- 1Departamento de Física Aplicada I, Universidad de Sevilla, Seville, Spain
- 2Condensed Matter Physics Center (IFIMAC), Universidad Autónoma de Madrid, Madrid, Spain
- 3Departamento de Física Teórica de La Materia Condensada, Universidad Autónoma de Madrid, Madrid, Spain
Azurin proteins are the workhorse of protein electronics. This is a branch of biomolecular electronics, a recent research field which investigates electronics based on biomolecules such as proteins, peptides, amino acids, bacterial nanowires or DNA. In general, the possibility of including biosystems in solid-state junctions has opened the way to the development of novel electrical devices, and proteins have attracted enormous attention thanks to their many interesting properties. In the particular case of metal-azurin-metal junctions, experimental measurements have revealed extremely efficient electron transport over large distances, showing conductance values which are higher than certain conjugated molecules of similar lengths. Moreover, the electrical current has often been found to be temperature-independent, which has been used as an evidence of coherent transport or quantum tunneling. Interesting effects have been observed, moreover, upon insertion of single amino-acid mutations. In spite of a huge amount of work, the exact mechanism for the charge flow through these systems is still under debate. In this review, we will revise the recent advances made in the electron-transport measurements of azurin-based junctions as well as the corresponding theoretical modelling. We will discuss the interpretation of the currently-available experimental results as well as the open issues which still remain to be clarified.
1 Introduction
The blue-copper azurin has been one of the workhorses for the field of protein-based electronics, which is one of the most recent branches developed out of molecular electronics [1–3]. Research on this subject aims at either exploring the use of biosystems of various kinds (such as proteins, amino acids, peptides, DNA and bacterial nanowires) in electrical devices or at mimicking their conductivity mechanism in bio-inspired electrical components [4]. Amongst the plethora of biosystems available in nature, proteins have attracted remarkable interest because of a large variety of properties which make them suitable for being incorporated into a multifunctional devices. These includes their capability of catalyzing a large number of reactions; their properties in terms of chemical recognition and selectivity; their mechanical and optoelectronic properties; their biocompatibility. The use of proteins has indeed been proposed for sensors, electrical noses, solar cells, field-effect transistors and flexible implants [5–7]. One of the traits which make proteins promising candidates for active components in electrical devices is their extremely efficient electron-transport properties over large distances [3,8]. This is indeed the case for azurins, which are a type of redox metalloproteins involved in the denitrification processes, whose function is related to electron shuttling between cytochrome enzymes in the respiratory chain [9,10].
Extensive characterization on blue copper azurins has been carried out over the years by means of multiple techniques which include X-ray diffraction [11,12], electron paramagnetic resonance [13,14] or nuclear magnetic resonance [15,16]. The work by Solomon’s group was very important to unveil the electronic structure of the Cu site in azurin and related blue copper proteins using a combination of spectroscopic techniques and quantum calculations [9,17–21]. In azurins the Cu center is coordinated by two histidine nitrogen atoms and a cysteine thiolate group in a trigonal planar structure (Figure 1A). The first coordination sphere is completed by two axial ligands: a methionine thioether group and a backbone carbonyl oxygen atom of a glycine residue. Overall, the Cu complex embedded in the azurin proteins displays a distorted pentacoordinated trigonal bipyramidal structure [12,14].
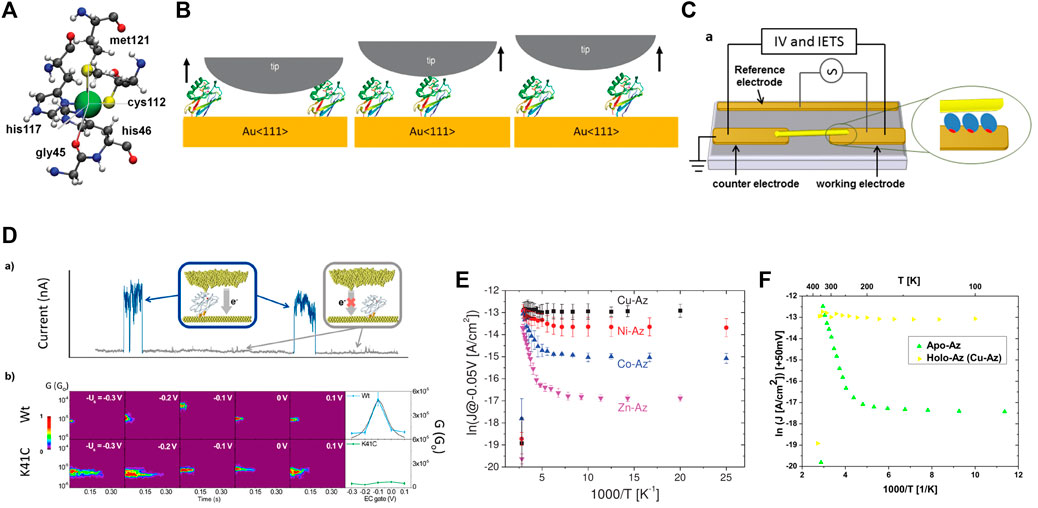
FIGURE 1. (A) First coordination sphere of the Cu complex [56]; (B) Schemes of a single-azurin junction formation by the STM-BJ approach [24]; (C) Scheme of the Azurin junction fabricated by trapping nanowires by an ac electric field [26]; (D) Representative “blinks” (blue traces) identified in the transients of the current flowing between the two electrodes at a constant distance (upper panel) and Semilog 2D-blinking maps for the wild type blue-copper azurin (Wt) and K41C mutant at different EC gate potentials (lower panel) [38]; (E) ln(J) versus 1,000/T of Cu-Az, Ni-Az, Co-Az, and Zn-Az at −50 mV bias [42]; (F) Experimental ln(J) versus 1,000/T of Apo- and Holo-Az junction at +50 mV bias [41].
In the last decade, the electron transport through redox copper proteins has been analyzed extensively [3,22–24]. Interest in this particular type of proteins stems from the unique interplay between optical and vibronic properties with electron transfer in these systems as well as their biorecognition capabilities [8,25–29]. In the following sections, we will review what has been studied and understood about the electron transport through azurins incorporated in solid-state junctions (i.e., electrode-azurin-electrode juctions). We will analyze the most recent advances from both an experimental and theoretical perspective. In particular, we will focus on the efforts made by the community to understand the charge transport mechanism and to which extent azurin proteins can be considered promising candidates for molecular bioelectronic applications.
The review is organized as follow: in section 2, we will review the most recent experimental conductance measurements carried out on azurin proteins; in section 3, we will review attempts made in the field to interpret the experimental results by use of phenomenological models; in section 4, we will discuss studies carried out by large-scale (mainly ab-initio) calculations. Finally, in section 5 we will draw some conclusions to highlight what the main controversies are and what still needs further investigation. Note that, within each section, results and conclusions from the literature will not be presented in chronological order, but rather sorted into paragraphs devoted to discussing specific issues.
2 Electron-Transport Measurements
Several electron-transport measurements have been carried out on the blue-copper azurin over the years. The presence of native cysteins makes it possible to bind this protein to gold electrodes in a well-defined orientation. It was even suggested that inserting them at different locations could actually allow studying this system along many orientations [23]. In Ref. [24], STM (scanning tunneling microscopy) break-junction measurements on single-azurin junctions between a Au substrate electrode and an electrochemical scanning tunneling microscopy (STM) probe under bipotentiostatic control were reported. In this configuration, the tip is brought into contact with the protein-support gold surface followed by a fast pulling of the protein junction (Figure 1B). The measurements showed a transistor-like behaviour. The maximum conductance value was found to be (2.1 ± 0.7) × 10−5G0, with a 20-fold on/off ratio in the conductance between zero and high-electrochemical gate voltages. The results could be fitted to a two-step electron transfer model. The formation of a single-azurin junctions in a very similar experimental set up (in an electrochemical environment) was then also studied by keeping the STM tip at a fixed distance from the surface [8,24]. In this configuration, the contact between the STM tip and the protein is formed thanks to thermal fluctuations of the molecule which induce its spontaneous attachment or detachment to/from the tip. When the contact is established, sudden “blinks” are recorded in the current vs. time trace. These measurements showed evidence of switching events in spontaneously formed single-wire protein junctions. Moreover, the statistical analysis of the results seemed to confirm that the switching events at low bias voltages were related to the electrochemical gate potential of the system according to the redox behavior of azurin. Indeed, measurements on the Zn-azurin (a non-redox protein) within the same experimental set-up had showed no particular electrochemical-gate dependence [23,24]. Transition voltage spectroscopy was also proposed as a useful tool to study this system [30], showing that the transition voltage is strongly regulated by the electronic coupling of the protein with the STM probe. Results from STM imaging of azurin on gold can be found in Ref. [31].
The conductance via the blue copper azurin was also measured via conducting probe atomic force microscopy (CP-AFM), typically on self-assembled monolayers [31–35]. In this set up, the protein is trapped between an AFM conductive probe and a metallic substrate are used. The current flowing via application of a voltage bias is then recorded, while the feedback loop controls the pressure exercised by the probe. This makes it possible to measure topography, lateral forces, and current images simultaneously. An important output of these kinds of experiments were the observations on the temperature dependence of the conductance. In Ref. [32], at low forces the transport through azurin monolayer was found to be temperature independent, whereas at higher temperature (
An important detail which was found to affect the transport properties of this protein is the coupling to the electrodes. In Ref. [28,36], it was shown that modifying the coupling on one side of the junction can convert the transport mechanism from off-resonant to resonant without need of an external gate voltage. This was done within an experimental setup in which the top electrode was a gold nanowire which becomes connected to the protein upon landing on it (Figure 1C). A mercapto-propionic acid (MPA) group was bound covalently to the gold nanowire via an S atom and various types of chemical modifications allowed modification of the coupling. The importance of the contact at the protein-metal interface has been acknowledged also for other types of proteins [37]. The effects of inserting mutation was also explored in Ref. [38], where experiments revealed that inserting mutations in the blue-copper azurin can change the conductance mechanism drastically, changing the gate-dependent conductance curve from a Gaussian shape to a rather flat behaviour (Figure 1D). In Ref. [39] a 10-fold increase in switching of conductance was found for the N42C mutant, obtained by replacing the two native disulfide bridge-forming cysteine residues (3 and 26) by alanines, and the native asparagine residue 42 by a cysteine. This type of mutation affects the orientation of the protein within the junction and increases the coupling, causing higher current values. A complete discussion about the importance of having control over the protein-electrode interface can be found in a recent review reported in Ref. [40].
3 Interpretation of Experimental Results by Phenomenological Models
The results reported above suggest that the exact nature of the electron transport through azurins is unknown and various possibilities should be taken into account. Indeed, over the years, many suggestions have been brought forward to try understanding the mechanism of transport. For instance, in Ref. [24,38], two-step tunneling models were considered, describing an incoherent type of process that involves partial relaxation in the Cu ion. There, the current was expressed in terms of reorganization energy, bias voltage, overpotential and model parameters describing the shift of the effective electrode potential at the redox center with the voltage and the overpotential.
Particular attention must be paid to a theoretical work by [41], in which various simple models were developed to try understanding the results of two experimental studies on self-assembled azurin monolayer carried out in different conditions. The first experimental study concerned measurements reported in Ref. [42], which employed Si-oxide substrate-Azurin-Au junctions or Hg lift-on-float-on (LOFO) heterojunctions. There, the voltage and temperature dependencies of Holo-Azurin (with Cu as the redox site) and Apo-Azurin (with Cu removed) had been compared, as well as the temperature dependencies of other metal-substituted azurins (Ni, Co., and Zn) (Figure 1E). The second experimental study was instead that reported in Ref. [26], which involved Au microelectrode-Azurin-Au micro-electrode heterojunctions and measurements of the current-voltage and current-temperature dependencies of holo-azurin. In both types of experiments, the current through the holo-azurin had been found to be approximately temperature-independent, but its bias voltage dependence of the holo-Az current in the two experiments differed considerably. In particular, an interesting observation in Ref. [42] concerned the apo-azurin which, in contrast with the temperature-independent holo-azurin, had shown activationless behavior at lower temperatures (T
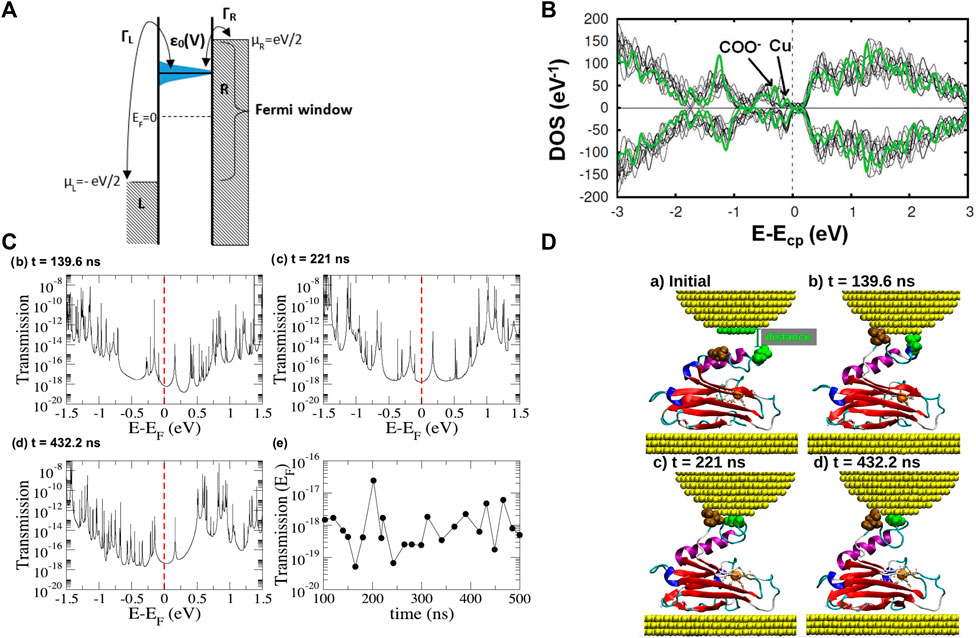
FIGURE 2. (A) Schematic representation of the Landauer tunneling model [41]; (B) Spin resolved total density of the states of the azurin protein [56]; (C) Transmission as a function of energy for three selected Au-azurin-Au junctions obtained from molecular dynamics simulations and transmission at the Fermi energy for 23 representative geometries in the simulated blinking process [58]; (D) geometries corresponding to the transmission curves displayed in panel (C).
4 Large-Scale Computational Simulations
The remarkable conductance measured in the experiments for the blue copper azurin is very surprising taking into account the low tunneling conductance which was computed for junctions based on single amino acids or single peptides [44–46], as well as its size (with a diameter of approximately 2.5 nm). This has inspired ab-initio-based calculations devoted to unraveling this puzzle. Until recently, most computational studies had either employed isolated partial fragments of the protein [47–51] or made use of quantum mechanics/molecular mechanics methods [52,53] which were not fully ab-initio. The study of the single Cu site or other fragment of the protein is, indeed, still currently fundamental for understanding many properties [54,55]. Nevertheless, an analysis of the electronic configuration of proteins when contacted to electrodes should not exclude any part of its physical structure. This is because the electron-transport properties through such hybrid structures are determined by a delicate interplay between the protein orbitals and the coupling to the leads, as it was mentioned in the previous sections. Unfortunately, studies of the whole protein structure had been hindered by computational limitations until very recently. Nevertheless, the current advances in computing performance have now made it possible to overcome this barrier. Recently, ab-initio (DFT) calculations have elucidated the electronic structure of the entire protein [56]. It was found that close to the Cu d levels are many orbitals localized on oxygen atoms which belong to carboxyl groups located all over the peripheral region of the protein (Figure 2B). This was later confirmed by an ab-initio study of the whole metal-azurin-metal junction [57]. In the same work, molecular dynamics simulations also reproduced the evolution of the typical junction created via the blinking technique described in section 1 (Figure 2D). It was observed that the protein-metal contact is created upon separation of the α helix from the β barrel. Interestingly, the DFT calculations showed that such a significant mechanical deformation does not affect the electronic structure of the Cu ion and of its first coordination sphere. Differences were, instead, observed in residues located either close to the tip or to the substrate or belonging to the intermediate region in which the α-helix is separated from the β-barrel. Later, electron-transport calculations based on Green’s function techniques, reproducing a coherent-transport mechanism, were carried out for geometries extracted from selected frames of these simulations [58]. The results there reported revealed that the tunneling conductance of those junctions would be extremely low (between 10–19 and 10−8G0, see Figure 2C). This is due to the extreme low coupling of the Cu ion with the leads. Subsequent calculations [59] showed that similar results are obtained by replacing the Cu ion with other metals (Co., Ni and Zn). This can be ascribed to the fact that the main transmission background is provided by mostly glutamate and aspartate residues located on the common peripheral area, whereas the metallic ions give rise to very sharp resonances (originated, again, by their low coupling with the metal electrodes). Consequently, a striking similarity was observed between the conductance values calculated for the four proteins, in disagreement with the experimental results discussed in section 3 [42]. Overall, these theoretical results cast doubts about whether the dominant role in the electron transport is played by the only metallic ion or whether, instead, other moieties provide a relevant contribution. This would be in line with the conclusions drawn in other recent experiments carried out on proteins [60] and protoporphyrins [61]. Furthermore, the low conductance values computed suggest that probably the transport mechanism involved in most of the experiments described in section 2 and section 3 is not fully coherent. It is worth mentioning that a low tunneling conductance was calculated by Green’s function technique also for other kinds of proteins [62]. The results discussed above indicate that different theoretical models must be used to calculate the current flow in azurin-based junctions, probably by taking into account incoherent components.
The quest for a theoretical model which describes all the complex features of the transport through these kinds of systems is still actually underway for other types of proteins as well (see, for instance, the theoretical models applied to the study of the CTPR8 protein in Ref. [63] or Ref. myoglobin [64]). It is also worth mentioning that, in the context of incoherent transport through proteins, a crucial quantity is the reorganization energy. This quantity is the energy required to relax the structure of the protein and the surrounding solvent upon electron transfer. Thus it is equivalent to the energy difference between the product state in its equilibrium geometry and in the equilibrium geometry of the reactant state, or vice versa [65]. In the case of blue-copper proteins, the experimental value obtain for this energy is approximately 0.7 eV (see Ref. [66] and references therein), which is lower than in other metalloproteins. High reduction potential and low electron-transfer reorganization energy of azurin have been ascribed to a constrained inner-sphere copper geometry (see Ref. [67] and references therein). In Ref. [66], by means of a QM/MM study it was found that, in the case of azurin, the values of reorganization energy do not change when switching from dry to aqueous solution. Moreover, no significant reduction was observed when contacting the protein to gold surfaces. These conclusions implied that a thermally-activate transport would be required.
In addition, the role played by mutations is currently still under investigation. Besides the effects on the protein-electrode coupling described in the previous sections, in Ref. [68] it was shown that single amino-acid mutations can induce quenching of protein vibrations, ultimately resulting in its overall stiffening. What the effect of this is on the electron-transport still needs to be clarified.
5 Conclusion
The nature of the exact mechanism of the electron transport through azurin-based junctions is still far from being understood. The two main controversies concern what the exact role played by the metal ion is and what kind of transport (coherent vs. incoherent) takes place in the system. While experimental studies from different groups have suggested either fully-coherent transport or 2-step tunneling mechanism, theoretical studies have already ruled out the possibility of fully-coherent transport and suggested the need of considering other types of transport. Hence, this puzzle still needs to be unraveled and further investigation is needed.
Author Contributions
CR-M and LZ wrote the manuscript, with input from all the authors.
Conflict of Interest
The authors declare that the research was conducted in the absence of any commercial or financial relationships that could be construed as a potential conflict of interest.
Publisher’s Note
All claims expressed in this article are solely those of the authors and do not necessarily represent those of their affiliated organizations, or those of the publisher, the editors and the reviewers. Any product that may be evaluated in this article, or claim that may be made by its manufacturer, is not guaranteed or endorsed by the publisher.
Acknowledgments
The authors thankfully acknowledge the computer resources, technical expertise, and assistance provided by the Red Española de Supercomputación (RES) at Cesga. CR-M acknowledges funding from the Plan Andaluz de Investigación, Desarrollo e Innovación (PAIDI 2020) of Junta de Andalucía. JV and RP acknowledge financial support from the Spanish Research Agency (Project No. PID2020-115864RB-I00). JV also thanks financial support from the Spanish CM “Talento Program” (Project No. 2020-T1/ND-20306). JC acknowledges funding from the Spanish Ministry of Science and Innovation (Grant No. PID2020-114880 GB-I00). We thank the Spanish MINECO for the “María de Maeztu” Programme for Units of Excellence in R&D (grant No. CEX2018-000805-M). LZ thanks financial support from the Universidad Autónoma de Madrid and the Comunidad de Madrid (grant No. SI3/PJI/2021-00191).
References
1. Cuevas JC, Scheer E. Molecular Electronics: An Introduction to Theory and Experiment. 2nd ed. World Scientific (2017).
3. Amdursky N, Marchak D, Sepunaru L, Pecht I, Sheves M, Cahen D. Electronic Transport via Proteins. Adv Mater (2014) 26:7142–61. doi:10.1002/adma.201402304
4. Alfinito E, Reggiani L, Pousset J. Proteotronics: Electronic Devices Based on Proteins. In: Sensors. Springer (2015). p. 3–7.
5. Bostick CD, Mukhopadhyay S, Pecht I, Sheves M, Cahen D, Lederman D. Protein Bioelectronics: A Review of what We Do and Do Not Know. Rep Prog Phys (2018) 81:026601. doi:10.1088/1361-6633/aa85f2
6. Ing NL, El-Naggar MY, Hochbaum AI. Going the Distance: Long-Range Conductivity in Protein and Peptide Bioelectronic Materials. J Phys Chem B (2018) 122:10403–23. doi:10.1021/acs.jpcb.8b07431
7. Qiu X, Chiechi RC. Printable Logic Circuits Comprising Self-Assembled Protein Complexes. Nat Commun (2022) 13:2312. doi:10.1038/s41467-022-30038-8
8. Artés JM, López-Martínez M, Díez-Pérez I, Sanz F, Gorostiza P. Conductance Switching in Single Wired Redox Proteins. Small (2014) 10:2537–41. doi:10.1002/smll.201303753
9. Solomon EI, Szilagyi RK, DeBeer George S, Basumallick L. Electronic Structures of Metal Sites in Proteins and Models: Contributions to Function in Blue Copper Proteins. Chem Rev (2004) 104:419–58. doi:10.1021/ja00301a02410.1021/cr0206317
10. Huheey JE, Keiter EA, Keiter RL. Inorganic Chemistry: Principles of Structure and Reactivity. 4th ed. HarperCollins College Publishers (1993).
11. Baker EN. Structure of Azurin from Alcaligenes Denitrificans Refinement at 1·8 Å Resolution and Comparison of the Two Crystallographically Independent Molecules. J Mol Biol (1988) 203:1071–95. doi:10.1016/0022-2836(88)90129-5
12. Nar H, Messerschmidt A, Huber R, van de Kamp M, Canters GW. Crystal Structure Analysis of Oxidized Pseudomonas aeruginosa Azurin at pH 5·5 and pH 9·0. J Mol Biol (1991) 221:765–72. doi:10.1016/0022-2836(91)80173-R
13. Groeneveld CM, Canters GW, Aasa R, Reinhammar B. EPR of Azurins from pseudomonas Aeruginosa and Alcaligenes Denitrificans Demonstrates pH-Dependence of the Copper-Site Geometry in pseudomonas Aeruginosa Protein. J Inorg Biochem (1987) 31:143–54. doi:10.1016/0162-0134(87)80059-4
14. Murphy LM, Strange RW, Karlsson BG, Lundberg LG, Pascher T, Reinhammar B, et al. Structural Characterization of Azurin from pseudomonas Aeruginosa and Some of its Methionine-121 Mutants. Biochemistry (1993) 32:1965–75. doi:10.1021/bi00059a013
15. Fittipaldi M, Warmerdam GCM, de Waal EC, Canters GW, Cavazzini D, Rossi GL, et al. Spin-density Distribution in the Copper Site of Azurin. ChemPhysChem (2006) 7:1286–93. doi:10.1002/cphc.200500551
16. Lipton AS, Heck RW, de Jong WA, Gao AR, Wu X, Roehrich A, et al. Low Temperature 65Cu NMR Spectroscopy of the Cu+ Site in Azurin. J Am Chem Soc (2009) 131:13992–9. doi:10.1021/ja901308v
17. Penfield KW, Gewirth AA, Solomon EI. Electronic Structure and Bonding of the Blue Copper Site in Plastocyanin. J Am Chem Soc (1985) 107:4519–29. doi:10.1021/ja00301a024
18. Gewirth AA, Solomon EI. Electronic Structure of Plastocyanin: Excited State Spectral Features. J Am Chem Soc (1988) 110:3811–9. doi:10.1021/ja00220a015
19. Guckert JA, Lowery MD, Solomon EI. Electronic Structure of the Reduced Blue Copper Active Site: Contributions to Reduction Potentials and Geometry. J Am Chem Soc (1995) 117:2817–44. doi:10.1038/nchem.248910.1021/ja00115a016
20. LaCroix LB, Shadle SE, Wang Y, Averill BA, Hedman B, Hodgson KO, et al. Electronic Structure of the Perturbed Blue Copper Site in Nitrite Reductase: Spectroscopic Properties, Bonding, and Implications for the Entatic/rack State. J Am Chem Soc (1996) 118:7755–68. doi:10.1021/ja961217p
21. Solomon EI, Penfield KW, Gewirth AA, Lowery MD, Shadle SE, Guckert JA, et al. Electronic Structure of the Oxidized and Reduced Blue Copper Sites: Contributions to the Electron Transfer Pathway, Reduction Potential, and Geometry. Inorg Chim Acta (1996) 243:67–78. doi:10.1016/0020-1693(95)04893-6
22. Ron I, Sepunaru L, Itzhakov S, Belenkova T, Friedman N, Pecht I, et al. Proteins as Electronic Materials: Electron Transport through Solid-State Protein Monolayer Junctions. J Am Chem Soc (2010) 132:4131–40. doi:10.1021/ja907328r
23. Artés JM, Díez-Pérez I, Sanz F, Gorostiza P. Direct Measurement of Electron Transfer Distance Decay Constants of Single Redox Proteins by Electrochemical Tunneling Spectroscopy. ACS Nano (2011) 5:2060–6. doi:10.1021/nn103236e
24. Artés JM, Díez-Pérez I, Gorostiza P. Transistor-like Behavior of Single Metalloprotein Junctions. Nano Lett (2012) 12:2679–84. doi:10.1021/nl2028969
25. Baldacchini C, Kumar V, Bizzarri AR, Cannistraro S. Electron Tunnelling through Single Azurin Molecules Can Be On/off Switched by Voltage Pulses. Appl Phys Lett (2015) 106:183701. doi:10.1063/1.4919911
26. Yu X, Lovrincic R, Sepunaru L, Li W, Vilan A, Pecht I, et al. Insights into Solid-State Electron Transport through Proteins from Inelastic Tunneling Spectroscopy: The Case of Azurin. ACS Nano (2015) 9:9955–63. doi:10.1021/acsnano.5b03950
27. Kradolfer S, Lipiec E, Baldacchini C, Bizzarri AR, Cannistraro S, Zenobi R. Vibrational Changes Induced by Electron Transfer in Surface Bound Azurin Metalloprotein Studied by Tip-Enhanced Raman Spectroscopy and Scanning Tunneling Microscopy. ACS Nano (2017) 11:12824–31. doi:10.1021/acsnano.7b07768
28. Fereiro JA, Yu X, Pecht I, Sheves M, Cuevas JC, Cahen D. Tunneling Explains Efficient Electron Transport via Protein Junctions. Proc Natl Acad Sci U.S.A (2018) 115:E4577. doi:10.1073/pnas.1719867115
29. Pradhan B, Engelhard C, Van Mulken S, Miao X, Canters GW, Orrit M. Single Electron Transfer Events and Dynamical Heterogeneity in the Small Protein Azurin from pseudomonas Aeruginosa. Chem Sci (2020) 11:763–71. doi:10.1039/C9SC05405G
30. Artés JM, López-Martínez M, Giraudet A, Díez-Pérez I, Sanz F, Gorostiza P. Current-voltage Characteristics and Transition Voltage Spectroscopy of Individual Redox Proteins. J Am Chem Soc (2012) 134:20218–21. doi:10.1021/ja3080242
31. Baldacchini C, Bizzarri AR, Cannistraro S. Electron Transfer, Conduction and Biorecognition Properties of the Redox Metalloprotein Azurin Assembled onto Inorganic Substrates. Eur Polym J (2016) 83:407–27. doi:10.1016/j.eurpolymj.2016.04.030
32. Li W, Sepunaru L, Amdursky N, Cohen SR, Pecht I, Sheves M, et al. Temperature and Force Dependence of Nanoscale Electron Transport via the Cu Protein Azurin. ACS Nano (2012) 6:10816–24. doi:10.1021/nn3041705
33. Alessandrini A, Facci P. Electron Transfer in Nanobiodevices. Eur Polym J (2016) 83:450–66. doi:10.1016/j.eurpolymj.2016.03.028
34. Kayser B, Fereiro JA, Bhattacharyya R, Cohen SR, Vilan A, Pecht I, et al. Solid-state Electron Transport via the Protein Azurin Is Temperature-independent Down to 4 K. J Phys Chem Lett (2020) 11:144–51. doi:10.1021/acs.jpclett.9b03120
35. Zhao J, Davis JJ, Sansom MSP, Hung A. Exploring the Electronic and Mechanical Properties of Protein Using Conducting Atomic Force Microscopy. J Am Chem Soc (2004) 126:5601–9. doi:10.1021/ja039392a
36. Fereiro JA, Porat G, Bendikov T, Pecht I, Sheves M, Cahen D. Protein Electronics: Chemical Modulation of Contacts Control Energy Level Alignment in Gold-Azurin-Gold Junctions. J Am Chem Soc (2018) 140:13317–26. doi:10.1021/jacs.8b07742
37. Zhang B, Song W, Pang P, Lai H, Chen Q, Zhang P, et al. Role of Contacts in Long-Range Protein Conductance. Proc Natl Acad Sci U.S.A (2019) 116:5886–91. doi:10.1073/pnas.1819674116
38. Ruiz MP, Aragonès AC, Camarero N, Vilhena JG, Ortega M, Zotti LA, et al. Bioengineering a Single-Protein junction. J Am Chem Soc (2017) 139:15337–46. doi:10.1021/jacs.7b06130
39. Fereiro JA, Bendikov T, Pecht I, Sheves M, Cahen D. Protein Binding and Orientation Matter: Bias-Induced Conductance Switching in a Mutated Azurin junction. J Am Chem Soc (2020) 142:19217–25. doi:10.1021/jacs.0c08836
40. Ha TQ, Planje IJ, White JRG, Aragonès AC, Díez-Pérez I. Charge Transport at the Protein-Electrode Interface in the Emerging Field of BioMolecular Electronics. Curr Opin Electrochemistry (2021) 28:100734. doi:10.1016/j.coelec.2021.100734
41. Valianti S, Cuevas J-C, Skourtis SS. Charge-transport Mechanisms in Azurin-Based Monolayer Junctions. J Phys Chem C (2019) 123:5907–22. doi:10.1021/acs.jpcc.9b00135
42. Amdursky N, Sepunaru L, Raichlin S, Pecht I, Sheves M, Cahen D. Electron Transfer Proteins as Electronic Conductors: Significance of the Metal and its Binding Site in the Blue Cu Protein, Azurin. Adv Sci (2015) 2:1400026. doi:10.1002/advs.201400026
43. Cahen D, Pecht I, Sheves M. What Can We Learn from Protein-Based Electron Transport Junctions? J Phys Chem Lett (2021) 12:11598–603. doi:10.1021/acs.jpclett.1c02446
44. Zotti LA, Cuevas JC. Electron Transport through Homopeptides: Are They Really Good Conductors? ACS Omega (2018) 3:3778–85. doi:10.1021/acsomega.7b01917
45. Schosser WM, Zotti LA, Cuevas JC, Pauly F. Doping Hepta-Alanine with Tryptophan: A Theoretical Study of its Effect on the Electrical Conductance of Peptide-Based Single-Molecule Junctions. J Chem Phys (2019) 150:174705. doi:10.1063/1.5090457
46. Zotti LA, Bednarz B, Hurtado-Gallego J, Cabosart D, Rubio-Bollinger G, Agrait N, et al. Can One Define the Conductance of Amino Acids? Biomolecules (2019) 9:580. doi:10.3390/biom9100580
47. Pierloot K, De Kerpel JOA, Ryde U, Olsson MHM, Roos BO. Relation between the Structure and Spectroscopic Properties of Blue Copper Proteins. J Am Chem Soc (1998) 120:13156–66. doi:10.1021/ja982385f
48. van Gastel M, Coremans JWA, Sommerdijk H, van Hemert MC, Groenen EJJ. An Ab Initio Quantum-Chemical Study of the Blue-Copper Site of Azurin. J Am Chem Soc (2002) 124:2035–41. doi:10.1021/ja0028166
49. Corni S, De Rienzo F, Di Felice R, Molinari E. Role of the Electronic Properties of Azurin Active Site in the Electron-Transfer Process. Int J Quan Chem. (2005) 102:328–42. doi:10.1002/qua.20374
50. Sarangi R, Gorelsky SI, Basumallick L, Hwang HJ, Pratt RC, Stack TD, et al. Spectroscopic and Density Functional Theory Studies of the Blue-Copper Site in M121SeM and C112SeC Azurin: Cu-Se versus Cu-S Bonding. J Am Chem Soc (2008) 130:3866–77. doi:10.1021/ja076495a
51. Hadt RG, Sun N, Marshall NM, Hodgson KO, Hedman B, Lu Y, et al. Spectroscopic and DFT Studies of Second-Sphere Variants of the Type 1 Copper Site in Azurin: Covalent and Nonlocal Electrostatic Contributions to Reduction Potentials. J Am Chem Soc (2012) 134:16701–16. doi:10.1021/ja306438n
52. Lancaster KM, Zaballa M-E, Sproules S, Sundararajan M, DeBeer S, Richards JH, et al. Outer-sphere Contributions to the Electronic Structure of Type Zero Copper Proteins. J Am Chem Soc (2012) 134:8241–53. doi:10.1021/ja302190r
53. Bernini C, Andruniów T, Olivucci M, Pogni R, Basosi R, Sinicropi A. Effects of the Protein Environment on the Spectral Properties of Tryptophan Radicals in pseudomonas Aeruginosa Azurin. J Am Chem Soc (2013) 135:4822–33. doi:10.1021/ja400464n
54. Bím D, Alexandrova AN. Electrostatic Regulation of Blue Copper Sites. Chem Sci (2021) 12:11406–13. doi:10.1039/D1SC02233D
55. Zhang Y, Yu X, Tian G, Zhu Y. First-principles Study on the Structure and Optical Spectroscopy of the Redox-Active center of Blue Copper Proteins. Chem Phys (2020) 537:110859. doi:10.1016/j.chemphys.2020.110859
56. Romero-Muñiz C, Ortega M, Vilhena JG, Díez-Pérez I, Cuevas JC, Pérez R, et al. Ab Initio electronic Structure Calculations of Entire Blue Copper Azurins. Phys Chem Chem Phys (2018) 20:30392–402. doi:10.1039/C8CP06862C
57. Romero-Muñiz C, Ortega M, Vilhena JG, Diéz-Pérez I, Cuevas JC, Pérez R, et al. Mechanical Deformation and Electronic Structure of a Blue Copper Azurin in a Solid-State junction. Biomolecules (2019) 9:506. doi:10.3390/biom9090506
58. Romero-Muñiz C, Ortega M, Vilhena JG, Díez-Pérez I, Pérez R, Cuevas JC, et al. Can Electron Transport through a Blue-Copper Azurin Be Coherent? an Ab Initio Study. J Phys Chem C (2021) 125:1693–702. doi:10.1021/acs.jpcc.0c09364
59. Romero-Muñiz C, OrtegaOrtega M, Vilhena JG, Pérez R, Cuevas JC, Zotti LA. The Role of Metal Ions in the Electron Transport through Azurin-Based Junctions. Appl Sci (2021) 11:3732. doi:10.3390/app11093732
60. Fereiro JA, Kayser B, Romero‐Muñiz C, Vilan A, Dolgikh DA, Chertkova RV, et al. A Solid‐State Protein Junction Serves as a Bias‐Induced Current Switch. Angew Chem Int Ed (2019) 58:11852–9. doi:10.1002/anie.201906032
61. Agam Y, Nandi R, Kaushansky A, Peskin U, Amdursky N. The Porphyrin Ring rather Than the Metal Ion Dictates Long-Range Electron Transport across Proteins Suggesting Coherence-Assisted Mechanism. Proc Natl Acad Sci U.S.A (2020) 117:32260–6. doi:10.1073/pnas.2008741117
62. Matsuura Y. Coherent Spin Transport in a Natural Metalloprotein Molecule. J Appl Phys (2021) 130:184301. doi:10.1063/5.0069552
63. Krishnan S, Lindsay S, Matyushov D, Aksimentiev A. Probing Electrical Conductivity of Proteins through Microscopic Simulations. Biophysical J (2022) 121:538a. doi:10.1016/j.bpj.2021.11.2834
64. Papp E, Jelenfi DP, Veszeli MT, Vattay G. A Landauer Formula for Bioelectronic Applications. Biomolecules (2019) 9:599. doi:10.3390/biom9100599
65. Hu L, Farrokhnia M, Heimdal J, Shleev S, Rulíšek L, Ryde U. Reorganization Energy for Internal Electron Transfer in Multicopper Oxidases. J Phys Chem B (2011) 115:13111–26. doi:10.1021/jp205897z
66. Kontkanen OV, Biriukov D, Futera Z. Reorganization Free Energy of Copper Proteins in Solution, in Vacuum, and on Metal Surfaces. J Chem Phys (2022) 156:175101. doi:10.1063/5.0085141
67. Chen X, Chen M, Wolynes PG, Wittung-Stafshede P, Gray HB. Frustration Dynamics and Electron-Transfer Reorganization Energies in Wild-type and Mutant Azurins. J Am Chem Soc (2022) 144:4178–85. doi:10.1021/jacs.1c13454
Keywords: protein, azurin, biomolecular electronics, DFT, electron transport, molecular dynamics, amino acids, peptides
Citation: Romero-Muñiz C, Vilhena JG, Pérez R, Cuevas JC and Zotti LA (2022) Recent Advances in Understanding the Electron Transport Through Metal-Azurin-Metal Junctions. Front. Phys. 10:950929. doi: 10.3389/fphy.2022.950929
Received: 23 May 2022; Accepted: 07 June 2022;
Published: 04 July 2022.
Edited by:
Alexander Smogunov, CEA Saclay, FranceReviewed by:
Ruby Srivastava, Centre for Cellular and Molecular Biology (CCMB), IndiaCopyright © 2022 Romero-Muñiz, Vilhena, Pérez, Cuevas and Zotti. This is an open-access article distributed under the terms of the Creative Commons Attribution License (CC BY). The use, distribution or reproduction in other forums is permitted, provided the original author(s) and the copyright owner(s) are credited and that the original publication in this journal is cited, in accordance with accepted academic practice. No use, distribution or reproduction is permitted which does not comply with these terms.
*Correspondence: L. A. Zotti, bGluZGEuem90dGlAdWFtLmVz