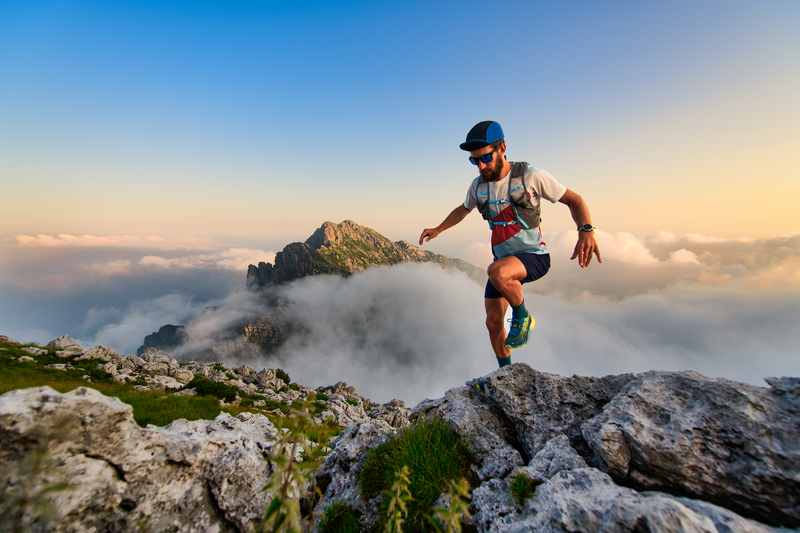
95% of researchers rate our articles as excellent or good
Learn more about the work of our research integrity team to safeguard the quality of each article we publish.
Find out more
MINI REVIEW article
Front. Phys. , 16 September 2022
Sec. Medical Physics and Imaging
Volume 10 - 2022 | https://doi.org/10.3389/fphy.2022.956983
This article is part of the Research Topic Innovative instrumentation and methods for patient-tailored treatment in radiation therapy and theranostics and their roadmap to clinical trials View all 9 articles
Brachytherapy is a well-established treatment option for different cancer types. One treatment option, namely temporary afterloading brachytherapy, utilizes an encapsulated radioactive source, which is guided through implanted applicators to pre-defined dwell positions, to deliver highly conformal and concentrated doses to the target volume. However, treatment errors and uncertainties might still occur. The treatment therefore requires a stringent verification on an individual patient level, particularly in the form of applicator reconstruction. Electromagnetic tracking (EMT) is a possible verification option whose application neither relies on direct line of sight nor does it increase the radiation exposure. However, its systematic clinical application is not yet realized. This review will separate the concept of treatment verification through the use of electromagnetic tracking into four focus groups and categorize the relevant papers within said groups. The four focus groups are geometric assessment, applicator reconstruction, patient-individual quality assurance and adaptive treatment planning. The reviewed papers can be mainly sorted into the focus groups of geometric assessment and applicator reconstruction underlining the potential of EMT as an asset for reconstruction and localization. A smaller group of papers can be associated with patient-individual quality assurance where the detection of implant variations such as swaps within the connector cohort or single catheter shifts take center stage. The final focus group, adaptive treatment planning, is sparsely represented, yet the feasibility of an adaptive treatment course can be derived. This review will close with a discussion of possible improvements and illustrate the path ahead to clinical implementation.
Shortly after the discovery of radioactivity, the newly discovered principle and its effect on biological tissue were investigated, resulting in the development of brachytherapy as the first radiation-based treatment. In contrast to external beam radiation, the radioactive source, nowadays typically Ir-192, is directly brought into the vicinity of the target volume, delivering the prescribed dose in temporary afterloading brachytherapy by resting for pre-defined dwell times at pre-defined dwell positions. One of its most characteristic features is its dose gradient, which declines quickly due to the inverse square law. Brachytherapy can be divided into four dose rate categories (low, medium, high and pulsed) ranging from 2 Gy/h up to 40 Gy/h at the reference location. It is a well-established treatment option for several different cancer sites including prostate, breast and gynecology and has seen constant advances through the development of new applicator and catheter technologies [1].
This review mainly covers high dose rate (HDR) interstitial brachytherapy (iBT), where the radiation source is guided through implanted rigid needles or flexible catheters or adjustable applicators. Due to the steep dose gradient in brachytherapy, small positional changes may result in large changes in the dose distribution. With ever shorter fractionation schemes down to single-session treatments on the rise, even more thorough thought must be given to the highly accurate delivery of the dose. Hence, a precise quality assurance (QA), especially for in-vivo pretreatment verification and implant/applicator reconstruction, is necessary to ensure the good oncological outcome. Capitalizing on this need, mainly two novel techniques have emerged in recent years: in-vivo dosimetry (IVD) and electromagnetic tracking (EMT). While IVD is able to measure the applied dose during treatment delivery by a small-sized dosimeter inserted into the patient, it is limited in other aspects that are covered by EMT [2]. Assessing the 3D geometry of the catheter implant is one of the most important aspects of the QA, but the most common tracking modes – optical and radiation-based tracking – present shortcomings. Optical tracking relies on direct line of sight of the trackable probe and is therefore impossible for implanted applicators [3]. In-vivo dose verification specializes only on the dose distribution with implicit determination of the geometry [4], while 3D imaging reflects reconstruction errors and anatomic variations [5, 6]. Meanwhile, EMT is investigated for implant reconstruction, determination of anatomic variations, and QA of correct treatment application before dose delivery. Still, only a joint application where several verification modes are readily available within the treatment room will eliminate all shortcomings. EMT functions on the principle of induction. Usually, a defined inhomogeneous external magnetic field is produced through a set of coils in the so-called field generator. Typical dimensions of the detection volume are approximately 50 × 50 × 50 cm. The probe sensor is then introduced into said detection volume where a current induced by the field within the probe changes the surrounding field. Measuring the magnetic flux of the external field allows localization of the probe since the physical model of the field is known [7]. This review will showcase the capabilities of EMT in HDR iBT, as well as highlight the current progress in regards of a clinical introduction of EMT.
For this review, a keyword search on PubMed, ScienceOpen, IEEE Xplore and ResearchGate utilizing the keywords “EMT brachytherapy”, “EMT verification”, “treatment verification”, “magnetic tracking” and “tracking brachytherapy” has been used resulting in a paper count of 54 papers after accounting for doubles. Following a selection based on the abstracts of said papers, the count was further reduced to 40 papers. A cross-check if all relevant papers were present was performed by utilizing ConnectedPapers [8] centering on the most cited paper [9] as well as validation through centering on the oldest [10] and the most recently published paper [11].
This review centers on the advances made towards an application of EMT in HDR iBT brachytherapy which can be classified into four focus groups:
1) Geometric assessment
2) Applicator reconstruction
3) Patient-individual quality assurance, i.e. detection of treatment errors and uncertainties
4) Adaptive treatment planning
An overview of the main papers is provided in Supplementary Table S1 which is structured according to these focus groups. Evidently, each level builds upon the former level, increasing in complexity. The reviews by Franz et al. [9] and Sorriento et al. [18] present an overview of the components of an EMT system, the main ones being the field generator and at least one sensor as well as a control unit in order to apply EMT in a medical setting. Figure 1 shows a schematic setup of the EMT components for a breast implant. The EMT sensors are approximately 1 mm long while being thin enough to comfortably fit into 6F catheter tubes and therefore small enough to be attached to medical instruments for intra-body localization as, for instance, in endoscopies, biopsies, or computer-assisted surgery. However, in order to assess the 3D shape of a brachytherapy applicator such as catheters or needles, the EMT sensor is gradually moved through it. In tracking an electromagnetic sensor along a defined shape, any object such as applicators or catheters paths can be traced if dimension and curvature of the object mechanically allow it [31]. This way, EMT provides an easy and inexpensive way to visualize the applicator paths inside a patient without any dose exposure or line-of-sight restrictions. However, in order to derive clinically relevant information from the EMT data, it must be referred and compared to the modalities that also provide a link to patient anatomy, i.e. target volumes and organs at risk. By registering the tracked shape to established imaging modalities such as computed tomography (CT) or (3D) ultrasound (US) as outlined in the review by Bert et al. [2], the link to the target geometry can be established and allows for the detection of variability. Likewise, combining EMT and IVD, both the dose and the position can be linked to form a more complete assessment of the prescribed treatment. While said variability already proves fundamental to quality assurance, it can be even further extended by including (retrospective) dose variation calculations. Finally, an adaptive treatment planning can be achieved by performing live dose calculations based on detected anatomy and implant.
FIGURE 1. Schematic EM setup for a breast implant. The field generator (FG) is mounted above the detection volume. An EM sensor is added to a drive of a modified afterloader and then moved through the implant. A dedicated tracking system records the data from the sensor and the field generator.
General assessment of a 3D geometry forms the base for any analysis, since it aims at tracking of any object. EMT can achieve an overall precision and accuracy of <1 mm and is therefore a viable candidate for geometric assessments [2, 18]. Concerning data acquisition, two main modes are currently deployed. In the first mode, the sensor is moved continuously, while separate dwell positions and dwell times are refined for another mode, which allows for an approximation of the treatment plan. The data produced in the second mode consists of separate point clouds which then form the basis for a reconstruction algorithm. EM-based tracking is easily applicable and reproducible while its overall accuracy and precision mainly depends on the implemented EMT-system (EMTS) and has seen mayor improvements within the last decade. One of the first developments of an CT based EM navigated system for interstitial brachytherapy was implemented by Straßmann et al. in 2000 finding already an improvement on the accuracy of a CT-guided technique by reducing the range of accuracy by one third when utilizing an electromagnetic digitizer [14]. However, influences of nearby (metallic) equipment will influence the performance of the tracking system. As studied by Boutaleb et al. [12] utilizing a polycarbonate phantom, the positional error of EMT is within (2 ± 1) mm and the orientational error is within ±2° both errors taking nearby equipment into consideration. Their findings indicate a necessary for an EM sterile environment in order to achieve the intended precision and accuracy as described by the manufactures of EMT systems.
In 2020, Gomes-Fonseca et al. [32] studied the accuracy and precision of two different field generator types in a controlled phantom study utilizing a scaffold with supports for surgical instruments. They found no noteworthy distinguishment between the two types of field generators, but observed that the tabletop field generator (TTFG) slightly outperforms the planar field generator (PFG) in regards to accuracy with a positional accuracy of 0.72 mm and an angular accuracy of 0.39° (PFG: 0.85 mm & 0.43°), while being outperformed in regards to its precision (TTFG: 0.20 mm & 0.12°, PFG: 0.10 mms.03°). Likewise, only the four-dimensional mechanical ultrasound probe caused noticable distortions, making readings unreliable.
The work of van Heerden et al. [31] studied the residual error of an EMTS by using both cervix and prostate phantoms. They created treatment plans based on CT images with 270/65 dwell positions for the prostate/cervix phantom and found a mean residual error of the interstitial channels of 0.5/0.4 mm, respectively. Comparing the CT and patient bed setups to the distortion free sourrounding, they found slightly larger residual errors still <1 mm.
The groups from Boston, Ma, USA and Québec, Quebec, Canada focused on cervix cancer and found targeting errors of EMT navigation systems <2.9 mm [33] and an average positional uncertainty of 0.2 mm as well as a maximum angular uncertainty of 1.1° [23, 34], which is comparable to the targeting errors in commercial clinical navigation system.
While standalone EMT is a functional method for 3D representation, the registration to other established image modalities such as CT, US or MRI, establishes a link to the patient’s anatomy. Performing a successful registration requires several steps and relies on surrounding landmarks depending on the specific body site. The registration can therefore vary greatly in complexity and might also deploy other means of registration, such as hand-eye calibration. Concerning the choice of reconstruction algorithm of catheter traces, especially for data obtained whilst following individual dwell points, both polynomial fits of degree 3 or 5 and linear, cubic or b-spline interpolations are used and yield similar results [27, 28]. With a successful reconstruction, geometrical variabilities can be studied, which is the current focus of most research in this field. The study of Damato et al. [24] showed a mean registration error of (0.6 ± 0.2) mm for the digitization of catheters utilizing linear interpolation and registration to CT images with 1.25 mm slice thickness, underlining the feasibility of registration for EMT. In comparison to a µCT image (voxel size 89 µm) Poulin et al. reported that their EMT system outperformed conventional CT (voxel size 2 mm) in terms of speed and accuracy [15].
The research group in Royal Oak, Mi, USA studying prostate cancer found that the assistance of EMT during needle implantation results in dosimetric improvements in the urethra V100 dose without EMT (90.96 ± 3.10)% vs with EMT guidance (85.78 ± 7.76)% as well as an overall reduction of implanted needles [29]. They also reported an overall accuracy of the EMT dynamic tracking of (0.9 ± 0.2) mm, emphasizing the necessity for proper calibration of the EMT system in the treatment room [25]. Similarly, Sadjadi et al. observed a reduction in needle tip position estimation error by (52 ± 17)% when tracking the tip and the base of the implanted needles compared to model-based methods [35]. Bharat et al. proposed an extended calibration algorithm achieving mean accuracies of <0.5 mm in a non-distorting environment [17]. Similar registration errors between ultrasound and EMT were reported during the commissioning of a new clinical investigational system in Québec, Canada [19, 36].
Tho et al. also showed in their studies towards an application of EMT for cervix cancer patients the possibility for reconstruction of individual catheters with an identification up to an inter-catheter shift of 1 mm [13, 37] while recommending a sensor speed of <5 cm/s.
Studying iBT of the breast, Janssen et al. registered EM-tracked catheters to CT images finding accuracies of at most (2.0 ± 1.2) mm [38].
To improve the catheter detection rate of EMT in iBT of the prostate, Lungez et al. implemented an advanced nonholonomic extended Kalman filter resulting in a path reconstruction accuracy of 1.9 mm and a precision of 0.8 mm [39]. Götz et al. developed a complete analysis routine by implementing a coherent processing chain consisting of a dedicated particle filter for improved geometric assessment, followed by a noise suppression algorithm and finally a signal decomposition [16, 26, 27, 40–42].
The main aspect of quality assurance is the prevention of errors and identification of uncertainties [21]. In the field of quality assurance an error is defined as a preventable occurrence such as human mistakes or machine failures, while uncertainties can only be reducible but never fully preventable and are most common as source strength uncertainty, implant geometry variations or limitations from the dose calculation engine.
Human mistakes are the most common error during treatment delivery and treatment planning, emphasizing the importance of studies to identify and avoid human mistakes. This results in a further classification of human mistakes. Examples include wrong catheter length, catheter reconstruction errors such as partial catheter shifts, misidentified first dwell positions or an inverted catheter direction.
Although EMT alone cannot be used for dose assessment, it could still play an important role for error detection [13, 22]. Since it allows high frequency position measurements, an EMT sensor might be coupled with the source cable allowing for precise afterloader source positioning as well as applicator tracing. Alternatively, mounting the sensor to the check cable eliminates the high dose rates close to the EMT sensor and, potentially even more important, identification of errors prior delivery. This setup would also allow for detection of general afterloader malfunctions. However, it would not be able to detect decoupling of the source from the cable, but this task is of minor importance since afterloading devices and dosimeter in the treatment room would catch this error. Since the implant geometry is patient specific, EMT allows for patient identification and can prevent a mix-up of patients. Comparison of the EMT measurement to the reference implant geometry would allow for detection of interfraction movement of applicator parts, such as individual catheter movements, also including the detection of mixing and interchange of catheter traces. Extending the measurement by introducing a fiducial coordinate system through additional sensors positioned on the skin of the patient would also show relative motion of the implant site compared to the patient’s rest frame allowing for breathing motion correction of the EMT data.
The research group in Erlangen, Germany focuses on EMT in multi-catheter iBT of the breast [20, 22, 27, 28, 30, 43, 44]. An ongoing patient study in Erlangen covers several topics, including breathing motion compensation, interfractional variations such as positioning of the patient. They showed clinical feasibility for assessment of interfractional variations by reporting deviations in dwell positions of (2.5 ± 1.5) mm within the 5-days treatment schedule [44]. By introducing a dedicated algorithm for breathing compensation and defining criterions for various geometric deviations, a fundament for standalone quality assurance has been built. A study with 54 patients in which breathing motion compensation was archived by either determination of the center of mass of three skin sensors or a resting sensor within single catheters showed, that the mean sensor displacement per dwell position after breathing motion correction is below the precision of the EMT system (<1 mm) [43]. Studying the error detection with a cohort of 10 patients and additional simulations in phantoms, detection rates of 100% for catheter swaps and 97% for shifts >1.1 mm were reported [22].
Time sensitive optimization in the clinical workflow is one of the main goals of clinically applicable research. The detection of uncertainties or errors alone does benefit a patient significantly, but by also reducing said uncertainties and adapting the existing plan, the patient specific adaptive treatment planning results in individualized and therefore potentially more successful treatments. In order to perform adaptive treatment planning, current representations of the patient have to be available and fast planning algorithms need to be implemented. Beaulieu et al. were able to implement a 3DUS-based HDR brachytherapy procedure for prostate cancer, which has been tested on a phantom resulting in a total workflow time of approximately 15 min and is expected to increase for patients [45]. Even though their approach targeted at single fraction high dose HDR treatments, the individual components of the workflow (reconstruction, quick dose calculation, registration of EMT and imaging data) are also needed for an adaptive workflow which can thus be rendered feasible.
EMT as an adjuvant QA method in brachytherapy proves feasible in regards to necessary equipment, total time gain and validity. Ongoing studies show reliable implant reconstruction and geometric assessment possibilities through the use of EMT and further the general understanding of this method in a clinical context. While an actual implementation of EMT in adaptive treatment planning has yet to be achieved, the fundamentals for an in-time analysis are well studied.
CB and BS determined the scope of the review. BS wrote the first draft. All authors contributed to manuscript revision, read, and approved the submitted version.
The research in regards to electromagnetic tracking in interstitial brachytherapy at the Universitätsklinikum Erlangen is funded by an unrestricted research grant from Elektra. We acknowledge financial support by Deutsche Forschungsgemeinschaft and Friedrich-Alexander-Universität Erlangen-Nürnberg within the funding program “Open Access Publication Funding”.
The authors declare that the research was conducted in the absence of any commercial or financial relationships that could be construed as a potential conflict of interest.
All claims expressed in this article are solely those of the authors and do not necessarily represent those of their affiliated organizations, or those of the publisher, the editors and the reviewers. Any product that may be evaluated in this article, or claim that may be made by its manufacturer, is not guaranteed or endorsed by the publisher.
The Supplementary Material for this article can be found online at: https://www.frontiersin.org/articles/10.3389/fphy.2022.956983/full#supplementary-material
1. Lim YK, Kim D. Brachytherapy: A comprehensive review. Prog Med Phys (2021) 32(2):25–39. doi:10.14316/pmp.2021.32.2.25 |
2. Bert C, Kellermeier M, Tanderup K. Electromagnetic tracking for treatment verification in interstitial brachytherapy. J Contemp Brachytherapy (2016) 8(5):448–53. doi:10.5114/jcb.2016.63356 | |
3. Weersink R. SP-0033: Optical and tracking technologies for navigation in brachytherapy. Radiother Oncol (2017) 123:S11. doi:10.1016/s0167-8140(17)30477-2 |
4. Jaselskė E, Adliene D, Rudzianskas V, Urbonavicius BG, Inciura A. In vivo dose verification method in catheter based high dose rate brachytherapy. Physica Med (2017) 44:1–10. doi:10.1016/j.ejmp.2017.11.003 |
5. Westendorp H, Hoekstra CJ, Immerzeel JJ, van de Pol SM, Niel CG, Kattevilder RA, et al. Cone-beam CT-based adaptive planning improves permanent prostate brachytherapy dosimetry: An analysis of 1266 patients. Med Phys (2017) 44(4):1257–67. doi:10.1002/mp.12156 | |
6. Zoberi JE, Garcia-Ramirez J, Hedrick S, Rodriguez V, Bertelsman CG, Mackey S, et al. MRI-based treatment planning and dose delivery verification for intraocular melanoma brachytherapy. Brachytherapy (2018) 17(1):31–9. doi:10.1016/j.brachy.2017.07.011 | |
7. Cunha JAM, Flynn R, Belanger C, Callaghan C, Kim Y, Jia X, et al. Brachytherapy future directions. Semin Radiat Oncol (2020) 30(1):94–106. doi:10.1016/j.semradonc.2019.09.001 | |
8. Eitan AT, Smolyansky E, Harpaz IK, Perets S. Connected papers. 2021; Available from: https://www.connectedpapers.com/. (Accessed April 02, 2022)
9. Franz AM, Haidegger T, Birkfellner W, Cleary K, Peters TM, Maier-Hein L. Electromagnetic tracking in medicine—a review of technology, validation, and applications. IEEE Trans Med Imaging (2014) 33(8):1702–25. doi:10.1109/tmi.2014.2321777 | |
10. Trejos AL, Lin AW, Pytel MP, Patel RV, Malthaner RA. Robot-assisted minimally invasive lung brachytherapy. Int J Med Robotics Comput Assist Surg (2007) 3:41–51. doi:10.1002/rcs.126 | |
11. Grajales D, Kadoury S, Shams R, Barkati M, Delouya G, Beliveau-Nadeau D, et al. Performance of an integrated multimodality image guidance and dose-planning system supporting tumor-targeted HDR brachytherapy for prostate cancer. Radiother Oncol (2021) 166:154–61. doi:10.1016/j.radonc.2021.11.026 | |
12. Boutaleb S, Racine E, Fillion O, Bonillas A, Hautvast G, Binnekamp D, et al. Performance and suitability assessment of a real-time 3D electromagnetic needle tracking system for interstitial brachytherapy. J Contemp Brachytherapy (2015) 7(4):280–9. doi:10.5114/jcb.2015.54062 | |
13. Tho D, Lavallée MC, Beaulieu L. Performance of an enhanced afterloader with electromagnetic tracking capabilities for channel reconstruction and error detection. Med Phys (2021) 48(8):4402–10. doi:10.1002/mp.14877 | |
14. Straßmann G, Kolotas C, Heyd R, Walter S, Baltas D, Martin T, et al. Navigation system for interstitial brachytherapy. Radiother Oncol (2000) 56(1):49–57. doi:10.1016/s0167-8140(00)00209-7 | |
15. Poulin E, Racine E, Binnekamp D, Beaulieu L. Fast, automatic, and accurate catheter reconstruction in HDR brachytherapy using an electromagnetic 3D tracking system. Med Phys (2015) 42(3):1227–32. doi:10.1118/1.4908011 | |
16. Götz TI, Wankerl H, Tome AM, Meyer‐Baese A, Bert C, Hensel B, et al. Technical note: A comparison of point set registration methods for electromagnetic tracking. Med Phys (2019) 46(5):2025–30. doi:10.1002/mp.13443 | |
17. Bharat S, Kung C, Dehghan E, Ravi A, Venugopal N, Bonillas A, et al. Electromagnetic tracking for catheter reconstruction in ultrasound-guided high-dose-rate brachytherapy of the prostate. Brachytherapy (2014) 13(6):640–50. doi:10.1016/j.brachy.2014.05.012 | |
18. Sorriento A, Porfido MB, Mazzoleni S, Calvosa G, Tenucci M, Ciuti G, et al. Optical and electromagnetic tracking systems for biomedical applications: A critical review on potentialities and limitations. IEEE Rev Biomed Eng (2020) 13:212–32. doi:10.1109/rbme.2019.2939091 | |
19. Dehghan E, Bharat S, Kung C, Bonillas A, Beaulieu L, Pouliot J, et al. EM-enhanced US-based seed detection for prostate brachytherapy. Med Phys (2018) 45(6):2357–68. doi:10.1002/mp.12894 | |
20. Kellermeier M, Herbolzheimer J, Kreppner S, Lotter M, Strnad V, Bert C. Electromagnetic tracking (EMT) technology for improved treatment quality assurance in interstitial brachytherapy. J Appl Clin Med Phys (2017) 18(1):211–22. doi:10.1002/acm2.12021 | |
21. Kirisits C, Rivard MJ, Baltas D, Ballester F, De Brabandere M, van der Laarse R, et al. Review of clinical brachytherapy uncertainties: Analysis guidelines of GEC-ESTRO and the AAPM. Radiother Oncol (2014) 110(1):199–212. doi:10.1016/j.radonc.2013.11.002 | |
22. Masitho S, Kallis K, Strnad V, Fietkau R, Bert C. Error detection using an electromagnetic tracking system in multi-catheter breast interstitial brachytherapy. Phys Med Biol (2019) 64(20):205018. doi:10.1088/1361-6560/ab4336 | |
23. Tho D, Beaulieu L. Technical Note: Identification of an optimal electromagnetic sensor for in vivo electromagnetic-tracked scintillation dosimeter for HDR brachytherapy. Med Phys (2019) 46(5):2031–6. doi:10.1002/mp.13508 | |
24. Damato AL, Viswanathan AN, Don SM, Hansen JL, Cormack RA. A system to use electromagnetic tracking for the quality assurance of brachytherapy catheter digitization. Med Phys (2014) 41(10):101702. doi:10.1118/1.4894710 | |
25. Zhou J, Sebastian E, Mangona V, Yan D. Real-time catheter tracking for high-dose-rate prostate brachytherapy using an electromagnetic 3D-guidance device: A preliminary performance study. Med Phys (2013) 40(2):021716. doi:10.1118/1.4788641 | |
26. Götz TI, Lahmer G, Strnad V, Bert C, Hensel B, Tome AM, et al. A tool to automatically analyze electromagnetic tracking data from high dose rate brachytherapy of breast cancer patients. PLoS One (2017) 12(9):e0183608. doi:10.1371/journal.pone.0183608 | |
27. Götz TI, Tome AM, Hensel B, Bert CH, Lang EW. Electromagnetic tracking in high dose rate brachytherapy - a composite analysis model. Adv Appl Sci Res (2018) 9.
28. Kallis K, Kreppner S, Lotter M, Fietkau R, Strnad V, Bert C. Introduction of a hybrid treatment delivery system used for quality assurance in multi-catheter interstitial brachytherapy. Phys Med Biol (2018) 63(9):095008. doi:10.1088/1361-6560/aabb5a | |
29. Zhou J, Sebastian E, Yan D. SU-D-213AB-01: Dosimetry improvement and needle number reduction in prostate brachytherapy using electromagnetically guided needle placement. Med Phys (2012) 39(6-3):3611. doi:10.1118/1.4734661 | |
30. Kallis K, Abu-Hossin N, Kreppner S, Lotter M, Strnad V, Fietkau R, et al. Estimation of inter-fractional variations in interstitial multi-catheter breast brachytherapy using a hybrid treatment delivery system. Radiother Oncol (2019) 141:312–20. doi:10.1016/j.radonc.2019.08.012 | |
31. van Heerden L, Schiphof-Godart J, Christianen M, Mens JW, Franckena M, Maenhout M, et al. Accuracy of dwell position detection with a combined electromagnetic tracking brachytherapy system for treatment verification in pelvic brachytherapy. Radiother Oncol (2020) 154:249–54. doi:10.1016/j.radonc.2020.09.061 | |
32. Gomes-Fonseca J, Veloso F, Queiros S, Morais P, Pinho ACM, Fonseca JC, et al. Technical Note: Assessment of electromagnetic tracking systems in a surgical environment using ultrasonography and ureteroscopy instruments for percutaneous renal access. Med Phys (2020) 47(1):19–26. doi:10.1002/mp.13879 | |
33. Mehrtash A, Damato A, Pernelle G, Barber L, Farhat N, Viswanathan A, et al. EM-navigated catheter placement for gynecologic brachytherapy: An accuracy study. Proc SPIE Int Soc Opt Eng (2014) 9036:90361f. doi:10.1117/12.2044381 | |
34. Tho D, Beaulieu L. Technical note: A novel electromagnetic-tracked scintillation dosimeter for accurate in vivo dosimetry in HDR brachytherapy. Ithaca, New York, United States: arXiv Cornell University (2018).
35. Sadjadi H, Hashtrudi-Zaad K, Fichtinger G. Needle deflection estimation: Prostate brachytherapy phantom experiments. Int J Comput Assist Radiol Surg (2014) 9(6):921–9. doi:10.1007/s11548-014-0985-0 | |
36. Lavallée MC, Cantin A, Moneger F, Lefebvre M, Foster W, Vigneault E, et al. Commissioning of an intra-operative US guided prostate HDR system integrating an EM tracking technology. Brachytherapy (2021) 20(6):1296–304. doi:10.1016/j.brachy.2021.05.163 | |
37. Tho D, Racine E, Easton H, Song WY, Beaulieu L. Technical note: On EM reconstruction of a multi channel shielded applicator for cervical cancer brachytherapy: A feasibility study. Med Phys (2018) 45(4):1673–6. doi:10.1002/mp.12789 | |
38. Janssen NNY, Brastianos H, Akingbade A, Olding T, Vaughan T, Ungi T, et al. Electromagnetic (EM) catheter path tracking in ultrasound-guided brachytherapy of the breast. Int J Comput Assist Radiol Surg (2020) 15(10):1645–52. doi:10.1007/s11548-020-02233-9 | |
39. Lugez E, Sadjadi H, Joshi CP, Akl SG, Fichtinger G. Improved electromagnetic tracking for catheter path reconstruction with application in high-dose-rate brachytherapy. Int J Comput Assist Radiol Surg (2017) 12(4):681–9. doi:10.1007/s11548-017-1534-4 | |
40. Götz TI, Ermer M, Salas-Gonzalez D, Kellermeier M, Strnad V, Bert C, et al. On the use of multi-dimensional scaling and electromagnetic tracking in high dose rate brachytherapy. Phys Med Biol (2017) 62(20):7959–80. doi:10.1088/1361-6560/aa8944 | |
41. Götz TI, Herzberger F, Tome AM, Hensel B, Lang EW. Emtlab: A toolbox for the analysis of electromagnetic tracking data in brachytherapy. Adv Appl Sci Res (2017).
42. Götz TI, Lahmer G, Brandt T, Kallis K, Strnad V, Bert C, et al. On the use of particle filters for electromagnetic tracking in high dose rate brachytherapy. Phys Med Biol (2017) 62(19):7617–40. doi:10.1088/1361-6560/aa8591 | |
43. Dürrbeck C, Gulde S, Abu-Hossin N, Fietkau R, Strnad V, Bert C. Influence and compensation of patient motion in electromagnetic tracking based quality assurance in interstitial brachytherapy of the breast. Med Phys (2022) 49(4):2652–62. doi:10.1002/mp.15517 | |
44. Kellermeier M, Fietkau R, Strnad V, Bert C. Assessment of the implant geometry in fractionated interstitial HDR breast brachytherapy using an electromagnetic tracking system. Brachytherapy (2018) 17(1):94–102. doi:10.1016/j.brachy.2017.10.007 | |
Keywords: brachytherapy, electromagnetic tracking, in-vivo verification, treatment uncertainties, quality assurance
Citation: Sauer BC, Dürrbeck C and Bert C (2022) Electromagnetic tracking in interstitial brachytherapy: A systematic review. Front. Phys. 10:956983. doi: 10.3389/fphy.2022.956983
Received: 30 May 2022; Accepted: 01 September 2022;
Published: 16 September 2022.
Edited by:
Francesco Pennazio, National Institute of Nuclear Physics of Turin, ItalyReviewed by:
Yusung Kim, The University of Iowa, United StatesCopyright © 2022 Sauer, Dürrbeck and Bert. This is an open-access article distributed under the terms of the Creative Commons Attribution License (CC BY). The use, distribution or reproduction in other forums is permitted, provided the original author(s) and the copyright owner(s) are credited and that the original publication in this journal is cited, in accordance with accepted academic practice. No use, distribution or reproduction is permitted which does not comply with these terms.
*Correspondence: Birte Christina Sauer, YmlydGUuc2F1ZXJAdWstZXJsYW5nZW4uZGU=
Disclaimer: All claims expressed in this article are solely those of the authors and do not necessarily represent those of their affiliated organizations, or those of the publisher, the editors and the reviewers. Any product that may be evaluated in this article or claim that may be made by its manufacturer is not guaranteed or endorsed by the publisher.
Research integrity at Frontiers
Learn more about the work of our research integrity team to safeguard the quality of each article we publish.