- Ruđer Bošković Institute, Zagreb, Croatia
Multinucleon transfer reactions, characterized by the exchange of many nucleons at energies in the vicinity of the Coulomb barrier, have been extensively used in the last decades to understand the production of neutron-rich nuclei, as well as to study their structure. In this Mini Review, recent results related to the production mechanism of heavy neutron-rich nuclei obtained with stable and radioactive beams will be discussed together with the results concerning the proton transfer channels. Additionally, newest results from a series of experiments carried out to study nucleon-nucleon correlations for closed-shell and superfluid systems employing the large solid angle magnetic spectrometer PRISMA will be summarized.
Introduction
Multinucleon transfer (MNT) reactions between heavy ions at energies around the Coulomb barrier are characterized by the exchange of many nucleons between the target and the projectile with a population of relatively low excitation energy and of relatively high spins [1]. They have been extensively used in the last decades to populate moderately neutron-rich mid-mass nuclei with cross sections large enough to study their structure [1–3]. Specifically, valuable information on single-particle states, collective excitations, and their coupling [4–13], are offered by one-nucleon transfer channels, while transfer of nucleon pairs yields information on nucleon-nucleon correlations [14–17]. As more nucleons are getting transferred, it is possible to populate nuclei farther from the stability and to study the evolution of reaction from quasi-elastic to deep-inelastic regime.
In the quasi-elastic regime, the mass and charge distributions of transfer products are governed by optimum Q-value considerations and transfer form factors [1]. As a result, the neutron pick-up and the proton stripping channels of the projectile-like fragments are dominantly populated when lighter stable projectiles are used on heavy targets [1]. The transfer flux changes already with the use of more neutron-rich stable projectiles in which case proton pick-up channels open up [18]. With neutron-rich projectiles, the trend should turn and the proton pick-up and neutron stripping channels should dominate, leading to the population of neutron-rich heavy fragments [18–23]. This pathway is very interesting for nuclear structure investigations, for example for the understanding of the evolution of magic numbers far from stability [24–26], and for nuclear astrophysics investigations, where heavy-element synthesis in the r-process is particularly intriguing [27, 28].
MNT as a method to produce heavy neutron-rich nuclei
Multinucleon transfer reactions were predicted to be a competitive method for the production of heavy neutron-rich nuclei in the pivotal work of Dasso and collaborators [19]. More recently, it has been predicted that the neutron-rich region around the magic number N = 126 can be approached by using stable beams at energies around the Coulomb barrier in deep-inelastic collisions (DIC) [29]. These predictions were experimentally studied in the last years with careful selection of the colliding systems which are suitable to populate proton pick-up channels at energies around the Coulomb barrier. Many of these studies are based on the powerful combination of selective large solid angle magnetic spectrometers such as PRISMA [30–32] in Legnaro National Laboratories in Italy and efficient γ-ray detectors [2, 33, 34]. Even so, the study of proton pick-up channels is still in its beginnings and reaction products have been identified in atomic number, mass and Q-value for only a few systems.
In this section we will present our selection of some of the measurements concerning the production of heavy neutron-rich nuclei. Measurements have been performed for the 144Sm+88Sr [35] and 48Ca+124Sn [36] systems to study nucleon correlation effects and complex (i.e., pair/cluster) degrees of freedom in the transfer process. On the other hand, indirect measurements of cross sections based on the knowledge of the level schemes, using characteristic γ rays, were performed for few heavy systems [37–39].
Recently a high resolution study of the absolute production cross sections of neutron-rich nuclei in the 136Xe+198Pt system [22] gained a lot of attention. Measurement performed with the VAMOS++ magnetic spectrometer [40], to identify light reaction partner, and EXOGAM γ-array [41] showed that the main contribution to the formation of heavy neutron-rich nuclei such as Hg and Os arise in collisions with a small excitation energies where the particle, mainly neutron, evaporation is minimized. Experimental results have been compared with the GRAZING code [42–44] that calculates the evolution of the reaction by taking into account, besides the relative motion, the intrinsic degrees of freedom of projectile and target. These are the isoscalar surface modes and the single-nucleon transfer channels. The multinucleon transfer channels are described via a multistep mechanism. The relative motion of the system is calculated in a nuclear plus Coulomb field. The model, to calculate the isotopic distributions of the produced fragments, takes into account, in a simple way, the effect of neutron evaporation. The code has been successfully applied in the description of MNT reactions and in general gives good description of the neutron and few proton transfer channels. However, it was observed that for more proton stripping and pick-up channels the measured distributions peak at lower and higher mass values, respectively, as compared to the GRAZING calculations that take into account only impact parameters close to the grazing angle. The difference from the calculation indicates the presence of large energy losses and significant influence of neutron evaporation.
The total cross sections of proton pick-up channels were further measured for the 40Ar+208Pb system where the focus was on obtaining the total transfer strength [18]. This was achieved by measuring the angular distribution for the light reaction partners over large range of angles spanning three angular and magnetic field settings of the PRISMA spectrometer and by taking into account the spectrometer’s response function [45, 46]. This allowed to study the evolution of quasi-elastic to DIC. Disentangling between different contributions is challenging from both experimental and theoretical side since they are substantially mixed and may strongly overlap. However, insight was gained by identifying reaction products in mass and charge, and by measuring total kinetic energy loss (TKEL) and angular distributions over wide range of angles, as well as by comparing these experimental observables with theoretical calculations. As can be seen from Figure 1A, the deviations between experimental data and GRAZING calculations are more pronounced for the proton (especially pick-up) channels, when neutron transfer channels are involved. The contributions from DIC were extracted and identified to be significant. This is especially relevant at energies substantially higher than the Coulomb barrier, where DIC, as well as secondary effects, become more and more relevant.
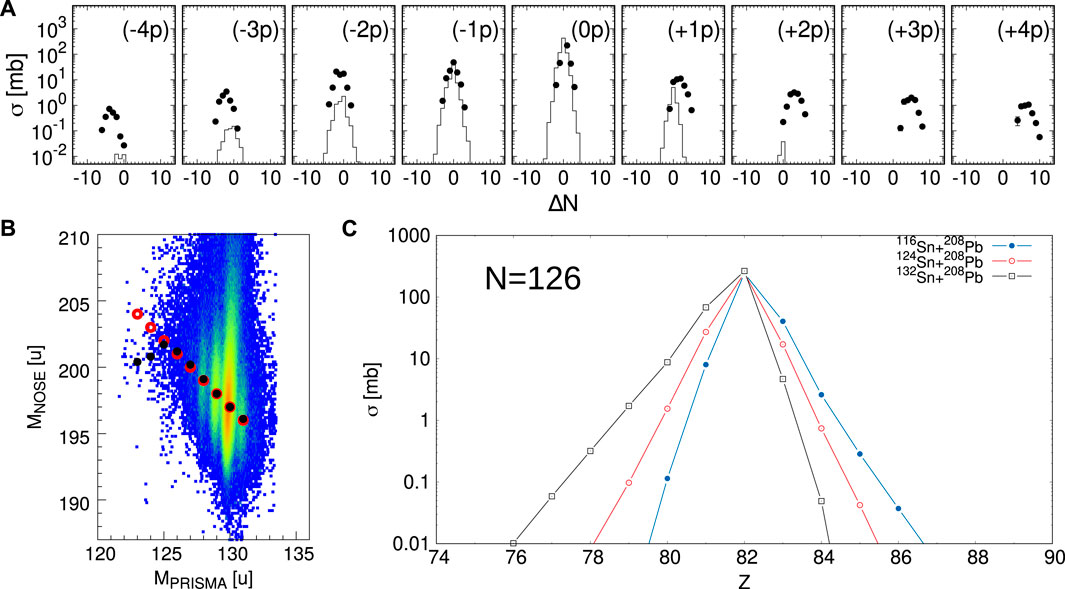
FIGURE 1. (A) Total experimental cross section for the 40Ar+208Pb system, at beam energy Elab = 6.4 MeV/A (points), and the GRAZING calculations with neutron evaporation (solid line), figure is adapted with permission from [18] (B) Mass-mass correlation matrix of Te isotopes detected in PRISMA and the heavy partner detected in coincidence with NOSE for the 197Au+130Te system. The red circles indicate the centroids of the correlated masses of the primary neutron transfer channels, the black dots indicate the experimental centroids as derived from the fits of their projections [47] (C) GRAZING calculations for the 116Sn (stable), 124Sn (the most neutron-rich stable) and 132Sn (neutron-rich) projectiles on the 208Pb target at the beam energy 20% above the Coulomb barrier.
Secondary processes, such as neutron evaporation and fission, especially from 238U, can significantly modify the final yield distribution of heavy primary nuclei mainly towards the lighter isotopes. It is important to quantitatively understand their relevance and to study the best experimental conditions for the largest survival probability of the neutron-rich nuclei populated in MNT reactions. The cross section studies for proton pick-up channels have been performed with the 238U target for the 64Ni+238U [20] and 136Xe+238U [21] systems. In the latter case, studies of the influence of secondary processes on MNT were carried out by measuring the time-of-flight differences between different coincident reaction products. In this way it was possible to distinguish between transfer, fission and elastically scattered particles which helped to determine the fraction of MNT reaction products compared to fission. Neutron evaporation channels were clearly identified thanks to AGATA [33, 34] and it was observed that as more neutrons are transferred to the target nucleus, more evaporated neutrons are detected. This strongly affects the final yield distribution of both binary partners and limits the production of very neutron-rich nuclei. It is important to note that proton pick-up channels have higher survival probability than stripping channels, leading to more neutron-rich final reaction products.
Although information on the heavy partner can be obtained indirectly by detecting the coincident γ rays produced by the reaction products [21, 31], significant progress has been made with a simultaneous detection of light and heavy transfer products in the 197Au + 130Te system [47]. The high-resolution kinematic coincidence measurement has been performed with PRISMA coupled to a second arm detector NOSE [48]. This helped, via a mass-mass correlation, to understand and quantify the production process also for the heavy partner of the reaction. To better understand the effect of secondary processes, the de-excitation process of the produced heavy fragments has been simulated with a Monte Carlo method, starting from the binary character of the reaction, to understand their final mass distribution. These results, shown in Figure 1B, indicated that the primary fragments acquire significant excitation energy and allowed to extract information on the average number of evaporated neutrons for each channel associated with the Te isotopes. The focus was on pure neutron transfer channels and the extracted total cross sections well agree with calculations performed with the GRAZING code down to several neutron transfers. However, a better knowledge of the underlying mechanisms is essential considering that the main excitation energy of the recoils can be rather high.
A definite dominance of the proton pick-up and neutron stripping channels in the distribution of the transfer flux of the light partner, leading to the proton stripping and neutron pick-up in the heavy partner of the reaction, is predicted to occur by using neutron-rich projectiles, in most cases, five to seven neutrons away from the last stable isotope [19]. Results of the GRAZING calculations for the 116Sn (stable), 124Sn (the most neutron-rich stable) and 132Sn (neutron-rich) projectiles on the 208Pb target at the beam energy 20% above the Coulomb barrier are shown in Figure 1C for the reaction products with N = 126. The shift toward more neutron-rich reaction products with Z < 82 is clearly seen, and calculations predict an order of magnitude larger cross section when using neutron-rich rare-isotope beam. Experimentally, first step in this direction is the absolute cross sections determination for neutron transfer channels populated in the 94Rb+208Pb reaction [23]. Cross sections have been extracted by directly identifying the lead isotopes with the high-efficiency MINIBALL γ-ray array [49]. The observed sizable cross sections in the neutron-rich mass region are in fair agreement with the GRAZING calculations. This confirms the predicted change of population pattern and is of great importance for future studies of nuclear structure, in particular near the N = 126 shell closure, with MNT reactions and radioactive beams. These studies will be considerably improved with the particle detection systems able to identify reaction products in A and Z and by measuring the cross sections to the ground states which can be substantial.
Experimental results confirm that MNT reactions are a suitable tool to produce exotic neutron-rich nuclei [22, 23]. In comparison with fragmentation and fission, the production cross sections of nuclei along N = 126, Z < 82 with MNT reactions appear to be much larger, especially for lower atomic numbers [22]. However, one should take into account the larger target thicknesses and experimental efficiencies in the fragmentation reactions [50, 51]. Even so, it is still of great interest to continue these studies to see if MNT reactions and radioactive beams offer access to neutron-rich region south of doubly magic Pb nucleus, that is out of reach for other methods.
Finally, different theoretical models are becoming available partially thanks to the use of super-computers. These developments in reaction theory must consider the adequate treatment of the reaction dynamics, realistic structure models and proper effective interactions. Some of the newer approaches include the improved quantum molecular dynamics (ImQMD) model [52–56] and time-dependent Hartree-Fock (TDHF) theory [57–66]. Nowadays it is possible to take into account quantal fluctuations and correlations going beyond the TDFH approach [67–70]. Therefore, precise measurements of experimental observables, especially absolute cross sections, angular and TKEL distributions, and disentangling between different effects that contribute to the reaction cross section, can be used to validate different theoretical models in order to optimize the population of the exotic nuclei of interest. This comparison of different models and experimental data should give better insight in the MNT reaction mechanism and help to develop our understanding of low-energy heavy-ion reactions.
Nucleon-nucleon correlations
Two-nucleon transfer reactions are among the best tools to investigate nucleon-nucleon correlations which are induced by the pairing interaction [71–74]. With heavy ions, the reaction dynamics is complex and only recently microscopic calculations achieved a good agreement with the experimental data [14, 15, 17]. This was possible because measurements were performed in a wide energy range from near to well below the Coulomb barrier where the interacting nuclei are only slightly influenced by the nuclear potential and Q values are restricted to a few MeV for the open transfer channels. These conditions diminish the complexity of coupled channel calculations. The data were represented via the transfer probability (Ptr) (defined as a ratio of the transfer yield over the quasielastic one) plotted as a function of the distance of closest approach (D) for the Coulomb trajectory. The use of the PRISMA spectrometer and inverse kinematics allowed one to measure Ptr down to very large values of D, sufficiently far from the nuclear absorption region, with good ion identification, and information could be extracted on the nucleon-nucleon correlations.
The total transfer probability for one-neutron transfer channel was obtained by summing over all possible transitions that can be constructed from the single-particle states in the projectile and target for the 96Zr+40Ca [14] and 116Sn+60Ni [15, 16] systems and the results are shown in Figure 2. These calculations well reproduce the experimental slope and the absolute values of the transfer probabilities. For the two-nucleon transfer, the transfer probability was calculated for the 0+ states. The ground-to-ground state transition alone could not reproduce experimental result for the 96Zr+40Ca system as can be seen in Figure 2A. In fact, the predicted transfer probability for the transition to the excited 0+ state in 42Ca is much larger, in agreement with the measured Q-value spectra. In conclusion, it was found that the two-neutron transfer probability is enhanced as compared to a simple expectation of independent particle transfer process, i.e., the square of one-neutron transfer probability. The coupled-channels calculations performed for this system also suggested a significant effect of the couplings to the collective excited states around the Coulomb barrier [75]. The TDHF + BCS formalism was also applied for this type of reactions [76, 77].
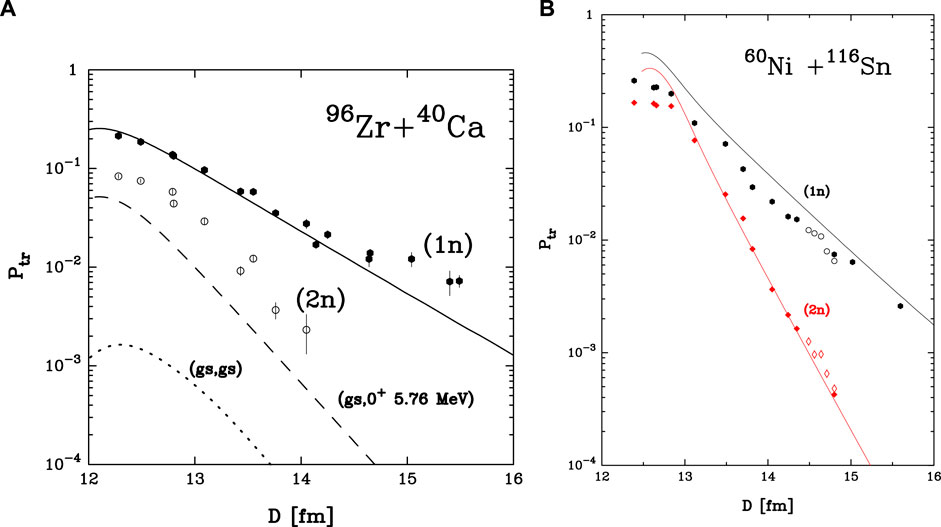
FIGURE 2. Theoretical transfer probabilities Ptr for the one- and two-neutron transfer (lines) in comparison with the experimental data (symbols) for the 96Zr+40Ca (A) and 116Sn+60Ni (B) systems plotted as a function of the distance of closest approach D (A) The full line represents the inclusive transfer probability for one-neutron transfer, the dotted line the ground-to-ground state transition for the two-neutron transfer, and the dashed line the transition to the first 0+ excited state at 5.76 MeV in 42Ca [14] (B) Open symbols correspond to the results from the angular distribution in direct kinematics while solid symbols refer to the excitation function measurement performed in inverse kinematics [16].
The experimental transfer probabilities for 116Sn+60Ni shown in Figure 2B have been well reproduced, for the first time with heavy ions for the two-neutron transfer channel, in absolute values and in slope by microscopic calculations which incorporate nucleon-nucleon pairing correlations, microscopically calculated optical potentials and successive transfer processes [15]. In particular, the employed microscopic theory reproduces very well the data by considering solely the ground-to-ground state transition. This was possible because in this system (superfluid nuclei) the ground-to-ground state Q values for one- and two-neutron transfer are very close to optimum (∼0 MeV). The validity of this approach was confirmed by performing a fragment-γ coincidence experiment for the same system [16], employing the PRISMA spectrometer coupled to the AGATA demonstrator [33]. It was possible to conclude that a large fraction of the total strength of the (2n) channel, more than 76%, goes to the ground state.
Recent theoretical calculations interpreted 116Sn+60Ni data as a manifestation of a nuclear (alternating current) Josephson effect with Cooper pairs tunneling between the superfluid nuclei [17, 78, 79]. The coherence length was determined thanks to the large range of D over which transfer probability was measured. A transfer of a neutron pair between interacting binary partners generates a dipole oscillation whose frequency is determined by the ground state Q-value [17]. The short interaction time of scattering,
More work is needed in order to better understand the role of a transfer of a pair by confronting theory with experiments involving different nuclei. In this respect, valuable insight will be given by the 206Pb+118Sn system [80, 81] recently measured with PRISMA. This is the heaviest (asymmetric) semi-magic system with closed proton and open neutron shells, and well Q-value matched for neutron transfers. Important questions that can be answered in this case are whether and to what extent the effect of neutron-neutron correlations is modified in the presence of high Coulomb fields and do DIC and multistep processes significantly modify the transfer strength near the ground states. The transfer strength of the ground-to-ground state transitions may significantly change in the collisions of very heavy ions thanks to the population of final states with high excitation energies and large angular momenta. Additionally, it would be interesting to see whether any enhancement factors can be observed in the collisions of two doubly magic nuclei.
Nucleon-nucleon correlations studies should be extended to even less understood role of neutron-proton and proton-proton correlations [82]. The study of neutron-proton correlations has been done with nuclei along the N = Z line to investigate the isovector and isoscalar components (for some of the recent results see Refs. [83–88]), while the data for the proton-proton correlations are more scarce [82]. It would be of great interest to extend these studies to transfer reactions with heavy ions even if the transfer probabilities for protons are generally smaller at the same D and more difficulties are encountered than in the case of neutrons from both experimental and theoretical point of view. These measurements can be advanced by the coupling of a spectrometer and an efficient γ-array which could provide important additional information for both one and two-proton transfer.
Finally, the advent of new radioactive beam facilities [89–92] should provide access to the nuclei with an extended neutron distribution and should allow the possibility to study the density dependence of the pairing force. Particularly interesting is the (closed shell) region of 132Sn and beyond to look at the modification of the properties of pair transfers. Some work has been done with the halo nuclei, as for instance with 11Li induced reactions providing evidence of phonon mediated pairing [93, 94]. The comparison of data concerning the dynamic effects of pairing correlations with different microscopic theories (for instance [15, 17, 95]), as well as cross comparison between reactions performed with stable and radioactive beams, will provide valuable insight into the role of the pair (neutron and/or proton) transfer in the MNT reactions.
Author contributions
TM wrote the manuscript on behalf of the PRISMA collaboration.
Funding
This work has been supported in part by the Croatian Science Foundation under Project no. 7194 and under Project no. IP-2018-01-1257, as well as Center of Excellence for Advanced Materials and Sensing Devices (CEMS). The research leading to these results has received funding from the European Union Seventh Framework Programme FP7/2007- 2013 under Grant Agreement 262010 - ENSAR.
Acknowledgments
The author acknowledges the support of the PRISMA, CLARA and AGATA collaborations, in particular L. Corradi, E. Fioretto, F. Galtarossa, A. Goasduff, G. Montagnoli, D. Montanari, G. Pollarolo, A.M. Sefanini, S. Szilner whose work is presented in this article.
Conflict of interest
The author declares that the research was conducted in the absence of any commercial or financial relationships that could be construed as a potential conflict of interest.
Publisher’s note
All claims expressed in this article are solely those of the authors and do not necessarily represent those of their affiliated organizations, or those of the publisher, the editors and the reviewers. Any product that may be evaluated in this article, or claim that may be made by its manufacturer, is not guaranteed or endorsed by the publisher.
References
1. Corradi L, Pollarolo G, Szilner S. Multinucleon transfer processes in heavy-ion reactions. J Phys G: Nucl Part Phys (2009) 36:113101. doi:10.1088/0954-3899/36/11/113101
2. Gadea A, Napoli DR, de Angelis G, Menegazzo R, Stefanini AM, Corradi L, et al. Coupling a CLOVER detector array with the PRISMA magnetic spectrometer. Eur Phys J A (2003) 20:193–7. doi:10.1140/epja/i2002-10352-9
3. Bracco A, Duchêne G, Podolyák Z, Reiter P. Gamma spectroscopy with AGATA in its first phases: New insights in nuclear excitations along the nuclear chart. Prog Part Nucl Phys (2021) 121:103887. doi:10.1016/j.ppnp.2021.103887
4. Liang X, Azaiez F, Chapman R, Haas F, Bazzacco D, Beghini S, et al. Study of the neutron-rich nucleus Si36. Phys Rev C (2006) 74:014311. doi:10.1103/PhysRevC.74.014311
5. Lunardi S, Lenzi SM, Vedova FD, Farnea E, Gadea A, Mărginean N, et al. Spectroscopy of neutron-rich Fe isotopes populated in the 64Ni+238U reaction. Phys Rev C (2007) 76:034303. doi:10.1103/physrevc.76.034303
6. Fornal B, Janssens RVF, Broda R, Marginean N, Beghini S, Corradi L, et al. Yrast structure of the neutron-rich N = 31 isotones 51Ca and 52Sc. Phys Rev C (2008) 77:014304. doi:10.1103/physrevc.77.014304
7. Valiente-Dobón JJ, Lenzi SM, Freeman SJ, Lunardi S, Smith JF, Gottardo A, et al. Spectroscopy of neutron-rich 59−63Mn isotopes. Phys Rev C (2008) 78:024302. doi:10.1103/PhysRevC.78.024302
8. Montanari D, Leoni S, Mengoni D, Benzoni G, Blasi N, Bocchi G, et al. Probing the nature of particle-core couplings in 49Ca with γ spectroscopy and heavy-ion transfer reactions. Phys Lett B (2011) 697:288–93. doi:10.1016/j.physletb.2011.01.046
9. Szilner S, Corradi L, Haas F, Lebhertz D, Pollarolo G, Ur CA, et al. Interplay between single-particle and collective excitations in argon isotopes populated by transfer reactions. Phys Rev C (2011) 84:014325. doi:10.1103/physrevc.84.014325
10. Recchia F, Lenzi SM, Lunardi S, Farnea E, Gadea A, Mărginean N, et al. Spectroscopy of odd-mass cobalt isotopes toward the N = 40 subshell closure and shell-model description of spherical and deformed states. Phys Rev C (2012) 85:064305. doi:10.1103/PhysRevC.85.064305
11. Szilner S, Corradi L, Haas F, Pollarolo G, Angus L, Beghini S, et al. Structure of chlorine isotopes populated by heavy ion transfer reactions. Phys Rev C (2013) 87:054322. doi:10.1103/PhysRevC.87.054322
12. Varga Pajtler M, Szilner S, Corradi L, de Angelis G, Fioretto E, Gadea A, et al. Selective properties of neutron transfer reactions in the 90Zr + 208Pb system for the population of excited states in zirconium isotopes. Nucl Phys A (2015) 941:273–92. doi:10.1016/j.nuclphysa.2015.07.007
13. Chapman R, Hodsdon A, Bouhelal M, Haas F, Liang X, Azaiez F, et al. Spectroscopy of neutron-rich 34,35,36,37,38P populated in binary grazing reactions. Phys Rev C (2015) 92:044308. doi:10.1103/PhysRevC.92.044308
14. Corradi L, Szilner S, Pollarolo G, Colò G, Mason P, Farnea E, et al. Single and pair neutron transfers at sub-barrier energies. Phys Rev C (2011) 84:034603. doi:10.1103/PhysRevC.84.034603
15. Montanari D, Corradi L, Szilner S, Pollarolo G, Fioretto E, Montagnoli G, et al. Neutron pair transfer in Ni60+Sn116 Far below the Coulomb barrier. Phys Rev Lett (2014) 113:052501. doi:10.1103/PhysRevLett.113.052501
16. Montanari D, Corradi L, Szilner S, Pollarolo G, Goasduff A, Mijatović T., et al. Pair neutron transfer in 60Ni+116Sn probed viaγ-particle coincidences. Phys Rev C (2016) 93:054623. doi:10.1103/PhysRevC.93.054623
17. Potel G, Barranco F, Vigezzi E, Broglia RA Quantum entanglement in nuclear Cooper-pair tunneling with γ rays. Phys Rev C (2021) 103:L021601. doi:10.1103/PhysRevC.103.L021601
18. Mijatović T, Szilner S, Corradi L, Montanari D, Pollarolo G, Fioretto E, et al. Multinucleon transfer reactions in the 40Ar+208Pb system. Phys Rev C (2016) 94:064616. doi:10.1103/PhysRevC.94.064616
19. Dasso CH, Pollarolo G, Winther A Systematics of isotope production with radioactive beams. Phys Rev Lett (1994) 73:1907–10. doi:10.1103/PhysRevLett.73.1907
20. Corradi L, Stefanini AM, Lin CJ, Beghini S, Montagnoli G, Scarlassara F, et al. Multinucleon transfer processes in 64Ni+238U. Phys Rev C (1999) 59:261–8. doi:10.1103/PhysRevC.59.261
21. Vogt A, Birkenbach B, Reiter P, Corradi L, Mijatović T, Montanari D, et al. Light and heavy transfer products in 136Xe+238U multinucleon transfer reactions. Phys Rev C (2015) 92:024619. doi:10.1103/PhysRevC.92.024619
22. Watanabe YX, Kim YH, Jeong SC, Hirayama Y, Imai N, Ishiyama H, et al. Pathway for the production of neutron-rich isotopes around the N = 126 Shell closure. Phys Rev Lett (2015) 115:172503. doi:10.1103/physrevlett.115.172503
23. Čolović P, Szilner S, Illana A, Valiente-Dobón JJ, Corradi L, Pollarolo G, et al. Population of lead isotopes in binary reactions using a 94Rb radioactive beam. Phys Rev C (2020) 102:054609. doi:10.1103/PhysRevC.102.054609
24. Caurier E, Martínez-Pinedo G, Nowacki F, Poves A, Zuker AP. The shell model as a unified view of nuclear structure. Rev Mod Phys (2005) 77:427–88. doi:10.1103/RevModPhys.77.427
25. Broda R, Iskra LW, Janssens RVF, Brown BA, Fornal B, Wrzesiński J, et al. Two-neutron and core-excited states in 210Pb: Tracing E3 collectivity and evidence for a new β -decaying isomer in 210Tl. Phys Rev C (2018) 98:024324. doi:10.1103/PhysRevC.98.024324
26. Tang TL, Kay BP, Hoffman CR, Schiffer JP, Sharp DK, Gaffney LP, et al. First exploration of neutron shell structure below lead and beyond N = 126. Phys Rev Lett (2020) 124:062502. doi:10.1103/PhysRevLett.124.062502
27. Kajino T, Aoki W, Balantekin A, Diehl R, Famiano M, Mathews G Current status of r-process nucleosynthesis. Prog Part Nucl Phys (2019) 107:109–66. doi:10.1016/j.ppnp.2019.02.008
28. Horowitz CJ, Arcones A, Côté B, Dillmann I, Nazarewicz W, Roederer IU r-process nucleosynthesis: connecting rare-isotope beam facilities with the cosmos. J Phys G: Nucl Part Phys (2019) 46:083001. doi:10.1088/1361-6471/ab0849
29. Zagrebaev V, Greiner W Production of new heavy isotopes in low-energy multinucleon transfer reactions. Phys Rev Lett (2008) 101:122701. doi:10.1103/PhysRevLett.101.122701
30. Stefanini A, Corradi L, Maron G, Pisent A, Trotta M, Vinodkumar A The heavy-ion magnetic spectrometer PRISMA. Nucl Phys A (2002) 701:217–21. doi:10.1016/S0375-9474(01)01578-0
31. Szilner S, Ur CA, Corradi L, Mărginean N, Pollarolo G, Stefanini AM, et al. Multinucleon transfer reactions in closed-shell nuclei. Phys Rev C (2007) 76:024604. doi:10.1103/PhysRevC.76.024604
32. Corradi L, Szilner S, Pollarolo G, Montanari D, Fioretto E, Stefanini A, et al. Multinucleon transfer reactions: Present status and perspectives. Nucl Instr Methods Phys Res Section B: Beam Interactions Mater Atoms (2013) 317:743–51. XVIth International Conference on ElectroMagnetic Isotope Separators and Techniques Related to their Applications, December 2–7, 2012 at Matsue, Japan. doi:10.1016/j.nimb.2013.04.093
33. Akkoyun S, Algora A, Alikhani B, Ameil F, de Angelis G, Arnold L, et al. Agata - advanced GAmma tracking array. Nucl Instr Methods Phys Res Section A: Acc Spectrometers, Detectors Associated Equipment (2012) 668:26–58. doi:10.1016/j.nima.2011.11.081
34. Gadea A, Farnea E, Valiente-Dobón J, Million B, Mengoni D, Bazzacco D, et al. Conceptual design and infrastructure for the installation of the first AGATA sub-array at LNL. Nucl Instr Methods Phys Res Section A: Acc Spectrometers, Detectors Associated Equipment (2011) 654:88. doi:10.1016/j.nima.2011.06.004
35. Künkel R, Oertzen W, Bohlen HG, Gebauer B, Bösser HA, Kohlmeyer B, et al. Pairing effects in nucleon transfer reactions in the system 144Sm+88Sr at 4.7 MeV/u. Z Physik A - At Nuclei (1990) 336:71–89. doi:10.1007/BF01904165
36. Corradi L, Stefanini AM, He JH, Beghini S, Montagnoli G, Scarlassara F. Evidence of complex degrees of freedom in multinucleon transfer reactions of 48Ca+124Sn. Phys Rev C (1997) 56:938–41. doi:10.1103/PhysRevC.56.938
37. Broda R. Spectroscopic studies with the use of deep-inelastic heavy-ion reactions. J Phys G: Nucl Part Phys (2006) 32:R151–R192. doi:10.1088/0954-3899/32/6/r01
38. Barrett JS, Loveland W, Yanez R, Zhu S, Ayangeakaa AD, Carpenter MP., et al. 136Xe+208Pb reaction: A test of models of multinucleon transfer reactions. Phys Rev C (2015) 91:064615. doi:10.1103/PhysRevC.91.064615
39. Welsh T, Loveland W, Yanez R, Barrett J, McCutchan E, Sonzogni A., et al. Modeling multi-nucleon transfer in symmetric collisions of massive nuclei. Phys Lett B (2017) 771:119–24. doi:10.1016/j.physletb.2017.05.044
40. Rejmund M, Lecornu B, Navin A, Schmitt C, Damoy S, Delaune O. Performance of the improved larger acceptance spectrometer: VAMOS++. Nucl Instr Methods Phys Res Section A: Acc Spectrometers, Detectors Associated Equipment (2011) 646:184–91. doi:10.1016/j.nima.2011.05.007
41. Simpson J, Azaiez F, deFrance G, Fouan J, Gerl J, Julin R, et al. The EXOGAM array: A radioactive beam gamma-ray spectrometer. Acta Physica Hungarica, Ser A: Heavy Ion Phys (2000) 11:159–88.
42. Winther A. Grazing reactions in collisions between heavy nuclei. Nucl Phys A (1994) 572:191–235. doi:10.1016/0375-9474(94)90430-8
43. Winther A. Dissipation, polarization and fluctuation in grazing heavy-ion collisions and the boundary to the chaotic regime. Nucl Phys A (1995) 594:203–45. doi:10.1016/0375-9474(95)00374-A
44.[Dataset] Winther A. Program GRAZING (2002). Available from: http://www.to.infn.it/∼nanni/grazing. (Accessed July 12).
45. Montanari D, Farnea E, Leoni S, Pollarolo G, Corradi L, Benzoni G, et al. Response function of the magnetic spectrometer PRISMA. Eur Phys J A (2011) 47:4. doi:10.1140/epja/i2011-11004-9
46. Mijatović T, Szilner S, Corradi L, Montanari D, Pollarolo G, Fioretto E, et al. Study of the cross section determination with the PRISMA spectrometer: The 40Ar + 208Pb case. Eur Phys J A (2016) 52:113. doi:10.1140/epja/i2016-16113-3
47. Galtarossa F, Corradi L, Szilner S, Fioretto E, Pollarolo G, Mijatović T, et al. Mass correlation between light and heavy reaction products in multinucleon transfer 197Au+130Te collisions. Phys Rev C (2018) 97:054606. doi:10.1103/PhysRevC.97.054606
48. Fioretto E, Galtarossa F, Corradi L, Jia H, Collini F, Marchi T, et al. A gas detection system for fragment identification in low-energy heavy-ion collisions. Nucl Instr Methods Phys Res Section A: Acc Spectrometers, Detectors Associated Equipment (2018) 899:73–9. doi:10.1016/j.nima.2018.05.011
49. Warr N, Van de Walle J, Albers M, Ames F, Bastin B, Bauer C, et al. The Miniball spectrometer. Eur Phys J A (2013) 49. doi:10.1140/epja/i2013-13040-9
50. Heinz S. Multinucleon transfer reactions - a pathway to new heavy and superheavy nuclei? J Phys Conf Ser (2018) 1014:012005. doi:10.1088/1742-6596/1014/1/012005
51. Adamian GG, Antonenko NV, Diaz-Torres A, Heinz S. How to extend the chart of nuclides? Eur Phys J A (2020) 56:47. doi:10.1140/epja/s10050-020-00046-7
52. Li C, Zhang F, Li J, Zhu L, Tian J, Wang N. Multinucleon transfer in the 136Xe+208Pb reaction. Phys Rev C (2016) 93:014618. doi:10.1103/PhysRevC.93.014618
53. Li C, Wen P, Li J, Zhang G, Li B, Xu X. Production mechanism of new neutron-rich heavy nuclei in the 136Xe+198Pt reaction. Phys Lett B (2018) 776:278–83. doi:10.1016/j.physletb.2017.11.060
54. Li C, Xu X, Li J, Zhang G, Li B, Sokhna CAT. Production of new neutron-rich heavy nuclei with Z = 56-80 in the multinucleon transfer reactions of 136Xe+198Pt. Phys Rev C (2019) 99:024602. doi:10.1103/PhysRevC.99.024602
55. Zhang YX, Wang N, Li QF, Ou L, Tian JL, Liu M. Progress of quantum molecular dynamics model and its applications in heavy ion collisions. Front Phys (2020) 15:54301. doi:10.1007/s11467-020-0961-9
56. Li C, Tian J, Zhang FS. Production mechanism of the neutron-rich nuclei in multinucleon transfer reactions: A reaction time scale analysis in energy dissipation process. Phys Lett B (2020) 809:135697. doi:10.1016/j.physletb.2020.135697
57. Simenel C. Particle transfer reactions with the time-dependent Hartree-Fock theory using a particle number projection technique. Phys Rev Lett (2010) 105:192701. doi:10.1103/PhysRevLett.105.192701
58. Sekizawa K, Yabana K. Time-dependent Hartree-Fock calculations for multinucleon transfer processes in 40,48Ca+124Sn, 40Ca+208Pb, and 58Ni+208Pb reactions. Phys Rev C (2013) 88:014614. doi:10.1103/PhysRevC.88.014614
59. Sekizawa K, Yabana K. Time-dependent Hartree-Fock calculations for multinucleon transfer and quasifission processes in the 64Ni+238U reaction. Phys Rev C (2016) 93:054616. doi:10.1103/PhysRevC.93.054616
60. Sekizawa K. Microscopic description of production cross sections including deexcitation effects. Phys Rev C (2017) 96:014615. doi:10.1103/PhysRevC.96.014615
61. Sekizawa K. Enhanced nucleon transfer in tip collisions of 238U+124Sn. Phys Rev C (2017) 96:041601. doi:10.1103/PhysRevC.96.041601
62. Simenel C, Umar A. Heavy-ion collisions and fission dynamics with the time-dependent Hartree-Fock theory and its extensions. Prog Part Nucl Phys (2018) 103:19–66. doi:10.1016/j.ppnp.2018.07.002
63. Wu Z, Guo L. Microscopic studies of production cross sections in multinucleon transfer reaction 58Ni+124Sn. Phys Rev C (2019) 100:014612. doi:10.1103/PhysRevC.100.014612
64. Jiang X, Wang N. Probing the production mechanism of neutron-rich nuclei in multinucleon transfer reactions. Phys Rev C (2020) 101:014604. doi:10.1103/PhysRevC.101.014604
65. Simenel C, Godbey K, Umar AS. Timescales of quantum equilibration, dissipation and fluctuation in nuclear collisions. Phys Rev Lett (2020) 124:212504. doi:10.1103/PhysRevLett.124.212504
66. Godbey K, Simenel C, Umar AS. Microscopic predictions for the production of neutron-rich nuclei in the reaction 176Yb+176Yb. Phys Rev C (2020) 101:034602. doi:10.1103/PhysRevC.101.034602
67. Sekizawa K. TDHF theory and its extensions for the multinucleon transfer reaction: A mini review. Front Phys (2019) 7:20. doi:10.3389/fphy.2019.00020
68. Sekizawa K, Ayik S. Quantal diffusion approach for multinucleon transfer processes in the 58,64Ni+208Pb reactions: Toward the production of unknown neutron-rich nuclei. Phys Rev C (2020) 102:014620. doi:10.1103/PhysRevC.102.014620
69. Ayik S, Sekizawa K. Kinetic-energy dissipation and fluctuations in strongly damped heavy-ion collisions within the stochastic mean-field approach. Phys Rev C (2020) 102:064619. doi:10.1103/PhysRevC.102.064619
70. Williams E, Sekizawa K, Hinde DJ, Simenel C, Dasgupta M, Carter IP. Exploring zeptosecond quantum equilibration dynamics: From deep-inelastic to fusion-fission outcomes in 58Ni+60Ni reactions. Phys Rev Lett (2018) 120:022501. doi:10.1103/PhysRevLett.120.022501
71. Brink D, Broglia R. Nuclear superfluidity: Pairing in finite systems. Cambridge: Cambridge University Press (2005).
72. Broglia R, Zelevinsky V. Fifty years of nuclear BCS - pairing in finite systems. USA: World Scientific (2013).
73. Potel Aguilar G, Broglia RA. The nuclear cooper pair. The nuclear cooper pair: Structure and reactions. Cambridge: Cambridge University Press (2021). doi:10.1017/9781108919036
74. Oertzen W, Vitturi A. Pairing correlations of nucleons and multi-nucleon transfer between heavy nuclei. Rep Prog Phys (2001) 64:1247–337. doi:10.1088/0034-4885/64/10/202
75. Scamps G, Hagino K. Coupled-channels description of multinucleon transfer and fusion reactions at energies near and far below the Coulomb barrier. Phys Rev C (2015) 92:054614. doi:10.1103/PhysRevC.92.054614
76. Scamps G, Lacroix D. Effect of pairing on one- and two-nucleon transfer below the Coulomb barrier: A time-dependent microscopic description. Phys Rev C (2013) 87:014605. doi:10.1103/PhysRevC.87.014605
77. Scamps G, Sargsyan VV, Adamian GG, Antonenko NV, Lacroix D. Extraction of pure transfer probabilities from experimental transfer and capture data. Phys Rev C (2016) 94:064606. doi:10.1103/PhysRevC.94.064606
78. Broglia RA, Barranco F, Potel G, Vigezzi E. Transient weak links between superconducting nuclei: Coherence length. Nucl Phys News (2021) 31:24–9. doi:10.1080/10619127.2021.1990668
79. Magierski P. The tiniest superfluid circuit in nature. Physics (2021) 14:27. doi:10.1103/physics.14.27
80. Corradi L, Szilner S, Pollarolo G, Fioretto E, Galtarossa F, Mijatović T. Probing nucleon-nucleon correlations in heavy ion transfer reactions using large solid angle magnetic spectrometers. JPS Conf Proc (2020) 32:010043. doi:10.7566/JPSCP.32.010043
81. von Oertzen W, Peter I, Thummerer S, Bohlen HG, Gebauer B, Gerl J. Selection of cold transfer and enhanced neutron-pair transfer in the 206Pb+118Sn reaction. Eur Phys J A (2003) 20:153–6. doi:10.1140/epja/i2002-10342-y
82. Mijatović T, Szilner S, Corradi L, Galtarossa F, Montanari D, Pollarolo G, et al. Nucleon-nucleon correlation studies in heavy-ion transfer reactions. J Phys Conf Ser (2020) 1643:012097. doi:10.1088/1742-6596/1643/1/012097
83. Frauendorf S, Macchiavelli A. Overview of neutron-proton pairing. Prog Part Nucl Phys (2014) 78:24–90. doi:10.1016/j.ppnp.2014.07.001
84. Ayyad Y, Lee J, Tamii A, Lay JA, Macchiavelli AO, Aoi N. Investigating neutron-proton pairing in sd-shell nuclei via (p,3He) and (3He,p) transfer reactions. Phys Rev C (2017) 96:021303. doi:10.1103/PhysRevC.96.021303
85. Cederwall B, Liu X, Aktas O, Ertoprak A, Zhang W, Qi C, et al. Isospin properties of nuclear pair correlations from the level structure of the self-conjugate nucleus 88Ru. Phys Rev Lett (2020) 124:062501. doi:10.1103/PhysRevLett.124.062501
86. Llewellyn RDO, Bentley MA, Wadsworth R, Iwasaki H, Dobaczewski J, de Angelis G, et al. Establishing the maximum collectivity in highly deformed N = Z nuclei. Phys Rev Lett (2020) 124:152501. doi:10.1103/physrevlett.124.152501
87. Lay J, Ayyad Y, Macchiavelli A. Neutron-proton pair transfer reactions and corresponding Weisskopf-type units. Phys Lett B (2022) 824:136789. doi:10.1016/j.physletb.2021.136789
88. Le Crom B, Assié M, Blumenfeld Y, Guillot J, Sagawa H, Suzuki T, et al. Neutron-proton pairing in the N=Z radioactive fp-shell nuclei 56Ni and 52Fe probed by pair transfer. Phys Lett B (2022) 829:137057. doi:10.1016/j.physletb.2022.137057
89. Andrighetto A, Manzolaro M, Corradetti S, Scarpa D, Monetti A, Rossignoli M, et al. Spes: An intense source of neutron-rich radioactive beams at Legnaro. J Phys Conf Ser (2018) 966:012028. doi:10.1088/1742-6596/966/1/012028
90. Borge M. Highlights of the ISOLDE facility and the HIE-ISOLDE project. Nucl Instr Methods Phys Res Section B: Beam Interactions Mater Atoms (2016) 376:408–12. doi:10.1016/j.nimb.2015.12.048
91. Lewitowicz M. The SPIRAL2 project and experiments with high-intensity rare isotope beams. J Phys Conf Ser (2011) 312:052014. doi:10.1088/1742-6596/312/5/052014
92. Sherrill BM. Future opportunities at the facility for rare isotope beams. EPJ Web Conf (2018) 178:01001. doi:10.1051/epjconf/201817801001
93. Tanihata I, Alcorta M, Bandyopadhyay D, Bieri R, Buchmann L, Davids B, et al. Measurement of the two-halo neutron transfer reaction 1H(11Li, 9Li)3H at 3A MeV. Phys Rev Lett (2008) 100:192502. doi:10.1103/PhysRevLett.100.192502
94. Potel G, Barranco F, Vigezzi E, Broglia RA. Evidence for phonon mediated pairing interaction in the halo of the Nucleus 11Li. Phys Rev Lett (2010) 105:172502. doi:10.1103/PhysRevLett.105.172502
Keywords: low-energy heavy-ion reactions, multinucleon transfer reactions, neutron-rich nuclei, nucleon-nucleon correlations, magnetic spectrometers
Citation: Mijatović T (2022) Multinucleon transfer reactions: a mini-review of recent advances. Front. Phys. 10:965198. doi: 10.3389/fphy.2022.965198
Received: 09 June 2022; Accepted: 01 July 2022;
Published: 19 August 2022.
Edited by:
Leandro Gasques, University of São Paulo, BrazilCopyright © 2022 Mijatović. This is an open-access article distributed under the terms of the Creative Commons Attribution License (CC BY). The use, distribution or reproduction in other forums is permitted, provided the original author(s) and the copyright owner(s) are credited and that the original publication in this journal is cited, in accordance with accepted academic practice. No use, distribution or reproduction is permitted which does not comply with these terms.
*Correspondence: Tea Mijatović, dGVhLm1pamF0b3ZpY0BpcmIuaHI=