite surface of air and the substrate solid, resulting in increased hydrophobicity. The wetting state of surface topography apparently affects bacterial adhesion. An important example in nature is that the micro- or nano-structures on lotus and rice leaves make the surface superhydrophobic and exhibit excellent self-cleaning effects and non-contamination [60]. We created a series of submicron and micron sized textured pillar surfaces using polyurethane (PU) biomaterials, and then modified one set of these surfaces to be hydrophilic through glow discharge air plasma treatment. Results showed that the original textured hydrophobic surfaces showed significant reductions in adhesion of Stapholoccus epidermidis under shear as compared to smooth surfaces, regardless of the sizes of the patterns. However, bacterial adhesion on hydrophilic surfaces was dependent on the size of patterns. The submicron patterned surfaces showed reduced bacterial adhesion while the micron pattern surfaces were found to have increased bacterial adhesion. The data suggest that both the increased surface hydrophobicity and decreased availability of contact area contribute to a reduction in bacterial adhesion on hydrophobic textured surfaces, while in the case of hydrophilic textured surfaces, the availability of contact area is the primary determinant factor for bacterial adhesion [61].
The wettability of textured surfaces may also be transient and can change the adhesion at long time exposures. Friedlander et al. [51] reported that adhesion of Escherichia coli on polydimethylsiloxane (PDMS) surfaces having micron scale hexagonal features was significantly reduced during the first 2 h because of Cassie–Baxter state of the textured surface, but this behavior abruptly reversed to significantly increased adhesion at longer exposures. This is because flagella are able to reach into crevices, access additional surface area, and produce a dense, fibrous network. This facilitates the transition from Cassie–Baxter to Wenzel wetting state and changes the wettability of the surface, promoting biofilm formation, and suggesting that the future design of antifouling surfaces should consider the bacterial behavior in native, structured environments and also that the study of adhesion and biofilms for long term exposure is necessary.
2.2 Surface texturing with ordered arrays of submicron pillars
Pillars with square, round, or hexagonal shapes are the most common topographic features in the studies of surface topography modification for controlling bacterial biofilm formation. Other features include line patterns, irregular micro pits, honeycomb structures, cylindrical wells, and ridges. Fabrication of pillar shaped surface structures can be ordered or random. Random structures can be obtained by etching techniques, while order structures generally start from a silicon master pattern which is created by photolithography or electron beam lithography (EBL) technique. It is relatively easy to create structure at micron scale level of any desired shape using photolithography while EBL is a preferred tool of choices for writing submicron or nanoscale structure on a wide variety of materials. The minimum feature sizes of photolithography and EBL techniques are ∼500 nm and ∼6 nm, respectively [62]. A two-stage soft lithography replication process [63] can be used for transferring patterns from silicon master to polymeric biomaterial surfaces by working through a negative PDMS mold produced by casting against master patter. The polymer film with texture is then fabricated against the PDMS mold (Figure 1A). In our experience, a large advantage to the two-stage technique is that many replicates can be prepared from each silicone negative, while utilizing the wafer directly for fabrication often leads to damage when the first replicate is made making it prohibitively expensive. This process produces a very high yield of pillars, generally ∼99.8% on polymer film surfaces, indicating high efficiency in reproducing textured surfaces [64].
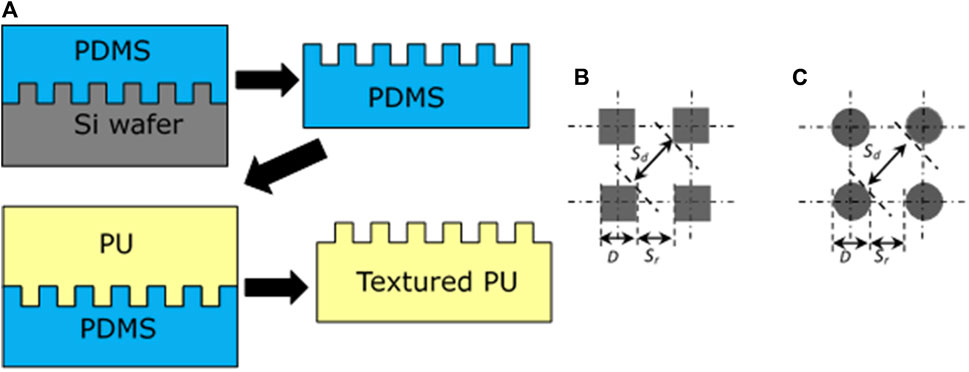
FIGURE 1. A two-stage soft lithography replication process for fabrication of textured polyurethane (PU) films (A), designs of square shape pillars (B) and round shape pillars (C) textured patterns. Reprinted from Xu and Siedlecki, Acta Biomaterialia, 2012. 8, 72–81.
Submicron textured surfaces have higher efficiency than micron size patterns in controlling bacterial adhesion [61,64]. While it is useful to study the design and application of submicron textured surfaces, the rationale for the design of such surface topography has been largely missing until a more recent study [65]. Generally, the dimensions of submicron pillars are desired to be smaller than the size of individual bacterial cells to limit the ability of the cells to access the space between pillars. Pillars reduce the surface contact area and thus reduce the opportunities for interactions between cells and surfaces. At the same time, the textured structure increases the surface hydrophobicity due to the trapped air in space between pillars. Both of these factors contribute to the interaction between cells and surfaces, resulting in the reduction of attachment and colonization of bacteria on surfaces. In a recent study, we created a series of textured surfaces with different pillar geometries (Table 1, Figures 1B,C) on polyurethane urea (PUU) biomaterial surfaces with two pillar shapes, square and round, and explored the Staphylococcal bacterial adhesion and biofilm formation on these submicron textured surfaces [65].
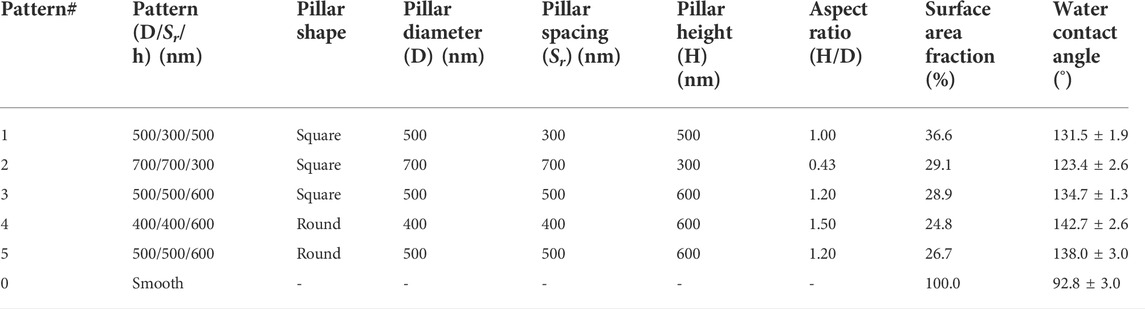
TABLE 1. Design parameters of textured pillars and the properties of textured surfaces on PUU substrate [65].
2.3 Inhibition of bacterial adhesion and biofilm formation by pillar textured surfaces
As discussed above, bacterial cells settle on the surfaces to maximize contact area to strengthen the binding with surfaces. Due to the limited access into pillar spaces, bacterial cells often adhere on the top surface areas of adjacent pillars individually or as 2-4 cell clusters on submicron textured surfaces (Figure 2) [65]. Normally, submicron textured surfaces lack the ability to kill bacteria, but in the case of small diameter pillars it appears the bacteria may experience pillar penetration through the cell. For example, cells were observed sitting on the top of pillars on Pattern #4 but the cell height appears less than pillar height, indicating that sharp polymeric pillars may have penetrated the cell and killed the bacteria (Figure 2D).
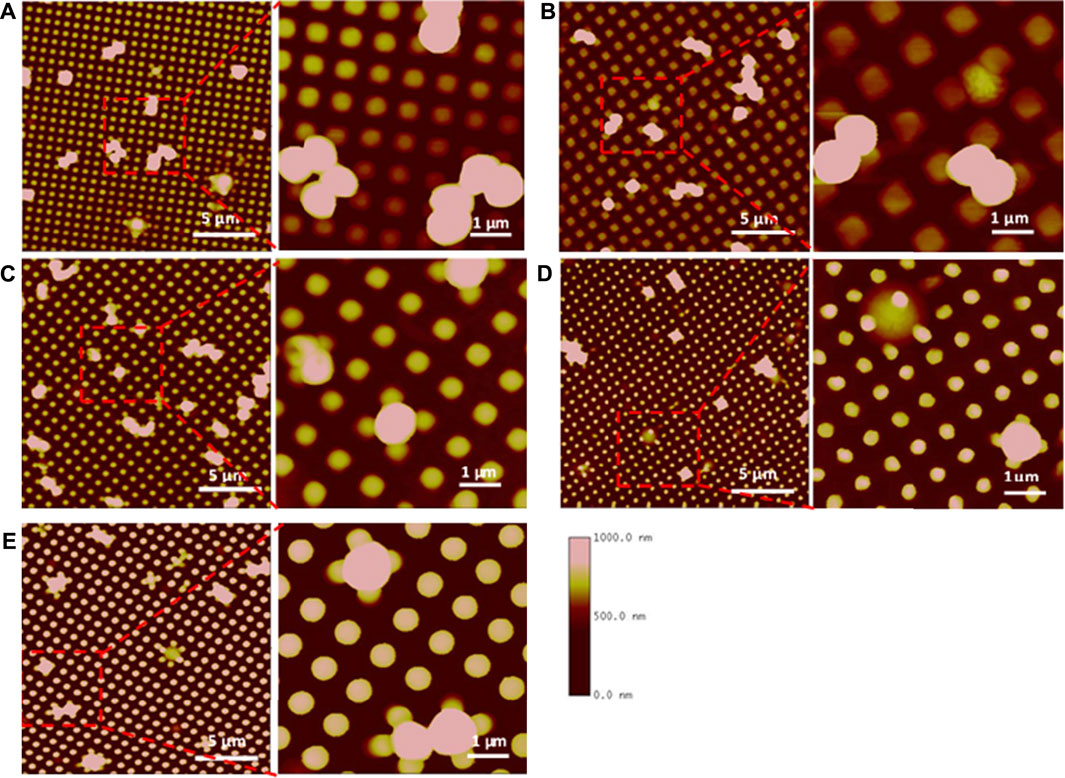
FIGURE 2. AFM images of S. aureus adhesion on textured surfaces. Square shaped pillars: Pattern 1, 500/300/500 (A), Pattern 2, 700/700/300 (B), Pattern 3, 500/500/600 (C). Round shaped pillars: Pattern 4, 400/400/600 (D), Pattern 5, 500/500/600 (E). Bacterial cells are captured in Pattern 3 or penetrated by the pillar in Pattern 4. Reprinted from Xu and Siedlecki, Journal of Biomedical Materials Research Part A, 2022.110, 1238–1250.
Surface contact area appears to be a more important factor in designing submicron textured surfaces compared to wettability [65]. Bacterial adhesion of both S. epidermidis RP62A and S. aureus Newman D2C increased linearly with surface contact area fraction (p < 0.05, Figure 3A), while the relationship between adhesion and wettability was not significant (p > 0.05, Figure 3B). These results suggest that the smaller the surface contact area, the lower the bacterial adhesion, but the degree of hydrophobicity has less effect on adhesion for submicron textured surfaces as long as the substrate is generally hydrophobic. The smaller surface contact area can be reached by reducing pillar size or increasing the spacing between pillars. However, decreasing the pillar size can cause adverse effects on the mechanical strength of the pillars, leading to pillar collapse or missing pillars following the replication process and this may lead to localized adhesion and biofilm formation after long exposure times. As a result, design of textured surfaces on polymeric biomaterials should consider ways to minimize the surface contact area such as reducing pillar size and increasing the spaces between pillars, while at the same time retaining pillar integrity to avoid defects in patterns resulting from either missing pillars or collapse of pillars from insufficient strength.
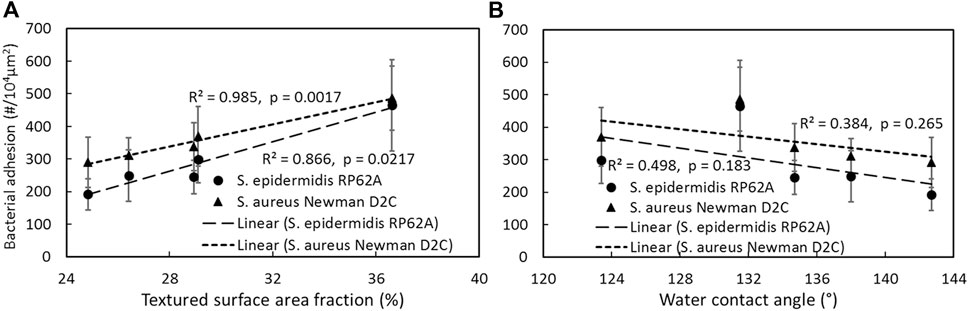
FIGURE 3. Correlation of bacterial adhesion with textured surface area fraction (A), textured surface water contact angle (B). Reprinted from Xu and Siedlecki, Journal of Biomedical Materials Research Part A, 2022.110, 1238–1250.
3 Combinatorial approach to improve biocompatibility of biomaterials
Utilizing a combination of two or more approaches in a biomaterial system is an important strategy for improving the biocompatibility of the biomaterials. Surface texturing with well-defined submicron textured surfaces inhibit bacterial adhesion and biofilm formation as well as platelet adhesion [34], however surface texturing is still far from ideal for controlling thrombosis and microbial infection. One of the reasons is that physical surface defects such as missed pillars during fabrication is always unavoidable, potentially leading to local adhesion and accumulation of platelets or bacteria. Considering the advantages of chemical modification, it is of interest to see how a combination of chemical and physical approaches will affect biological responses. Herein we discuss two combinatorial strategies of surface texturing with other chemical approaches including poly (ethylene glycol) (PEG) grafting and nitric oxide (NO) release to improve biocompatibility of implanted biomaterials.
3.1 Combination of surface texturing and poly (ethylene glycol) to improve the biocompatibility of biomaterials
Grafting polymer brushes to polymeric biomaterial substrates is an effective chemical surface modification approach to enhance biomaterial biocompatibility while maintaining the original bulk material performance [66]. Poly (ethylene glycol) (PEG) is widely used in the modification of biomaterials because of its high efficiency in resisting protein adsorption, weak immunogenicity, and good compatibility with living cells [67]. Both in vitro and in vivo experiments have shown PEG grafted surfaces have great potential for clinical applications in medical devices and implants [68]. Many studies have been reported that grafting PEG to PU biomaterial surfaces reduces protein adsorption [69], repels bacterial adhesion [70,71], and reduces platelet adhesion [72], thereby improving the biocompatibility. PU copolymers are among the primary materials used in a variety of blood-contacting medical devices due to their broad range of mechanical properties, fatigue resistance, and relatively good hemocompatibility, and as discussed above, PU copolymers are suitable for surface topography modification to improve the biological responses to materials. We studied combining chemical modification by grafting PEG onto physically modified (surface topography texturing) PU materials to yield a combinatorial surface with the expectation that biological responses to these surfaces will have significant reductions in platelet adhesion/activation and bacterial adhesion [73].
The method of grafting a polymer brush layer to inorganic or polymeric substrates depends on material surface chemical composition and properties [74]. Park et al. [70] reported a two-step treatment to graft PEG onto PDMS-based PUs. First -NCO groups were introduced onto the PU surface with from hexamethylene diisocyanate (HMDI) by allophanate reaction between urethane proton and isocyanate group in the presence of catalyst; subsequently the–NCO groups were reacted with PEG forming PEG-NCO-PU groups on the material. Liu et al. proposed a 3-step chemical treatment to graft high density PEG onto poly (ester-urethane) under mild-reaction conditions [75]. Similar to 2-step treatment, the film surface was first incorporated with -NCO groups on the surface by allophanate reaction; followed by reaction between the -NCO groups and NH2 groups in tris (2-aminoethyl) amine via condensation reaction to immobilize -NH2 on the surface; and finally, PEG was grafted on the polyurethane surface through Michael addition between terminal C=C bond of monoallyloxy PEG and -NH2 groups on the film surface. The material characterization shows the 3-step treated PEG surfaces have high grafting density and possess outstanding mechanical properties and resistance to protein adsorption and platelet adhesion [75]. To create a PEG-grafted-textured surface by combinatory approach, PU films were first physically textured with ordered arrays of pillars using a soft lithography two-stage replication molding technique as discussed prior. The textured PU films were then treated by two-step reactions with introduction of–NCO groups to surface from HMDI in the presence of triethylamine as a catalyst, then grafting the PEG onto PU surface [73].
PEG grafting generates a layer on the polymeric biomaterial and alters the surface chemistry and physiochemical surface properties. For example, surface wettability was changed from super hydrophobic to hydrophilic due the chemistry of PEG. PEG modification may also change the original surface topography during treatment. Due to the solvent involved in PEG modification, pillars with high aspect ratios may collapse and form a new pattern on surfaces. We found the submicron textured pillars with height of 600 nm collapsed (Figure 4A) while micron size pillars were intact after PEG grafting. The characterization of biological responses to surfaces including protein adsorption, platelet adhesion/activation, and bacterial adhesion/biofilm formation showed that PEG modification significantly reduced human fibrinogen (Fg) adsorption, suggesting PEG-grafted textured biomaterial surfaces were resistant to protein adsorption. Results also showed PEG-textured surfaces greatly increased the efficiency in reducing bacterial adhesion and platelet adhesion/activation due to the additive effects of physical topography and grafted PEG (Figures 4B,C), suggesting that a combination of chemical modification and surface texturing will be more efficient in preventing biomaterial-associated thrombosis and infection of biomaterials.
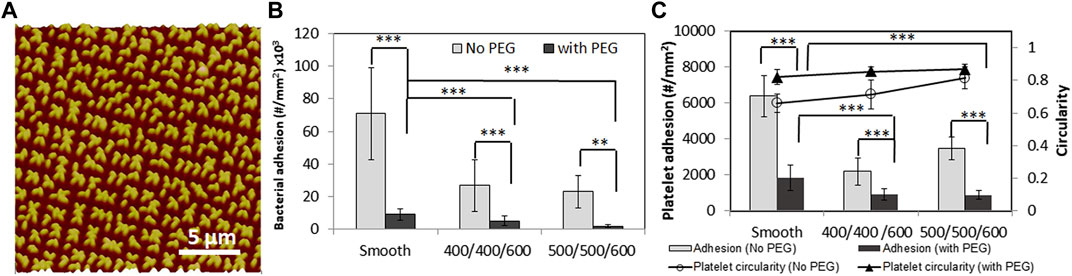
FIGURE 4. AFM topography image of PEG modified on textured PU surfaces with 500/500/600 nm pillar pattern (A). S. epidermidis RP62A adhesion (B) and platelet adhesion and activation (indicated by platelet circularity) (C) on smooth, textured with 400/400/600, 500/500/600 nm pillars, and PEG-textured PU surfaces in PBS for 1 h. **: p < 0.01, ***: p < 0.001. Reprinted from Xu and Siedlecki, Journal of Biomedical Materials Research Part B Applied Biomaterials 2017. 105, 668–678.
3.2 Combination of surface texturing and nitric oxide release
Nitric oxide (NO) releasing biomaterials represent another strategy towards multifunctional aspects in improving biocompatibility with potential for clinical use. NO is an endogenous gas molecule and its continuous release from the endothelial cells that line all blood vessels promotes various physiological functions in human body including enhancing endothelization, preventing the adhesion/activation of platelets, inhibiting bacterial proliferation and biofilm formation, signaling in the immune system response, and promoting angiogenesis and wound healing processes [76–81]. NO possesses broad-spectrum antibacterial activity against both Gram-positive and Gram-negative bacteria including methicillin-resistant Staphylococcus aureus (MRSA). The reactive species produced by NO, a diatomic free radical, can cross the membranes to enter the microbial cell readily and kill the microbe by degrading cell membranes, damaging DNA, denaturing proteins through production of potent nitrosating species or by combining with reactive oxygen species (e.g., superoxide, peroxide) and oxidizing the same targets [82,83]. NO can improve the activity of traditional antibiotics and increase bacterial susceptibility to multiple classes of antibiotics when used in combination antibiotics and it slows the development of antibiotic resistance [84]. For these reasons, polymeric materials that mimic endogenous NO release provide a potential solution against medical device-associated microbial infection and also can prevent thrombus formation [80,85–87]. However, the limits of lifetime, storage stability and costly synthesis of NO donors are challenges for the design of NO releasing materials, even though many NO donors have been synthesized and used for the goal of prolonged and controlled NO delivery [88,89]. Combinations of NO release with other antimicrobial and thromboresistant techniques such as surface topography modification might be an alternative approach to overcome their inherent drawbacks and enhance the biocompatibility of biomaterials.
Indeed, such combination of surface topography and NO release can be thought of as inspiration from a natural anticoagulative and antibacterial surface, the inner surface of a blood vessel. The inner surface of the aortic intima is not flat but rather possesses roughness over the scale of several microns, with micro-grooves in the blood flow direction and nano-protuberances on the ridges [90]. At the same time, endothelial cells on the blood vessel surface produce NO which contributes to anti-platelet activation/aggregation, anti-inflammation, and antibacterial properties of blood vessels in the cardiovascular system [91,92]. Motivated by this natural surface, we created a dual functional surface with a combination of surface texturing and NO release integrated on polyurethane biomaterials, where the NO donor, S-nitroso-N-acetylpenicillamine (SNAP) was incorporated in textured PU biomaterials consisting of ordered arrays of pillars on surfaces. Results showed this biomimetic modification of surface texturing and NO release provides an effective approach to improve the biocompatibility of polymeric materials in combating thrombosis and microbial infection [93–95].
NO donors can be incorporated into materials either by blending and physical dispersion within the polymeric matrix or by covalently binding them to either the polymer backbones and/or to the inorganic polymeric filler particles that are often employed to enhance the mechanics of biomedical polymers (e.g., fumed silica or titanium dioxide) [96]. Over the past 2 decades, researchers have focused on using N-diazeniumdiolates [97–99] and S-nitrosothiols [97,100,101] for controlled NO delivery due to their relatively high stability and the ability to release NO under physiological conditions. SNAP, a commonly used S-nitrosothiol, has been reported to be one of the most stable NO donors due to its intermolecular hydrogen bonding [102] and has been shown to be a very promising candidate in terms of antithrombosis and antimicrobial infection for fabrication of long term NO releasing biomedical polymers such as polyurethanes [103–105]. Incorporation of SNAP into PU biomaterials can be accomplished by either doping into the PU directly before fabrication of medical devices [103,106] or impregnation into PU after swelling in mild solvent following fabrication [107]. Under physiological conditions the former method was found to release NO for up to 3 weeks in low water uptake biomedical grade polymers such as Elast-eon E2As [103], while the later can achieve stable NO release for >4 weeks in silicon Foley urinary tract catheters [107].
Textured and NO releasing polyurethane biomaterials can be fabricated via two different processes based on the above-mentioned methods for incorporating SNAP. In a first process, SNAP was blended in PU solution with known concentration. Using soft lithography two-stage replication molding techniques (Figure 1A), a SNAP-free PU was first spin cast onto a textured silicone mold, then SNAP-doped PU was added on top of this first layer, and then finally, another layer of the regular PU was coated on SNAP-doped PU layer, resulting in SNAP-doped textured PU films composed of 3 layers with a base of PU, a middle layer containing SNAP, and a textured PU top layer with textured patterns (Figure 5A1) [94]. The thickness of each layer can be adjusted by additional spin coating of each material. Initially, the structure of the textured top thin layer appeared affected by diffusion of SNAP from the SNAP-doped middle layer, leading to some shallow pillars (Figure 5B1). To improve texturing, the increase in thickness of the top layer is necessary to limit the diffusion of SNAP into the textured layer during fabrication (Figure 5B2). In a second process, SNAP was dissolved in solvent of methanol (MeOH) and methyl ethyl ketone (MEK) (3:7 v/v). The textured PU films were first fabricated by soft lithography two-stage process and then soaked in SNAP-containing solutions and SNAP was impregnated into PU films by swelling the polymers (Figure 5A2) [93]. The loading amount of SNAP in impregnated PU films can be modified by adjusting the SNAP concentrations in MEK + MeOH solvent. It should be noted that the textured pillars may be affected by impregnation process and that pillars of small diameter and high aspect ratios, e.g., 500/500/600 nm pattern, may collapse and result in defects. Short pillars (e.g., 700/700/300 nm, Figure 5B3,4) or micron size pillars remain intact.
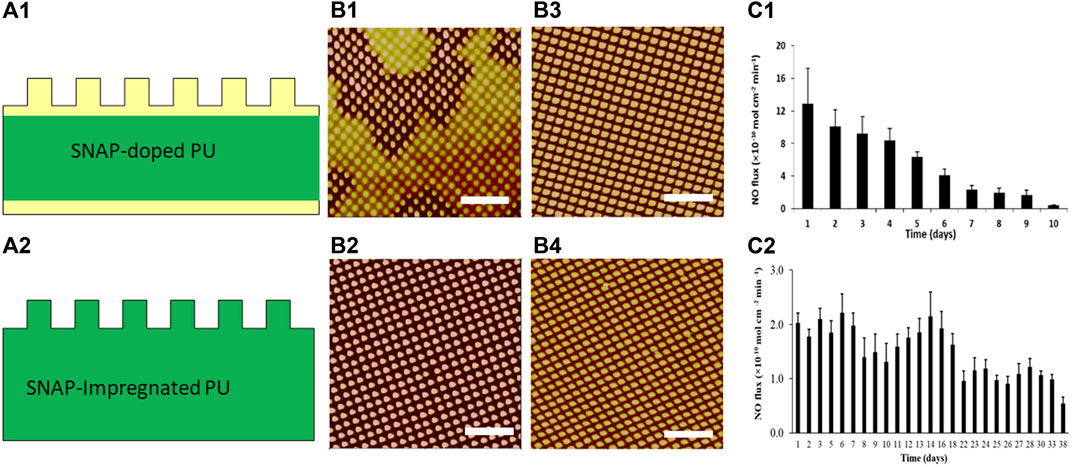
FIGURE 5. Schematic diagrams of textured and NO-releasing biomaterials by SNAP-doped (A1) and SNAP-impregnated (A2) PU films. Yellow color represents regular PU and green color represents SNAP containing PU. AFM topographic images of thin SNAP-doped PU (B1) and thick SNAP-doped PU (B2) with pattern 500/500/600 nm, textured PUs with pattern 700/700/300 nm before (B3) and after (B4) impregnated with SNAP; NO release from SNAP-doped PU (C1) and SNAP-impregnated PU (C2). Scale bar = 5 µm. Reprinted from Xu LC et al. Acta Biomaterialia, 2017. 51: p. 53–65 and Wo Y et al. Biomaterials Science, 2017. 5(7): p. 1265–1278.
NO release from SNAP textured PU films depends on the SNAP incorporation method and textured patterns. S-nitrosothiol can decompose into disulfides and release NO under light, heat or in the presence of metal ions. The NO release flux rate depends on the topcoat polymer layer thickness, the surface topography, and the content of S-nitrosothiol in the SNAP-doped polymer films. NO flux was generally higher on the textured surface than on the normal smooth surface, likely due to the increased surface area of the textured film [94]. NO flux >0.5 × 10–10 mol min−1 cm−2 released from 15% SNAP-doped textured with 500/500/600 nm patterns lasts up to 10 days (Figure 5C1). However, the SNAP-impregnated polymers release NO much more slowly and stably, with release out to 38 days (Figure 5C2). The short lifetime of NO release in SNAP-doped textured films is believed due to issues with recrystallization and degradation of the SNAP during the repeated layers spun coat during fabrication.
Textured and NO releasing biomaterials surfaces have shown synergistic and additive effects on inhibition of bacterial adhesion and biofilm formation. Under near static condition, surface texturing alone reduced S. epidermidis bacterial adhesion by ∼ 61%–64% compared to smooth samples, while SNAP-doped textured polymer films increased the reduction rate to 88% for textured films with 500/500/600 nm patterns and loaded with 15 wt% SNAP. Longer term experiments to observe biofilm formation demonstrate that the SNAP doped-textured PU surface inhibit biofilm formation for >28 days [94]. Additional experiments on SNAP-impregnated textured PU surfaces show the combined benefits of NO release and texturing on adhesion of 4 representative hospital infection strains (S. epidermidis, S. aureus, P. aeruginosa, and E. coli), where both additive and in some cases even synergistic effects were seen, with reductions in bacterial adhesion greater than the sum of the individual reductions. NO release also kills bacteria on surfaces; viability test shows reduction rates up to 97% on SNAP impregnated textured PU surfaces [93].
Surface topographic features at the submicrometer level show significant reductions in platelet response compared to smooth surfaces [34]. In a study of platelet adhesion to textured and NO releasing surfaces in plasma, results demonstrated that the textured film without SNAP significantly reduced platelet adhesion with a reduction of 58% compared to the smooth unloaded polyurethane biomaterials (p < 0.001) in plasma. The NO releasing surfaces also significantly reduced the platelet adhesion with a reduction rate of approximately 76%. The combination of surface texturing and NO release sinergistically worked to reduce platelet adhesion by ∼89%. An in vitro plasma coagulation assay showed that this combined surface significantly increased the plasma coagulation time and reduced coagulation factor XII (FXII) activation, thereby reducing the risk of blood coagulation and thrombosis [95]. All these results demonstrate that a biomimetic modification with combination of surface texturing and NO release provides an effective approach to improve the biocompatibility of polymeric materials in combating thrombosis and microbial infection.
4 Mechanistic study of bacterial adhesion and biofilm formation on combined surface texturing and NO releasing surfaces
While surface texturing and combination with other antimicrobial and anti-thrombogentic approaches have been widely studied, mechanistic studies of biological responses to a variety of surfaces are still limited and molecular mechanisms behind them are poorly understood. Surface texturing reduces the surface contact area for interactions of bacterial cells and surface, and appears to interrupt both bacterial attachment and biofilm formation. In contrast to bacterial adhesion on regular smooth surfaces where cells are likely to adhere with clusters and form biofilms (Figure 6A), individual cells or small clusters of 2-4 cells are often observed on textured surfaces, and these bacteria/small aggregates seem to settle into the areas between pillars to maximize contact area. When cells cannot access completely into the spaces between pillars, for example with S. epidermidis adhesion on submicron textured surfaces (Figure 2), the individual cells can be embedded or trapped, and line in the spaces between pillars if the spaces are large enough for the access of cell. An example of this is seen in Figure 6C showing P. aeruginosa adhesion on 700/700/300 nm patterned surfaces. In the case of NO releasing biomaterials, individual cells or small clusters with 2-4 cells were found adhered on smooth surfaces (Figure 6B), and generally only individual cells were attached to the top of pillars on the textured-NO releasing surfaces instead of accessing into the spaces although they have same geometry (Figure 6C vs Figure 6D), suggesting NO may serve signals to further inhibit bacterial interaction with surface during attachment.
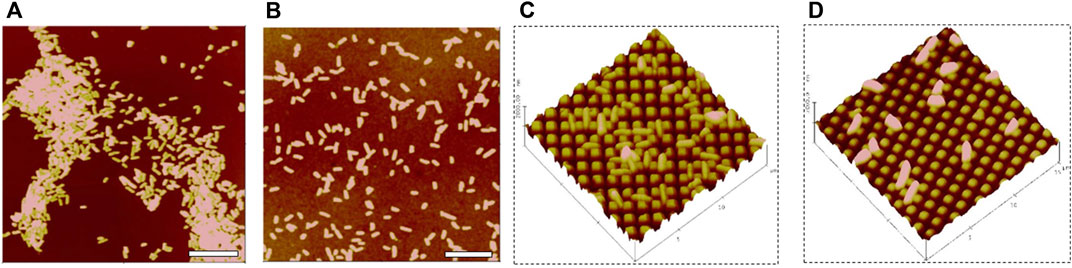
FIGURE 6. P. aeruginosa adhesion on smooth (A), smooth with 15% SNAP (B), side view bacterial adhesions on 700/700/300 nm patterned without SNAP (C), and 700/700/300 nm patterned with 10% SNAP PU films (D). Scale bar = 10 µm. Side-view (C) and (D) scan area: 15 × 15 µ2. Reprinted from Wo Y et al. Biomaterials Science, 2017. 5(7): p. 1265–1278.
Bacteria can use multiple signaling pathways to monitor and respond appropriately to changing environments. When transitioning from planktonic cells to the sessile biofilm community, bacterial cells undergo a series of physiological, metabolic, and phenotypic changes during the course of biofilm development via small molecule chemical signals [108]. Extracellular quorum sensing (QS) and intracellular nucleotide second messengers such as cyclic dimeric guanosine monophosphate (c-di-GMP) signaling, are two important chemical signaling systems that control the transition during biofilm formation. QS allows bacteria to sense the population density and species composition of the surrounding bacterial consortium through secretion and detection of chemical signals called autoinducers [109], while c-di-GMP is an universal intracellular secondary messenger which controls the biofilm life cycle in many bacterial species [110–114]. Although much is known about QS control of biofilms, the molecular mechanism by which c-di-GMP controls biofilm formation on the topography modified and NO releasing surfaces is less well understood.
C-di-GMP is a soluble molecule and functions as second messenger in bacterial cells attachment. It is synthesized from two molecules of GTP (Guanosine-5′-triphosphate) by di-guanylate cyclases (DGCs) and is degraded into pGpG (5′-Phosphoguanylyl-(3′,5′)-guanosine) and GMP by phosphodiesterases (PDEs) [115,116]. DGCs and PDEs respond to a broad range of environmental cues and modulate intracellular levels of c-di-GMP, which regulates various cellular functions including biofilm formation, virulence, and dispersal in many bacterial species [117–120]. It is commonly accepted that high intracellular c-di-GMP content enhances biofilm formation by reducing motility and inducing production of biofilm matrix while low c-di-GMP content in biofilm cells leads to increased motility and biofilm dispersal for many bacterial species [113,121,122]. In contrast to common observation as a positive regulator of the sessile state however, it was also observed that reducing c-di-GMP level can increase the attachment of bacteria for some species. For example, Suchanek et al. observed that the attachment of E coli was enhanced by deletion of the c-di-GMP-dependent flagellar brake YcgR or the diguanylate cyclase DgcE, due to the increased swimming speed of E. coli in absence of YcgR-mediated motor control [114]. More studies demonstrate that bacterial cells can sense the environments such as temperature [123], shear [124] and even material surface stiffness [125] to regulate biofilm formation via c-di-GMP signaling. NO was reported as a key mediator of biofilm dispersal that is conserved across microbial species [126,127]; and it induces P. aeruginosa biofilm dispersal by enhancing the activity of phosphodiesterase, resulting in the degradation of c-di-GMP [128]. However, the study of NO release and surface texturing on c-di-GMP signaling is still limited. In addition, microorganisms may also use other cyclic nucleotide second messengers to regulate adhesion and biofilm formations such as cyclic adenosine-monophosphate (cAMP), cyclic guanosine -monophosphate (cGMP), and cyclic-di-AMP [129]. It seems likely that generating increasingly detailed analysis of nucleotide second messengers in cells will reveal molecular mechanisms of bacterial cells in response to the biomaterial surfaces with surface texturing and/or NO release or other modified surfaces.
5 Overall perspective and conclusion
Surface topography modification is a promising approach to combat biomaterial associated microbial infection and blood thrombosis in order to improve the biocompatibility of biomaterials for the implantation of blood-contacting medical devices, all done without altering the bulk material properties and without the use of antibiotics. Surface texturing with submicron pillars reduces the surface contact area and changes the physicochemical properties at surfaces, thereby influencing the interaction between bacterial cells and surfaces and inhibiting initial attachment. Design of pillars should consider ways to minimize the surface contact area for the interaction of cells with surfaces, while retaining pillar integrity and minimizing the defects in patterns such as missing or collapsed pillars from insufficient strength should be avoided.
Surface texturing can be combined with other antibacterial or antithrombosis approaches to further improve the biocompatibility (Figure 7). Surface texturing with pillars interrupts the bacterial attachment and secretion of EPS for the biofilm matrix, inhibiting biofilms formed on surfaces. The combination of surface texturing and chemical modification with PEG increases the surface resistance to protein adsorption, platelet adhesion/activation, and bacterial adhesion, and increases the efficiency in preventing biomaterial-associated thrombosis and infection of biomaterials compared to each approach singularly. Similarly, biomimetic combinations of surface texturing and NO release also provides an effective approach to improve the biocompatibility of polymeric materials in combating thrombosis and microbial infection.
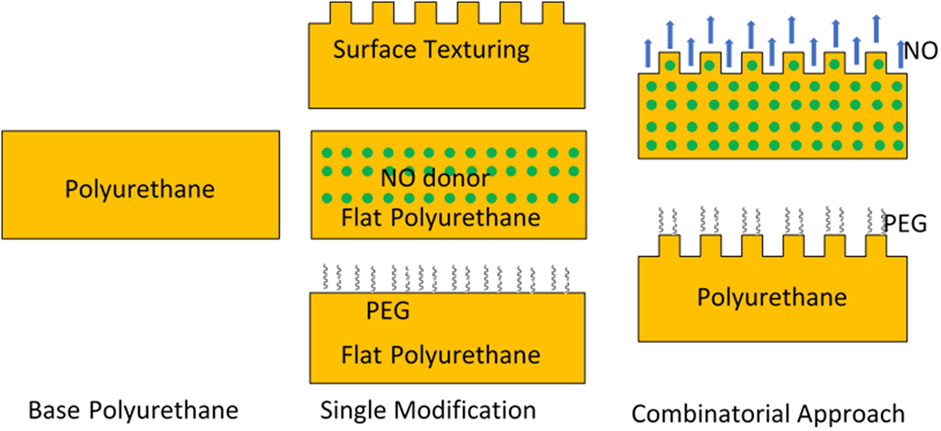
FIGURE 7. Scheme of single modification and combinatorial approaches to improve biocompatibility of materials.
While surface texturing and combinatorial approaches to minimize bacterial adhesion and biofilm formation have been elucidated in literature, the mechanistic studies of biological responses to the modified surfaces are still limited and molecular mechanisms behind the phenomena are less well understood. Quorum sensing and c-di-GMP signaling are two important chemical signaling systems that control bacterial adhesion and biofilm formation. Small-molecule chemical signals in these two systems convey information about the presence, nature, number, and characteristics of the surrounding bacterial species as well as the composition of the environment. The systematic analysis of these small molecules such as a combination study of QS and c-di-GMP would be beneficial to understand the molecular mechanisms of bacterial responses to biomaterial surfaces with different modifications, with the new knowledge obtained providing rational for further improvements in the design of biomaterial surfaces and development of synthetic materials intended to be used in implant applications.
Author contributions
CS wrote sections of the manuscript, edited the manuscript and submitted the manuscript. L-CX wrote sections of the manuscript and edited the manuscript.
Funding
The work is financially supported from NIH R01 HL153231 and R21 AI139706
Conflict of interest
The authors declare that the research was conducted in the absence of any commercial or financial relationships that could be construed as a potential conflict of interest.
Publisher’s note
All claims expressed in this article are solely those of the authors and do not necessarily represent those of their affiliated organizations, or those of the publisher, the editors and the reviewers. Any product that may be evaluated in this article, or claim that may be made by its manufacturer, is not guaranteed or endorsed by the publisher.
References
1. Rose EA, Gelijns AC, Moskowitz AJ, Heitjan DF, Stevenson LW, Dembitsky W, et al. Randomized Evaluation MechLong-term use of a left ventricular assist device for end-stage heart failure. N Engl J Med Overseas Ed (2001) 345:1435–43. doi:10.1056/nejmoa012175
2. Jaffer IH, Fredenburgh JC, Hirsh J, Weitz JI. Medical device-induced thrombosis: What causes it and how can we prevent it? J Thromb Haemost (2015) 13:S72–S81. doi:10.1111/jth.12961
3. Susen S, Rauch A, Van Belle E, Vincentelli A, Lenting PJ. Circulatory support devices: Fundamental aspects and clinical management of bleeding and thrombosis. J Thromb Haemost (2015) 13:1757–67. doi:10.1111/jth.13120
4. Pereda D, Conte JV. Left ventricular assist device driveline infections. Cardiol Clin (2011) 29:515–27. doi:10.1016/j.ccl.2011.08.004
5. Vanepps JS, Younger JG. Implantable device-related infection. Shock (2016) 46:597–608. doi:10.1097/shk.0000000000000692
6. Gorbet MB, Sefton MVMV. Biomaterial-associated thrombosis: Roles of coagulation factors, complement, platelets and leukocytes. Biomaterials (2004) 25:5681–703. doi:10.1016/j.biomaterials.2004.01.023
7. Rochford ETJ, Richards RG, Moriarty TF. Influence of material on the development of device-associated infections. Clin Microbiol Infect (2012) 18:1162–7. doi:10.1111/j.1469-0691.2012.04002.x
8. Labarrere CA, Dabiri AE, Kassab GS. Thrombogenic and inflammatory reactions to biomaterials in medical devices. Front Bioeng Biotechnol (2020) 8:123. doi:10.3389/fbioe.2020.00123
9. Yaseen M, Pan E, Zhao X, Lu J. Surface modification to improve biocompatibility. Compr Biotechnol (2011) 5:65–81. doi:10.1016/b978-0-08-088504-9.00178-1
10. Vogler EA. Chapter 8 - surface modification for biocompatibility. In: A Lakhtakia, and RJ Martín-Palma, editors. Engineered biomimicry. Boston: Elsevier (2013). p. 189–220.
11. Balakrishnan B, Kumar DS, Yoshida Y, Jayakrishnan A. Chemical modification of poly(vinyl chloride) resin using poly(ethylene glycol) to improve blood compatibility. Biomaterials (2005) 26:3495–502. doi:10.1016/j.biomaterials.2004.09.032
12. Monika SK, Das S, Ranjan A, Singh SK, Roy P, Misra N, et al. Chemical modification of poly (vinyl chloride) for blood and cellular biocompatibility. RSC Adv (2015) 5:45231–8. doi:10.1039/c5ra03362d
13. Variola F, Vetrone F, Richert L, Jedrzejowski P, Yi J-H, Zalzal S, et al. Improving biocompatibility of implantable metals by nanoscale modification of surfaces: An overview of strategies, fabrication methods, and challenges. Small (2009) 5:996–1006. doi:10.1002/smll.200801186
14. Harvey AG, Hill EW, Bayat A. Designing implant surface topography for improved biocompatibility. Expert Rev Med Devices (2013) 10:257–67. doi:10.1586/erd.12.82
15. Song J, Winkeljann B, Lieleg O. Biopolymer-based coatings: Promising strategies to improve the biocompatibility and functionality of materials used in biomedical engineering. Adv Mater Inter (2020) 7:2000850. doi:10.1002/admi.202000850
16. Mitra D, Kang E-T, Neoh KG. Polymer-based coatings with integrated antifouling and bactericidal properties for targeted biomedical applications. ACS Appl Polym Mater (2021) 3:2233–63. doi:10.1021/acsapm.1c00125
17. Bose S, Robertson SF, Bandyopadhyay A. Surface modification of biomaterials and biomedical devices using additive manufacturing. Acta Biomater (2018) 66:6–22. doi:10.1016/j.actbio.2017.11.003
18. Harawaza K, Cousins B, Roach P, Fernandez A. Modification of the surface nanotopography of implant devices: A translational perspective. Mater Today Bio (2021) 12:100152. doi:10.1016/j.mtbio.2021.100152
19. Lord MS, Foss M, Besenbacher F. Influence of nanoscale surface topography on protein adsorption and cellular response. Nano Today (2010) 5:66–78. doi:10.1016/j.nantod.2010.01.001
20. Hasan A, Saxena V, Pandey LM. Surface functionalization of Ti6Al4V via self-assembled monolayers for improved protein adsorption and fibroblast adhesion. Langmuir (2018) 34:3494–506. doi:10.1021/acs.langmuir.7b03152
21. Hansen JC, Lim JY, Xu L-C, Siedlecki CA, Mauger DT, Donahue HJ. Effect of surface nanoscale topography on elastic modulus of individual osteoblastic cells as determined by atomic force microscopy. J Biomech (2007) 40:2865–71. doi:10.1016/j.jbiomech.2007.03.018
22. Anselme K, Davidson P, Popa AM, Giazzon M, Liley M, Ploux L. The interaction of cells and bacteria with surfaces structured at the nanometre scale. Acta Biomater (2010) 6:3824–46. doi:10.1016/j.actbio.2010.04.001
23. Estévez M, Martínez E, Yarwood SJ, Dalby MJ, Samitier J. Adhesion and migration of cells responding to microtopography. J Biomed Mater Res A (2014) 103:1659–68. doi:10.1002/jbm.a.35293
24. Jeon H, Simon CG, Kim G. A mini-review: Cell response to microscale, nanoscale, and hierarchical patterning of surface structure. J Biomed Mater Res (2014) 102:1580–94. doi:10.1002/jbm.b.33158
25. Sousa MP, Arab-Tehrany E, Cleymand F, Mano JF. Surface micro- and nanoengineering: Applications of layer-by-layer technology as a versatile tool to control cellular behavior. Small (2019) 15:1901228. doi:10.1002/smll.201901228
26. Perera-Costa D, Bruque JM, González-Martín ML, Gómez-García AC, Vadillo-Rodríguez V. Studying the influence of surface topography on bacterial adhesion using spatially organized microtopographic surface patterns. Langmuir (2014) 30:4633–41. doi:10.1021/la5001057
27. Chang Y-R, Weeks ER, Ducker WA. Surface topography hinders bacterial surface motility. ACS Appl Mater Inter (2018) 10:9225–34. doi:10.1021/acsami.7b16715
28. Park JY, Gemmell CH, Davies JE. Platelet interactions with titanium: Modulation of platelet activity by surface topography. Biomaterials (2001) 22:2671–82. doi:10.1016/s0142-9612(01)00009-6
29. Koh LB, Rodriguez I, Venkatraman SS. The effect of topography of polymer surfaces on platelet adhesion. Biomaterials (2010) 31:1533–45. doi:10.1016/j.biomaterials.2009.11.022
30. Liu Y, Balazs AC. Modeling biofilm formation on dynamically reconfigurable composite surfaces. Langmuir (2018) 34:1807–16. doi:10.1021/acs.langmuir.7b03765
31. Hsu LC, Fang J, Borca-Tasciuc DA, Worobo RW, Moraru CI. Effect of micro- and nanoscale topography on the adhesion of bacterial cells to solid surfaces. Appl Environ Microbiol (2013) 79:2703–12. doi:10.1128/aem.03436-12
32. Liu L, Ercan B, Sun L, Ziemer KS, Webster TJ. Understanding the role of polymer surface nanoscale topography on inhibiting bacteria adhesion and growth. ACS Biomater Sci Eng (2016) 2:122–30. doi:10.1021/acsbiomaterials.5b00431
33. Hasan J, Jain S, Padmarajan R, Purighalla S, Sambandamurthy VK, Chatterjee K. Multi-scale surface topography to minimize adherence and viability of nosocomial drug-resistant bacteria. Mater Des (2018) 140:332–44. doi:10.1016/j.matdes.2017.11.074
34. Milner KR, Snyder AJ, Siedlecki CA. Sub-micron texturing for reducing platelet adhesion to polyurethane biomaterials. J Biomed Mater Res A (2006) 76:561–70. doi:10.1002/jbm.a.30554
35. Graham M, Cady N. Nano and microscale topographies for the prevention of bacterial surface fouling. Coatings (2014) 4:37–59. doi:10.3390/coatings4010037
36. Rigo S, Cai C, Gunkel-Grabole G, Maurizi L, Zhang X, Xu J, et al. Nanoscience-based strategies to engineer antimicrobial surfaces. Adv Sci (Weinh) (2018) 5:1700892. doi:10.1002/advs.201700892
37. Echeverria C, Torres MDT, Fernández-García M, De La Fuente-Nunez C, Muñoz-Bonilla A. Physical methods for controlling bacterial colonization on polymer surfaces. Biotechnol Adv (2020) 43:107586. doi:10.1016/j.biotechadv.2020.107586
38. Yang K, Shi J, Wang L, Chen Y, Liang C, Yang L, et al. Bacterial anti-adhesion surface design: Surface patterning, roughness and wettability: A review. J Mater Sci Techn (2021) 99:82–100. doi:10.1016/j.jmst.2021.05.028
39. Demirci S, Dikici T, Güllüoğlu AN. Micro/nanoscale surface modification of Ti6Al4V alloy for implant applications. J Mater Eng Perform (2022) 31:1503–11. doi:10.1007/s11665-021-06232-y
40. Song B, Zhang E, Han X, Zhu H, Shi Y, Cao Z. Engineering and application perspectives on designing an antimicrobial surface. ACS Appl Mater Inter (2020) 12:21330–41. doi:10.1021/acsami.9b19992
41. Zou Y, Zhang Y, Yu Q, Chen H. Dual-function antibacterial surfaces to resist and kill bacteria: Painting a picture with two brushes simultaneously. J Mater Sci Techn (2021) 70:24–38. doi:10.1016/j.jmst.2020.07.028
42. Ashcraft M, Douglass M, Chen Y, Handa H. Combination strategies for antithrombotic biomaterials: An emerging trend towards hemocompatibility. Biomater Sci (2021) 9:2413–23. doi:10.1039/d0bm02154g
43. Fu J, Zhang H, Guo Z, Feng D-Q, Thiyagarajan V, Yao H. Combat biofouling with microscopic ridge-like surface morphology: A bioinspired study. J R Soc Interf (2018) 15:20170823. doi:10.1098/rsif.2017.0823
44. Chung KK, Schumacher JF, Sampson EM, Burne RA, Antonelli PJ, Brennan AB. Impact of engineered surface microtopography on biofilm formation of Staphylococcus aureus. Biointerphases (2007) 2:89–94. doi:10.1116/1.2751405
45. Ivanova EP, Nguyen SH, Guo Y, Baulin VA, Webb HK, Truong VK, et al. Bactericidal activity of self-assembled palmitic and stearic fatty acid crystals on highly ordered pyrolytic graphite. Acta Biomater (2017) 59:148–57. doi:10.1016/j.actbio.2017.07.004
46. Linklater DP, Baulin VA, Juodkazis S, Crawford RJ, Stoodley P, Ivanova EP. Mechano-bactericidal actions of nanostructured surfaces. Nat Rev Microbiol (2021) 19:8–22. doi:10.1038/s41579-020-0414-z
47. Cheng Y, Feng G, Moraru CI. Micro- and nanotopography sensitive bacterial attachment mechanisms: A review. Front Microbiol (2019) 10:191. doi:10.3389/fmicb.2019.00191
48. Lee SW, Phillips KS, Gu H, Kazemzadeh-Narbat M, Ren D. How microbes read the map: Effects of implant topography on bacterial adhesion and biofilm formation. Biomaterials (2021) 268:120595. doi:10.1016/j.biomaterials.2020.120595
49. Ge X, Leng Y, Lu X, Ren F, Wang K, Ding Y, et al. Bacterial responses to periodic micropillar array. J Biomed Mater Res A (2015) 103:384–96. doi:10.1002/jbm.a.35182
50. Wang L, Chen W, Terentjev E. Effect of micro-patterning on bacterial adhesion on polyethylene terephthalate surface. J Biomater Appl (2015) 29:1351–62. doi:10.1177/0885328214563998
51. Friedlander RS, Vlamakis H, Kim P, Khan M, Kolter R, Aizenberg J. Bacterial flagella explore microscale hummocks and hollows to increase adhesion. Proc Natl Acad Sci U S A (2013) 110:5624–9. doi:10.1073/pnas.1219662110
52. Gu H, Chen A, Song X, Brasch ME, Henderson JH, Ren D. How Escherichia coli lands and forms cell clusters on a surface: A new role of surface topography. Sci Rep (2016) 6:29516. doi:10.1038/srep29516
53. Gordon VD, Wang L. Bacterial mechanosensing: The force will be with you, always. J Cel Sci (2019) 132:jcs227694. doi:10.1242/jcs.227694
54. Ivanova EP, Hasan J, Webb HK, Truong VK, Watson GS, Watson JA, et al. Natural bactericidal surfaces: Mechanical rupture of Pseudomonas aeruginosa cells by cicada wings. Small (2012) 8:2489–94. doi:10.1002/smll.201200528
55. Hasan J, Webb HK, Truong VK, Pogodin S, Baulin VA, Watson GS, et al. Selective bactericidal activity of nanopatterned superhydrophobic cicada Psaltoda claripennis wing surfaces. Appl Microbiol Biotechnol (2013) 97:9257–62. doi:10.1007/s00253-012-4628-5
56. Pogodin S, Hasan J, Baulin VA, Webb HK, Truong VK, Phong nguyen TH, et al. Biophysical model of bacterial cell interactions with nanopatterned cicada wing surfaces. Biophysical J (2013) 104:835–40. doi:10.1016/j.bpj.2012.12.046
57. Linklater DP, De Volder M, Baulin VA, Werner M, Jessl S, Golozar M, et al. High aspect ratio nanostructures kill bacteria via storage and release of mechanical energy. Acs Nano (2018) 12:6657–67. doi:10.1021/acsnano.8b01665
58. Ivanova EP, Hasan J, Webb HK, Gervinskas G, Juodkazis S, Truong VK, et al. Bactericidal activity of black silicon. Nat Commun (2013) 4:2838. doi:10.1038/ncomms3838
59. Wu S, Zuber F, Brugger J, Maniura-Weber K, Ren Q. Antibacterial Au nanostructured surfaces. Nanoscale (2016) 8:2620–5. doi:10.1039/c5nr06157a
60. Feng L, Li S, Li Y, Li H, Zhang L, Zhai J, et al. Super-hydrophobic surfaces: From natural to artificial. Adv Mater (2002) 14:1857–60. doi:10.1002/adma.200290020
61. Xu L-C, Siedlecki CA. Staphylococcus epidermidis adhesion on hydrophobic and hydrophilic textured biomaterial surfaces. Biomed Mater (2014) 9:035003. doi:10.1088/1748-6041/9/3/035003
62. Bogalecki A, Du Plessis M. Design and manufacture of quantum-confined SI light sources. SAIEE Afr Res J (2010) 101:11–6. doi:10.23919/saiee.2010.8532214
63. Xia Y, Whitesides GM. Soft lithography. Annu Rev Mater Sci (1998) 28:153–84. doi:10.1146/annurev.matsci.28.1.153
64. Xu L-C, Siedlecki CA. Submicron-textured biomaterial surface reduces staphylococcal bacterial adhesion and biofilm formation. Acta Biomater (2012) 8:72–81. doi:10.1016/j.actbio.2011.08.009
65. Xu L-C, Siedlecki CA. Submicron topography design for controlling staphylococcal bacterial adhesion and biofilm formation. J Biomed Mater Res A (2022) 110:1238–50. doi:10.1002/jbm.a.37369
66. Sun W, Liu W, Wu Z, Chen H. Chemical surface modification of polymeric biomaterials for biomedical applications. Macromol Rapid Commun (2020) 41:1900430. doi:10.1002/marc.201900430
67. Alibeik S, Zhu S, Brash JL. Surface modification with PEG and hirudin for protein resistance and thrombin neutralization in blood contact. Colloids Surf B: Biointerfaces (2010) 81:389–96. doi:10.1016/j.colsurfb.2010.07.024
68. Nagaoka S, Nakao A. Clinical application of antithrombogenic hydrogel with long poly (ethylene oxide) chains. Biomaterials (1990) 11:119–21. doi:10.1016/0142-9612(90)90126-b
69. Chen H, Zhang Y, Li D, Hu X, Wang L, Mcclung WG, et al. Surfaces having dual fibrinolytic and protein resistant properties by immobilization of lysine on polyurethane through a PEG spacer. J Biomed Mater Res A (2009) 90A:940–6. doi:10.1002/jbm.a.32152
70. Park KD, Kim YS, Han DK, Kim YH, Lee EHB, Suh H, et al. Bacterial adhesion on PEG modified polyurethane surfaces. Biomaterials (1998) 19:851–9. doi:10.1016/s0142-9612(97)00245-7
71. Park JH, Lee KB, Kwon IC, Bae YH. PDMS-based polyurethanes with MPEG grafts: Mechanical properties, bacterial repellency, and release behavior of rifampicin. J Biomater Sci Polym Edition (2001) 12:629–45. doi:10.1163/156856201316883458
72. Park JH, Park KD, Bae YH. PDMS-Based polyurethanes with MPEG grafts: Synthesis, characterization and platelet adhesion study. Biomaterials (1999) 20:943–53. doi:10.1016/s0142-9612(98)00250-6
73. Xu LC, Siedlecki CA. Protein adsorption, platelet adhesion, and bacterial adhesion to polyethylene‐glycol‐textured polyurethane biomaterial surfaces. J Biomed Mater Res (2017) 105:668–78. doi:10.1002/jbm.b.33592
74. Zdyrko B, Luzinov I. Polymer brushes by the “grafting to” method. Macromol Rapid Commun (2011) 32:859–69. doi:10.1002/marc.201100162
75. Liu L, Gao Y, Zhao J, Yuan L, Li C, Liu Z, et al. A mild method for surface-grafting PEG onto segmented poly(ester-urethane) film with high grafting density for biomedical purpose. Polymers (2018) 10:1125. doi:10.3390/polym10101125
76. Radomski MW, Palmer RMJ, Moncada S. The role of nitric oxide and cGMP in platelet adhesion to vascular endothelium. Biochem Biophysical Res Commun (1987) 148:1482–9. doi:10.1016/s0006-291x(87)80299-1
77. Bogdan C. Nitric oxide and the immune response. Nat Immunol (2001) 2:907–16. doi:10.1038/ni1001-907
78. Fang FC. Antimicrobial actions of nitric oxide. Nitric Oxide (2012) 27:S10. doi:10.1016/j.niox.2012.04.036
79. Barraud N, Kelso MJ, Rice SA, Kjelleberg S. Nitric oxide: A key mediator of biofilm dispersal with applications in infectious diseases. Curr Pharm Des (2015) 21:31–42. doi:10.2174/1381612820666140905112822
80. Wo Y, Brisbois EJ, Bartlett RH, Meyerhoff ME. Recent advances in thromboresistant and antimicrobial polymers for biomedical applications: Just say yes to nitric oxide (NO). Biomater Sci (2016) 4:1161–83. doi:10.1039/c6bm00271d
81. Mimansa K, Tapan B, Arun K, Sandeep A. Understanding the potential role and delivery approaches of nitric oxide in chronic wound healing management. Curr Pharm Des (2020) 26:1–16.
82. Fang FC. Perspectives series: Host/pathogen interactions. Mechanisms of nitric oxide-related antimicrobial activity. J Clin Invest (1997) 99:2818–25. doi:10.1172/jci119473
83. Jones ML, Ganopolsky JG, Labbé A, Wahl C, Prakash S. Antimicrobial properties of nitric oxide and its application in antimicrobial formulations and medical devices. Appl Microbiol Biotechnol (2010) 88:401–7. doi:10.1007/s00253-010-2733-x
84. Rouillard KR, Novak OP, Pistiolis AM, Yang L, Ahonen MJR, Mcdonald RA, et al. Exogenous nitric oxide improves antibiotic susceptibility in resistant bacteria. ACS Infect Dis (2021) 7:23–33. doi:10.1021/acsinfecdis.0c00337
85. Brisbois EJ, Major TC, Goudie MJ, Meyerhoff ME, Bartlett RH, Handa H. Attenuation of thrombosis and bacterial infection using dual function nitric oxide releasing central venous catheters in a 9 day rabbit model. Acta Biomater (2016) 44:304–12. doi:10.1016/j.actbio.2016.08.009
86. Goudie MJ, Pant J, Handa H. Liquid-infused nitric oxide-releasing (LINORel) silicone for decreased fouling, thrombosis, and infection of medical devices. Sci Rep (2017) 7:13623. doi:10.1038/s41598-017-14012-9
87. Devine R, Douglass M, Ashcraft M, Tayag N, Handa H. Development of novel amphotericin B-immobilized nitric oxide-releasing platform for the prevention of broad-spectrum infections and thrombosis. ACS Appl Mater Inter (2021) 13:19613–24. doi:10.1021/acsami.1c01330
88. Cheng J, He K, Shen Z, Zhang G, Yu Y, Hu J. Nitric oxide (NO)-Releasing macromolecules: Rational design and biomedical applications. Front Chem (2019) 7:530. doi:10.3389/fchem.2019.00530
89. Yu H, Cui L-X, Huang N, Yang Z-L. Recent developments in nitric oxide-releasing biomaterials for biomedical applications. Med Gas Res (2019) 9:0–191. doi:10.4103/2045-9912.273956
90. Klee D, Hocker H. Polymers for biomedical applications: Improvement of the interface compatibility. In: Biomedical applications: Polymer blends (1999). p. 1–57.
91. Strijdom H, Chamane N, Lochner A. Nitric oxide in the cardiovascular system: A simple molecule with complex actions. Cardiovasc J Afr (2009) 20:303–10.
92. Carpenter AW, Schoenfisch MH. Nitric oxide release: Part II. Therapeutic applications. Chem Soc Rev (2012) 41:3742–52. doi:10.1039/c2cs15273h
93. Wo Y, Xu L-C, Li Z, Matzger AJ, Meyerhoff ME, Siedlecki CA. Antimicrobial nitric oxide releasing surfaces based on S-nitroso-N-acetylpenicillamine impregnated polymers combined with submicron-textured surface topography. Biomater Sci (2017) 5:1265–78. doi:10.1039/c7bm00108h
94. Xu L-C, Wo Y, Meyerhoff ME, Siedlecki CA. Inhibition of bacterial adhesion and biofilm formation by dual functional textured and nitric oxide releasing surfaces. Acta Biomater (2017) 51:53–65. doi:10.1016/j.actbio.2017.01.030
95. Xu L-C, Meyerhoff ME, Siedlecki CA. Blood coagulation response and bacterial adhesion to biomimetic polyurethane biomaterials prepared with surface texturing and nitric oxide release. Acta Biomater (2019) 84:77–87. doi:10.1016/j.actbio.2018.11.035
96. Frost MC, Reynolds MM, Meyerhoff ME. Polymers incorporating nitric oxide releasing/generating substances for improved biocompatibility of blood-contacting medical devices. Biomaterials (2005) 26:1685–93. doi:10.1016/j.biomaterials.2004.06.006
97. Varu VN, Tsihlis ND, Kibbe MR. Basic science review: Nitric oxide--releasing prosthetic materials. Vasc Endovascular Surg (2009) 43:121–31. doi:10.1177/1538574408322752
98. Cai W, Wu J, Xi C, Meyerhoff ME. Diazeniumdiolate-doped poly(lactic-co-glycolic acid)-based nitric oxide releasing films as antibiofilm coatings. Biomaterials (2012) 33:7933–44. doi:10.1016/j.biomaterials.2012.07.027
99. Handa H, Brisbois EJ, Major TC, Refahiyat L, Amoako KA, Annich GM, et al. Invitro and in vivo study of sustained nitric oxide release coating using diazeniumdiolate-doped poly(vinyl chloride) matrix with poly(lactide-co-glycolide) additive. J Mater Chem B (2013) 1:3578–87. doi:10.1039/c3tb20277a
100. Coneski PN, Schoenfisch MH. Synthesis of nitric oxide-releasing polyurethanes with S-nitrosothiol-containing hard and soft segments. Polym Chem (2011) 2:906–13. doi:10.1039/c0py00269k
101. Laver JR, Mclean S, Bowman LA, Harrison LJ, Read RC, Poole RK. Nitrosothiols in bacterial pathogens and pathogenesis. Antioxid Redox Signal (2013) 18:309–22. doi:10.1089/ars.2012.4767
102. Wo Y, Li Z, Brisbois EJ, Colletta A, Wu J, Major TC, et al. Origin of long-term storage stability and nitric oxide release behavior of carboSil polymer doped with S-Nitroso-N-acetyl-d-penicillamine. ACS Appl Mater Inter (2015) 7:22218–27. doi:10.1021/acsami.5b07501
103. Brisbois EJ, Handa H, Major TC, Bartlett RH, Meyerhoff ME. Long-term nitric oxide release and elevated temperature stability with S-nitroso-N-acetylpenicillamine (SNAP)-doped Elast-eon E2As polymer. Biomaterials (2013) 34:6957–66. doi:10.1016/j.biomaterials.2013.05.063
104. Brisbois EJ, Davis RP, Jones AM, Major TC, Bartlett RH, Meyerhoff ME, et al. Reduction in thrombosis and bacterial adhesion with 7 Day implantation of S-nitroso-N-acetylpenicillamine (SNAP)-Doped elast-eon E2As catheters in sheep. J Mater Chem B (2015) 3:1639–45. doi:10.1039/c4tb01839g
105. Wo Y, Brisbois EJ, Wu J, Li Z, Major TC, Mohammed A, et al. Reduction of thrombosis and bacterial infection via controlled nitric oxide (NO) release from S-nitroso-N-acetylpenicillamine (SNAP) impregnated CarboSil intravascular catheters. ACS Biomater Sci Eng (2017) 3:349–59. doi:10.1021/acsbiomaterials.6b00622
106. Handa H, Major TC, Brisbois EJ, Amoako KA, Meyerhoff ME, Bartlett RH. Hemocompatibility comparison of biomedical grade polymers using rabbit thrombogenicity model for preparing nonthrombogenic nitric oxide releasing surfaces. J Mater Chem B (2014) 2:1059–67. doi:10.1039/c3tb21771j
107. Colletta A, Wu J, Wo Y, Kappler M, Chen H, Xi C, et al. S-Nitroso-N-acetylpenicillamine (SNAP) impregnated silicone Foley catheters: A potential biomaterial/device to prevent catheter-associated urinary tract infections. ACS Biomater Sci Eng (2015) 1:416–24. doi:10.1021/acsbiomaterials.5b00032
108. Stoodley P, Sauer K, Davies DG, Costerton JW. Biofilms as complex differentiated communities. Annu Rev Microbiol (2002) 56:187–209. doi:10.1146/annurev.micro.56.012302.160705
109. Jayaraman A, Wood TK. Bacterial quorum sensing: Signals, circuits, and implications for biofilms and disease. Annu Rev Biomed Eng (2008) 10:145–67. doi:10.1146/annurev.bioeng.10.061807.160536
110. Jenal U, Malone J. Mechanisms of cyclic-di-GMP signaling in bacteria. Annu Rev Genet (2006) 40:385–407. doi:10.1146/annurev.genet.40.110405.090423
111. Römling U, Galperin MY, Gomelsky M. Cyclic di-GMP: The first 25 Years of a universal bacterial second messenger. Microbiol Mol Biol Rev (2013) 77:1–52. doi:10.1128/mmbr.00043-12
112. Jenal U, Reinders A, Lori C. Cyclic di-GMP: Second messenger extraordinaire. Nat Rev Microbiol (2017) 15:271–84. doi:10.1038/nrmicro.2016.190
113. Yang Y, Li Y, Gao T, Zhang Y, Wang Q. C-di-GMP turnover influences motility and biofilm formation in Bacillus amyloliquefaciens PG12. Res Microbiol (2018) 169:205–13. doi:10.1016/j.resmic.2018.04.009
114. Suchanek VM, Esteban-López M, Colin R, Besharova O, Fritz K, Sourjik V. Chemotaxis and cyclic-di-GMP signalling control surface attachment of Escherichia coli. Mol Microbiol (2020) 113:728–39. doi:10.1111/mmi.14438
115. Hengge R. Principles of c-di-GMP signalling in bacteria. Nat Rev Microbiol (2009) 7:263–73. doi:10.1038/nrmicro2109
116. Valentini M, Filloux A. Biofilms and cyclic di-GMP (c-di-GMP) signaling: Lessons from Pseudomonas aeruginosa and other bacteria. J Biol Chem (2016) 291:12547–55. doi:10.1074/jbc.r115.711507
117. Thormann KM, Duttler S, Saville RM, Hyodo M, Shukla S, Hayakawa Y, et al. Control of formation and cellular detachment from Shewanella oneidensis MR-1 biofilms by cyclic di-GMP. J Bacteriol (2006) 188:2681–91. doi:10.1128/jb.188.7.2681-2691.2006
118. Cotter PA, Stibitz S. c-di-GMP-mediated regulation of virulence and biofilm formation. Curr Opin Microbiol (2007) 10:17–23. doi:10.1016/j.mib.2006.12.006
119. Chen Y, Chai Y, Guo J-H, Losick R. Evidence for cyclic di-GMP-mediated signaling in Bacillus subtilis. J Bacteriol (2012) 194:5080–90. doi:10.1128/jb.01092-12
120. Ha D-G, O'toole GA. c-di-GMP and its effects on biofilm formation and dispersion: A Pseudomonas aeruginosa review. Microbiol Spectr (2015) 3–0003-2014. doi:10.1128/microbiolspec.mb-0003-2014
121. Kulasakara H, Lee V, Brencic A, Liberati N, Urbach J, Miyata S, et al. Analysis of Pseudomonas aeruginosa diguanylate cyclases and phosphodiesterases reveals a role for bis-(3'-5')-cyclic-GMP in virulence. Proc Natl Acad Sci U S A (2006) 103:2839–44. doi:10.1073/pnas.0511090103
122. Yang S, Wu Y, Qu C, Fein JB, He Y, Huang Q, et al. Quantitative analysis of the surficial and adhesion properties of the Gram-negative bacterial species Comamonas testosteroni modulated by c-di-GMP. Colloids Surf B: Biointerfaces (2021) 198:111497. doi:10.1016/j.colsurfb.2020.111497
123. Townsley L, Yildiz FH. Temperature affects c-di-GMP signalling and biofilm formation in Vibrio cholerae. Environ Microbiol (2015) 17:4290–305. doi:10.1111/1462-2920.12799
124. Rodesney CA, Roman B, Dhamani N, Cooley BJ, Katira P, Touhami A, et al. Mechanosensing of shear by Pseudomonas aeruginosa leads to increased levels of the cyclic-di-GMP signal initiating biofilm development. Proc Natl Acad Sci U S A (2017) 114:5906–11. doi:10.1073/pnas.1703255114
125. Song F, Wang H, Sauer K, Ren D. Cyclic-di-GMP and oprF are involved in the response of Pseudomonas aeruginosa to substrate material stiffness during attachment on polydimethylsiloxane (PDMS). Front Microbiol (2018) 9:Article110. doi:10.3389/fmicb.2018.00110
126. Barraud N, Hassett DJ, Hwang S-H, Rice SA, Kjelleberg S, Webb JS. Involvement of nitric oxide in biofilm dispersal of Pseudomonas aeruginosa. J Bacteriol (2006) 188:7344–53. doi:10.1128/jb.00779-06
127. Barraud N, Storey MV, Moore ZP, Webb JS, Rice SA, Kjelleberg S. Nitric oxide-mediated dispersal in single- and multi-species biofilms of clinically and industrially relevant microorganisms. Microb Biotechnol (2009) 2:370–8. doi:10.1111/j.1751-7915.2009.00098.x
128. Barraud N, Schleheck D, Klebensberger J, Webb JS, Hassett DJ, Rice SA, et al. Nitric oxide signaling in Pseudomonas aeruginosa biofilms mediates phosphodiesterase activity, decreased cyclic di-GMP levels, and enhanced dispersal. J Bacteriol (2009) 191:7333–42. doi:10.1128/jb.00975-09
Keywords: biomaterials, infection, blood, surface modification, biocompability
Citation: Xu L-C and Siedlecki CA (2022) Surface texturing and combinatorial approaches to improve biocompatibility of implanted biomaterials. Front. Phys. 10:994438. doi: 10.3389/fphy.2022.994438
Received: 14 July 2022; Accepted: 31 October 2022;
Published: 16 November 2022.
Edited by:
Sungsook Ahn, Cook Medical INC, United StatesCopyright © 2022 Xu and Siedlecki. This is an open-access article distributed under the terms of the Creative Commons Attribution License (CC BY). The use, distribution or reproduction in other forums is permitted, provided the original author(s) and the copyright owner(s) are credited and that the original publication in this journal is cited, in accordance with accepted academic practice. No use, distribution or reproduction is permitted which does not comply with these terms.
*Correspondence: Christopher A. Siedlecki, Y3NpZWRsZWNraUBwc3UuZWR1