- 1Aerospace Information Research Institute, Chinese Academy of Sciences, Beijing, China
- 2School of Optoelectronics, University of Chinese Academy of Sciences, Beijing, China
To meet the needs of high-power fiber lasers, a new fiber structure called chirally coupled core (CCC) fiber has attracted the attention of researchers all over the world. CCC fiber consists of two cores, one of which is a central core distributed along the axial direction, and the other is a side core that is offset from the central axis and spirally distributed around the central core. Meanwhile, CCC fibers are helical-translation symmetric. The unique structure results in advantages of robust single-mode performance, mode-distortion-free splicing and compact coiling of CCC fiber. Based on a brief description of the theory about CCC fiber, this article focuses on the research progress and application prospect of CCC fiber.
1 Introduction
The rise in output power from different kinds of laser sources over the past decades has gradually made it possible to realize (or closer to) the directional energy, high-performance material processing, laser propulsion, etc., [1–8] Fiber laser has a distinguished position amongst high-power lasers due to the advantages of high efficiency, high beam quality and strong environmental adaptability, therefore, has become one of the key breakthrough directions in laser community. In recent years, the development momentum of fiber laser has been extremely rapid, and its output power has been greatly improved [9, 10].
In the past few years, people have devoted themselves to the power scaling of narrow linewidth (<100 GHz) output in all polarization-maintaining fiber lasers. In 2017, IPG Photonics developed a 1.5 kW polarization-maintaining narrow linewidth laser and a 2 kW non-polarization-maintaining narrow linewidth laser [11]. In 2018, they further increased the output power of the polarization-maintaining fiber laser to 2 kW, and the output power of the non-polarization-maintaining fiber laser to 2.5 kW [12]. In 2019, Huang et al. from the Department of Precision Instruments of Tsinghua University built a few-longitudinal-mode fiber oscillator using a narrow-linewidth fiber Bragg grating [13]. After amplification, a 2.19 kW laser output was achieved, with an output efficiency of 78.3%. In 2020, Wang et al. from the Institute of Applied Electronics, China Academy of Engineering Physics demonstrated a 3 kW-class narrow linewidth polarization-maintaining fiber laser [14]. The output power is up to 3.08 kW with the beam quality factor (M2) of 1.4 and the polarization extinction ratio of 94%. However, as the output power of the fiber laser increases, nonlinear effects such as stimulated Raman scattering (SRS), stimulated Brillouin scattering (SBS) and transverse mode instability (TMI) effects in the fiber gradually become apparent, leading to the reduction of output power and the degradation of beam quality [15–19]. These nonlinear effects limit the further improvement of fiber laser output power. In order to solve such problems, researchers have turned their attention to improving the structure and materials of optical fibers, hoping to design fibers that meet high peak power output. At present, commonly used fibers include large mode area fiber (LMA) and photonic crystal fiber (PCF). LMA fiber can also be used to achieve high power output from fiber lasers. But the large mode field area optical fiber transmission process easily leads to the appearance of high-order modes, and it is necessary to adopt the correct and reasonable mode control methods such as bending and coiling to realize single-mode transmission. Moreover, once the core diameter of a fiber with a large mode field area is larger than 25 μm, it is difficult to stably control the transmission mode of the fiber. Although PCF fiber can achieve single-mode output, it will cause great mode loss. It is not conducive to the integration of the system.
In response to the above problems, in 2007, the Ultrafast Optics Research Center of the University of Michigan [20] proposed a new optical fiber structure named chirally-coupled core (CCC) fiber. It can break through the limitation of the normalized cut-off frequency of V = 2.405 of traditional single-mode fiber, and achieve stable single-mode output in the case of large core size (greater than 30 μm) without any mode control technology. In addition, CCC fibers offer the benefits of modal distortion-free splices and compact coils (coil radius less than 15 cm), matching optics fabricated using standard fiber splicing and processing techniques [21]. With the advantage of high integration and high TMI threshold, CCC fiber provides a new approach to realizing high peak power and high energy lasing, therefore has become a promising research area in the laser community.
In this paper, the recent advances in CCC fiber are reviewed along with the introduction of its basic structure and related parameters. Meanwhile, the special functions of the CCC structure are expounded from three aspects, and the coupling between the higher-order modes in the fiber is discussed from the quasi-phase matching condition.
2 Concept and Development of Chirally Coupled Core Fiber
2.1 Basic Structure of Chirally Coupled Core Fiber
Chirality refers to the fact that the object cannot coincide with the mirror image after any spatial operation such as translation and rotation. The macroscopic continuous media formed by such chirality analysis are called chiral media. As early as 1989, Engheta and Pelet [22] proposed the concept of chiral waveguides, namely waveguide structures containing chiral media.
Different from the ordinary optical fibers, chiral coupling fibers are composed of two chiral waveguide fibers. There is a central straight core on the shaft with a large core diameter, which can be over 55 μm. An additional helix-side core deviates from the central one, which forms the CCC around the central straight core. Figure 1A shows the 3D geometry of this structure and Figure 1B shows the cross-section view. This chiral coupling fiber [22] structure can be formed in the fiber prefabricated rod using a conventional fiber prefabricated bar. The central straight core is used for signal light transmission [23]. The main function of the helix-side core [24, 25] is to control the mode of the central straight core, coupling the high order mode into the side core and producing high loss to it. The base modes [26, 27] in the central core can be transmitted almost without loss. In this way, the CCC fiber [28–33] does not rely on any mode control technology to maintain a single-mode transmission while achieving a large mode field area. And the above problems are well solved.
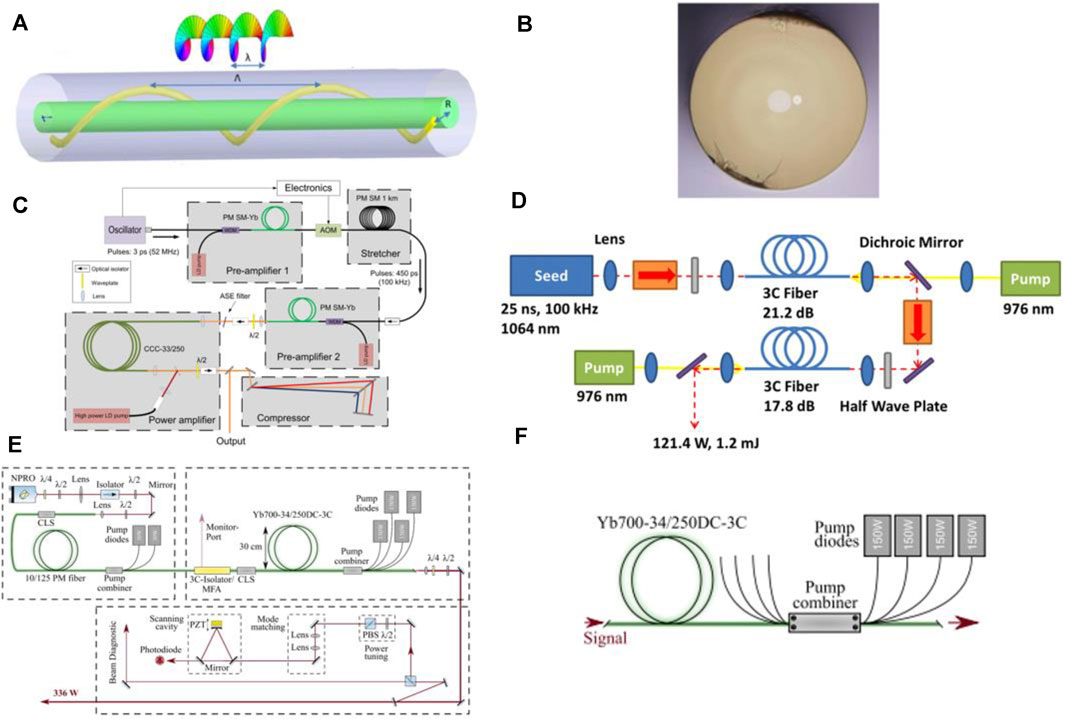
Figure 1. (A) Three-dimensional geometry of CCC structure [22], (B) Cross-section view of CCC fiber [22], (C) Experimental setup of a FCPA [51], (D) Fiber laser system configuration [56], (E) Experimental setup of the monolithic amplifier based on a CCC fiber in the second amplifier stage [60], (F) Experimental setup of an all-fiber amplifier based on a pump combiner directly integrated in an Yb-doped CCC fiber [65].
The reason why CCC can achieve stable single-mode transmission in the case of large core diameter is because of the special helical structure of its side core [34, 35]. The composite structure of the new type of fiber in which the side cores spiral around the central core can achieve the following three functions.
1) Firstly, the phase velocity matching of the fundamental mode in the central core and the mode in the side core is achieved, enabling the coupling of the two modes. Usually, the mode [36, 37] coupling between the two waveguides should satisfy the exact phase velocity matching condition, that is, the mode transmission constants of the two waveguides are equal. But in the CCC fibers, due to the existence of the helical factor, the transmission constants of the modes in the two cores are no longer equal, which will lead to the additional phase difference, so the matching condition becomes Angular-momentum [38]:
where
where
2) Secondly, efficient symmetrical selective coupling of higher-order modes between the central straight core and the helix-side cores is provided by satisfying the quasi-phase matching condition. The quasi-phase matching condition can be expressed as:
where
The value of
3) Thirdly, a reasonable selection of side core size, offset
2.2 Research Status of Chirally Coupled Core Fiber
In 2007, Liu et al. from the University of Michigan [38, 39] proposed the concept of CCC fiber and confirmed that CCC fiber is equivalent to standard single-mode fiber with single-mode transmission characteristics. In order to further verify the simulation results, Liu et al. [40] prepared CCC fiber with a central core diameter of 35 μm and a side core diameter of 12 μm. And the fundamental mode loss of the fiber is 0.095 dB/m. It is transmitted in the core with near lossless. This is the first demonstration of the stable single-mode transmission characteristics of CCC fiber. In 2008, the lab produced an ytterbium-doped double-clad CCC fiber. Among them, the central core diameter is 33 μm and the numerical aperture is 0.06. The side core diameter is 16 μm and the numerical aperture is
In 2009, Huang et al. from the University of Michigan [41] demonstrated single-mode power scaling in fiber laser systems built using 35 μm core Yb-doped double-clad CCC fiber. Output power up to 250 W is demonstrated in their study with pulse width of 10 ns and the pulse energy of 1.14 mJ. The peak power is 114 kW and the amplification slope efficiency reaches 74%. Also, the system output spot is single mode. In 2010, the team applied CCC fiber in a master oscillator power amplifier (MOPA) structure to increase the system output power. In the experiment, a 2.7 m long air-clad Ytterbium-doped CCC fiber was used as the gain medium of the power amplifier. The fiber was excited with 2.2 W signal light and the MOPA structure power output of 511 W was achieved. The amplifier slope efficiency is 70% [42–44]. In 2011, Ma et al. [22] established a new reference frame from optical angular momentum by using Maxwell equations and finite element method. A complete theoretical analysis of the mode effects in the CCC fiber is obtained, and the mode matching conditions of the fiber are obtained. This is also the theoretical basis for the first disclosed CCC fiber. The theoretical basis of CCC fiber has been introduced in the previous article and will not be described here.
The University of Michigan Center for Ultrafast Optics, Sosnowski et al. [45, 46] used CCC fibers to conduct experiments with high average power and high peak power, respectively. The experimental setup for high average power is as follows: The system front end consisted of a 0.5 W 1.06 μm wavelength pulsed diode seed source, a CCC fiber preamplifier and a CCC fiber final stage amplifier. The final amplifier fiber assembly consisted of both Yb-doped and passive 33 μm core, 250 μm clad CCC fibers with a 0.46 NA cladding. The maximum output power achieved is 257 W at 338 W of pump power for a net optical-to-optical efficiency of 76% [47, 48]. Based on CCC large-core-diameter fiber and high-dispersion mirror, Chen et al. [49] demonstrated a high-energy femtosecond laser system in 2011. The high-energy femtosecond laser emits 950 mW average power centered at 1045 nm with 38 MHz repetition rate, corresponding to 25 nJ single pulse energy [50].
In 2013, Želudevičius et al. from the Lithuanian Centre for Physical Sciences and Technology [51] demonstrated a femtosecond fiber chirped-pulse amplification (FCPA) system to enhance output power. The entire FCPA setting is shown in Figure 1C. In addition to the oscillator, it also includes the first preamplifier, an acousto-optic down-counter, a PM SM fiber stretcher, a second preamplifier, a power amplifier and a bulk grating compressor. The power amplification device in the system adopts 33 μm core CCC fiber as a gain medium. After compression with spectral filtering, pulses with a duration of 400 fs and energy as high as 50 μJ were achieved [52, 53].
In 2014, Ma et al. [54] reported further advances in effective single-mode CCC Ge-doped and Yb-doped double-clad fibers geometry, which enables increasing fiber core sizes into 55–60 μm range, and experimentally demonstrate their robust single-mode. In 2017, Pei et al. [55] coherently stacked the output based on an 85 μm Yb-doped CCC fiber into a single pulse. Output energy of 5.4 mJ was obtained at a repetition rate of 1 kHz. In 2018, Bai et al. of Carnegie Mellon University [56] designed a high-power, high-energy system based on a fiber laser and amplifier system. The configuration of the laser system is shown in Figure 1D. The seed amplifies a stable, computer-controlled, pulsed semiconductor laser emitting at 1064 nm. And the fiber has a core diameter of 34 μm and a cladding diameter of 250 μm [57]. This compact system is capable of generating outputs of up to 1.2 mJ for 25 ns pulses at a repetition rate of 100 kHz, with an average power of up to 121 W and a slope efficiency of 82%.
In 2020, Hochheim et al. [58] presented the characterization of a monolithic high-power fiber amplifier at 1064 nm, built using an ytterbium-doped CCC fiber, which achieves an output power of 100 W in a linearly polarized TEM00 mode in an all-fiber setup. Two years later, Hochheim et al. [59] demonstrated a splice-less all-fiber amplifier, where all optical components are directly integrated into a single Yb3+-doped CCC fiber. At an output power of 336 W [60] operating at 1064 nm, a fundamental mode content of 90.4% at a polarization extinction ratio above 13 dB was measured [61–64]. And the experimental setup is shown in Figure 1E. Hochheim et al. [65–67] designed a counter- and side-pumped all-fiber amplifier based on CCC fiber. The setup is shown in Figure 1F. Four fiber-coupled pump diodes deliver the required pump with an output power of 150 W at 976 nm [68–71]. This design allows for a stable and robust amplifier, which ensures a high output of more than 300 W [72].
The output parameters of the above CCC based laser are illustrated Table 1. Because of the special structure of this fiber, it has the advantages of high output power and high higher-order modes loss. However, when the fundamental mode loss changes greatly with the heat load, the slope efficiency of the CCC will be strongly dependent on the operation power [57].
3 Conclusion and Outlook
In general, CCC has many advantages, which not only overcomes the disadvantage of weak guide fiber with great bending loss, but also realize stable single-mode transmission without any mode control technology. A series of related experiments also confirmed that CCC fiber has the advantages of high output power, high slope efficiency and good polarization-maintaining characteristics, which provides an effective way to increase the power of high-power fiber lasers and is conducive to the integrated development of laser systems [73]. In addition, the CCC fiber structure can also control nonlinear effects to realize special functions such as quantum communication [74]. It is certain that the CCC fiber has very important significance and broad development prospects in both scientific research and practical applications.
Author Contributions
MY: Investigation, Writing-original draft preparation. YS: Investigation. ZB: Conceptualization, Writing-review and editing. ZF: Conceptualization, Writing-review and editing, Supervision. All authors have read and agreed to the published version of the manuscript.
Funding
This work was supported by the Guangdong Key Research and Development Program (2018B090904003).
Conflict of Interest
The authors declare that the research was conducted in the absence of any commercial or financial relationships that could be construed as a potential conflict of interest.
Publisher’s Note
All claims expressed in this article are solely those of the authors and do not necessarily represent those of their affiliated organizations, or those of the publisher, the editors and the reviewers. Any product that may be evaluated in this article, or claim that may be made by its manufacturer, is not guaranteed or endorsed by the publisher.
References
1. Zervas MN, Codemard CA. High Power Fiber Lasers: A Review. IEEE J Select Top Quan Electron. (2014) 20:219–41. doi:10.1109/JSTQE.2014.2321279
2. Wang H, Lin L, Ye X. Status and Development Trend of High Power Slab Laser Technology. Infrared Laser Eng (2020) 49:20190456. doi:10.3788/irla.2019-0456
3. Chen H, Bai Z, Yang X, Ding J, Qi Y, Yan B, et al. Enhanced Stimulated Brillouin Scattering Utilizing Raman Conversion in diamond. Appl Phys Lett (2022) 120:181103. doi:10.1063/5.0087092
4. Yang X, Bai Z, Chen D, Chen W, Feng Y, Mildren RP. Widely-tunable Single-Frequency diamond Raman Laser. Opt Express (2021) 29:29449–57. doi:10.1364/OE.435023
5. Bai Z, Zhang Z, Wang K, Gao J, Zhang Z, Yang X, et al. Comprehensive thermal Analysis of diamond in a High-Power Raman Cavity Based on FVM-FEM Coupled Method. Nanomaterials (2021) 11:1572. doi:10.3390/nano11061572
6. Yan Y, Zheng Y, Sun H, Duan J. Review of Issues and Solutions in High-Power Semiconductor Laser Packaging Technology. Front Phys (2021) 9:669591. doi:10.3389/fphy.2021.669591
7. Saraceno CJ, Sutter D, Metzger T, Abdou Ahmed M. The Amazing Progress of High-Power Ultrafast Thin-Disk Lasers. J Eur Opt Soc.-Rapid Publ (2019) 15:15. doi:10.1186/s41476-019-0108-1
8. Bai Z, Williams RJ, Kitzler O, Sarang S, Spence DJ, Wang Y, et al. Diamond Brillouin Laser in the Visible. APL Photon (2020) 5:031301. doi:10.1063/1.5134907
9. Supradeepa VR, Feng Y, Nicholson JW. Raman Fiber Lasers. J Opt (2017) 19:023001. doi:10.1088/2040-8986/19/2/023001
10. Wang WC, Zhou B, Xu SH, Yang ZM, Zhang QY. Recent Advances in Soft Optical Glass Fiber and Fiber Lasers. Prog Mater Sci (2019) 101:90–171. doi:10.1016/j.pmatsci.2018.11.003
11. Platonov N, Yagodkin R, De La Cruz J, Yusim A, Gapontsev V. 1.5kW Linear Polarized on PM Fiber and 2kW on Non-PM Fiber Narrow Linewidth CW Diffraction-Limited Fiber Amplifier. In: SPIE Proceedings,Components and Packaging for Laser Systems III; February 2017; San Francisco, CA, United States. San Francisco, CA, United States: SPIE Lase (2017). p. 100850M. doi:10.1117/12.2263926
12. Platonov N, Yagodkin R, Cruz JDL, Yusim A, Gapontsev V. Up to 2.5-kW on Non-PM Fiber and 2.0-kW Linear Polarized on PM Fiber Narrow Linewidth CW Diffraction-Limited Fiber Amplifiers in All-Fiber Format. In: Proceeding of the Fiber Lasers XV: Technology and Systems; February 2018; San Francisco, CA, United States. San Francisco, CA, United States: Spie Lase (2018). p. 105120E. doi:10.1117/2291100
13. Huang Y, Yan P, Wang Z, Tian J, Li D, Xiao Q, et al. 219 kW Narrow Linewidth FBG-Based MOPA Configuration Fiber Laser. Opt Express (2019) 27:3136. doi:10.1364/OE.27.003136
14. Wang Y, Wang J, Chang Z, Peng W, Sun Y, Ma Y, et al. Output of 3.08 kW Narrow Linewidth Linearly Polarized All-Fiber Laser Based on a Simple MOPA Structure. High Power Laser Part Beams (2020) 32:011006. doi:10.11884/HPLPB202032.200004
15. Bai Z, Yuan H, Liu Z, Xu P, Gao Q, Williams RJ, et al. Stimulated Brillouin Scattering Materials, Experimental Design and Applications: A Review. Opt Mater (2018) 75:626–45. doi:10.1016/j.optmat.2017.10.035
16. Bai Z, Williams RJ, Jasbeer H, Sarang S, Kitzler O, Mckay A, et al. Large Brightness Enhancement for Quasi-Continuous Beams by diamond Raman Laser Conversion. Opt Lett (2018) 43:563–6. doi:10.1364/OL.43.000563
17. Fu S, Shi W, Feng Y, Zhang L, Yang Z, Xu S, et al. Review of Recent Progress on Single-Frequency Fiber Lasers. J Opt Soc Am B (2017) 34:A49–A62. doi:10.1364/JOSAB.34.000A49
18. Jauregui C, Stihler C, Limpert J. Transverse Mode Instability. Adv Opt Photon (2020) 12:429–84. doi:10.1364/AOP.385184
19. Jauregui C, Eidam T, Otto H-J, Stutzki F, Jansen F, Limpert J, et al. Physical Origin of Mode Instabilities in High-Power Fiber Laser Systems. Opt Express (2012) 20:12912–25. doi:10.1364/OE.20.012912
20. Swan MC, Liu C-H, Guertin D, Jacobsen N, Tankala K, Galvanauskas A. 33μm Core Effectively Single-Mode Chirally-Coupled-Core Fiber Laser at 1064-nm. In: Proceeding of the OFC/NFOEC 2008 - 2008 Conference on Optical Fiber Communication/National Fiber Optic Engineers Conference; February 2008; San Diego, CA, USA. IEEE (2008). p. 10063691. doi:10.1109/OFC.2008.4528781
21. Fermann ME. Single-mode Excitation of Multimode Fibers with Ultrashort Pulses. Opt Lett (1998) 23:52–4. doi:10.1364/ol.23.000052
22. Ma X, Liu CH, Chang G, Galvanauskas A. Angular-momentum Coupled Optical Waves in Chirally-Coupled-Core Fibers. Opt Express (2011) 19:26515–28. doi:10.1364/OE.19.026515
23. Nicholson JW, Yablon AD, Ramachandran S, Ghalmi S. Spatially and Spectrally Resolved Imaging of Modal Content in Large-Mode-Area Fibers. Opt Express (2008) 16:7233. doi:10.1364/OE.16.007233
24. Ulrich R, Simon A. Polarization Optics of Twisted Single-Mode Fibers. Appl Opt (1979) 18:2241–51. doi:10.1364/AO.18.002241
26. Snitzer E. Cylindrical Dielectric Waveguide Modes. J Opt Soc America (1961) 51:5491–8. doi:10.1364/josa.51.000491
27. Nicolet A, Zolla F, Guenneau S. Modelling of Twisted Optical Waveguides with Edge Elements. Eur Phys J Appl Phys (2004) 25:2153–7. doi:10.1051/epjap:2004189
28. Wilson JL, Wang C, Fathy AE, Kang YW. Analysis of Rapidly Twisted Hollow Waveguides. IEEE Trans Microwave Theor Tech (2008) 57:130–9. doi:10.1109/tmtt.2008.2009042
29. Marcuse D. Curvature Loss Formula for Optical Fibers. J Opt Soc America (1976) 66:3216–220. doi:10.1364/josa.66.000216
30. Snyder AW, Love JD. Reflection at a Curved Dielectric Interface - Electromagnetic Tunneling. IEEE (1975) 23:1134–141. doi:10.1109/tmtt.1975.1128514
31. Peschel U, Peschel T, Lederer F. A Compact Device for Highly Efficient Dispersion Compensation in Fiber Transmission. Appl Phys Lett (1995) 67:2111–3. doi:10.1063/1.114736
32. Gibson G, Courtial J, Padgett M, Vasnetsov M, Pas'ko V, Barnett S, et al. Free-space Information Transfer Using Light Beams Carrying Orbital Angular Momentum. Opt Express (2004) 12:5448–56. doi:10.1364/opex.12.005448
33. Dienerowitz M, Mazilu M, Reece PJ, Krauss TF, Dholakia K. Optical Vortex Trap for Resonant Confinement of Metal Nanoparticles. Opt Express (2008) 16:4991–9. doi:10.1364/oe.16.004991
34. Gahagan KT, Swartzlander GA. Optical Vortex Trapping of Particles. Opt Lett (1996) 21:827–9. doi:10.1364/ol.21.000827
35. Allen L, Beijersbergen MW, Spreeuw RJ, Woerdman JP. Orbital Angular Momentum of Light and the Transformation of Laguerre-Gaussian Laser Modes. Phys Rev A (1992) 45:8185–9. doi:10.1103/physreva.45.8185
36. Beth RA. Mechanical Detection and Measurement of the Angular Momentum of Light. Phys Rev A (1936) 50:2115–125. doi:10.1103/physrev.50.115
37. Ma X, Hu IN, Galvanauskas A. Propagation-length Independent SRS Threshold in Chirally-Coupled-Core Fibers. Opt Express (2011) 19:22575–81. doi:10.1364/OE.19.022575
38. Liu C-H, Chang G, Litchinitser N, Guertin D, Jacobsen N, Tankala K, et al. Chirally Coupled Core Fibers at 1550-nm and 1064-nm for Effectively Single-Mode Core Size Scaling. In: Proceeding of the 2007 Conference on Lasers and Electro-Optics (CLEO); May 2007; Baltimore, MD, USA. IEEE (2007). doi:10.1109/CLEO.2007.4452926
39. Koplow JP, Kliner DA, Goldberg L. Single-mode Operation of a Coiled Multimode Fiber Amplifier. Opt Lett (2000) 25:442–4. doi:10.1364/ol.25.000442
40. Liu C, Chang G, Litchinitser N, Guertin D, Jacobsen N, Tankala K, et al. Effectively Single-Mode Chirally-Coupled Core Fiber. In: Proceeding of the Advanced Solid-State Photonics; January 2007; Vancouver Canada. Vancouver, Canada: OSA (2007). doi:10.1364/ASSP.2007.ME2
41. Huang S, Zhu C, Liu C, Ma X, Swan C, Galvanauskas A. Power Scaling of CCC Fiber-Based Lasers. In: Proceeding of the Conference on Lasers and Electro-Optics/International Quantum Electronics Conference; May–June 2009; Baltimore, Maryland United States. IEEE (2009). doi:10.1364/cleo.2009.cthgg1
42. Zhu C, Hu IN, Ma X, Galvanauskas A. Single-frequency and Single-Transverse Mode Yb-Doped CCC Fiber MOPA with Robust Polarization SBS-free 511W Output. In: Proceeding of the Advanced Solid-State Photonics 2011; February 2011; Istanbul Turkey. Istanbul, Turkey: OSA (2011). doi:10.1364/assp.2011.amc5
43. Goodno GD, McNaught SJ, Rothenberg JE, McComb TS, Thielen PA, Wickham MG, et al. Active Phase and Polarization Locking of a 1.4 kW Fiber Amplifier. Opt Lett (2010) 35:1542–4. doi:10.1364/OL.35.001542
44. Gray S, Liu A, Walton DT, Wang J, Li MJ, Chen X, et al. 502 Watt, Single Transverse Mode, Narrow Linewidth, Bidirectionally Pumped Yb-Doped Fiber Amplifier. Opt Express (2007) 15:17044–50. doi:10.1364/oe.15.017044
45. Sosnowski T, Kuznetsov A, Maynard R, Ma X, Zhu C, Hu I-N, et al. 3C Yb-Doped Fiber Based High Energy and Power Pulsed Fiber Lasers. In: Proceeding of the Fiber Lasers X: Technology, Systems, and Applications,SPIE Proceedings; March 2013; San Francisco, CA, United States. San Francisco, CA, United States: Spie Lase (2013). p. 86011M. doi:10.1117/12.2008306
46. Ma X, Kaplan A, Hu I-N, Galvanauskas A. Characterization of Single-Mode Performance of Chirally-Coupled-Core Fibers with Cores Larger Than 50μm. In: Conference on Lasers and Electro-Optics 2012; May 2012; San Jose, California United States. IEEE (2012). doi:10.1364/CLEO_SI.2012.CM1N.7
47. Brooks CD, Di Teodoro F. Multimegawatt Peak-Power, Single-Transverse-Mode Operation of a 100μm Core Diameter, Yb-Doped Rodlike Photonic crystal Fiber Amplifier. Appl Phys Lett (2006) 89:111119. doi:10.1063/1.2348742
48. Hu I-N, Ma X, Zhu C, Liu C-H, Sosnowski T, Galvanauskas A. Experimental Demonstration of SRS Suppression in Chirally-Coupled-Core Fibers. In: Proceeding of the Advanced Solid-State Photonics 2012; January–February 2012; San Diego, CA United States. San Diego, CA, United States: OSA (2012). doi:10.1364/ASSP.2012.AT1A.3
49. Chen H, Sosnowski T, Liu C, Chen L, Birge JR, Galvanauskas A, et al. High-energy Chirally-Coupled-Core Yb-Fiber Laser with High-Dispersion Mirror Compressor to Achieve 1W-Level, Sub-100fs Pulses with Diffraction-Limited Beam Quality. In: Proceeding of the Advanced Solid-State Photonics 2011; February 2011; Istanbul Turkey. Istanbul, Turkey: OSA (2011). doi:10.1364/assp.2011.atuc5
50. Lefrancois S, Liu CH, Stock ML, Sosnowski TS, Galvanauskas A, Wise FW. High-energy Similariton Fiber Laser Using Chirally Coupled Core Fiber. Opt Lett (2013) 38:43–5. doi:10.1364/OL.38.000043
51. Želudevičius J, Danilevičius R, Viskontas K, Viskontas N, Regelskis K. Femtosecond Fiber CPA System Based on Picosecond Master Oscillator and Power Amplifier with CCC Fiber. Opt Express (2013) 21:55338–5345. doi:10.1364/OE.21.005338
52. Jeong YC, Boyland AJ, Sahu JK, Chung SH, Nilsson J, Payne DN. Multi-kilowatt Single-Mode Ytterbium-Doped Large-Core Fiber Laser. J Opt Soc Korea (2009) 13:4416–422. doi:10.3807/josk.2009.13.4.416
53. Strickland D, Mourou G. Compression of Amplified Chirped Optical Pulses. Opt Commun (1985) 56:3219–221. doi:10.1016/0030-4018(85)90120-8
54. Ma X, Zhu C, Hu IN, Kaplan A, Galvanauskas A. Single-mode Chirally-Coupled-Core Fibers with Larger Than 50 Μm Diameter Cores. Opt Express (2014) 22:9206–19. doi:10.1364/OE.22.009206
55. Pei H, Ruppe J, Chen S, Sheikhsofla M, Nees J, Galvanauskas A. Multi-mJ Ultrashort Pulse Coherent Pulse Stacking Amplification in a Yb-Doped 85µm CCC Fiber Based System. In: Proceeding of the Conference on Lasers and Electro-Optics; May 2017; San Jose, CA United States. San Jose, CA, United States: OSA (2017). doi:10.1364/CLEO_SI.2017.SM1L.2
56. Bai J, Zhang J, Koponen J, Kanskar M, Towe E. A Unified Approach to Achieving High Power and High Energy in Chirally Coupled-Core Ytterbium-Doped Fiber Amplifier Systems. IEEE Photon J. (2018) 10:1–8. doi:10.1109/JPHOT.2018.2799589
57. Zhu S, Li J, Li L, Sun K, Hu C, Shao X, et al. Impact of the Heat Load on the Laser Performance of Chirally-Coupled-Core Fibers. Opt Express (2019) 27:37522–31. doi:10.1364/OE.380953
58. Hochheim S, Steinke M, Koponen J, Lowder T, Novotny S, Neumann J, et al. Monolithic Amplifier Based on a Chirally-Coupled-Core Fiber. In: Proceeding of the 2019 Conference on Lasers and Electro-Optics Europe & European Quantum Electronics Conference (CLEO/Europe-EQEC); June 2019; Munich, Germany. IEEE (2019). doi:10.1109/CLEOE-EQEC.2019.8872811
59. Hochheim S, Steinke M, Wessels P, de Varona Ortega O, Koponen J, Lowder T, et al. Single-frequency Chirally-Coupled-Core All-Fiber Amplifier with 100W in a Linearly-Polarized TEM00-Mode. In: Proceeding of the Fiber Lasers XVII: Technology and Systems; February 2020; San Francisco, CA, United States. San Francisco, CA, United States: Spie Lase (2020). p. 112601C. doi:10.1117/12.2542192
60. Hochheim S, Brockmuller E, Wessels P, Koponen J, Lowder T, Novotny S, et al. Single-Frequency 336 W Spliceless All-Fiber Amplifier Based on a Chirally-Coupled-Core Fiber for the Next Generation of Gravitational Wave Detectors. J Lightwave Technol (2022) 40:2136–43. doi:10.1109/JLT.2021.3133814
61. Baus C, Weßels P, Neumann J, Kracht D. High Power Single Frequency Solid State Master Oscillator Power Amplifier for Gravitational Wave Detection. Opt Lett (2012) 37:142862–4. doi:10.1364/OL.37.002862
62. Steinke M, Tunnermann H, Kuhn V, Theeg T, Karow M, de Varona O, et al. Single-Frequency Fiber Amplifiers for Next-Generation Gravitational Wave Detectors. IEEE J Select Top Quan Electron. (2018) 24:1–13. doi:10.1109/JSTQE.2017.2759275
63. Wellmann F, Steinke M, Meylahn F, Bode N, Willke B, Overmeyer L, et al. High Power, Single-Frequency, Monolithic Fiber Amplifier for the Next Generation of Gravitational Wave Detectors. Opt Express (2019) 27:28523–33. doi:10.1364/OE.27.028523
64. Rainville A, Chen M, Whittlesey M, Du Q, Galvanauskas A. 22mJ Coherent Beam Combining from Three 85μm Core CCC Fiber Amplifiers. In: Proceeding of the Conference on Lasers and Electro-Optics; May 2021; San Jose, California United States. IEEE (2021). doi:10.1364/CLEO_SI.2021.SW2B.4
65. Hochheim S, Brockmuller E, Wessels P, Steinke M, Koponen J, Lowder T, et al. Highly-Integrated Signal and Pump Combiner in Chirally-Coupled-Core Fibers. J Lightwave Technol (2021) 39:7246–50. doi:10.1109/JLT.2021.3111993
66. Holmen LG, Rustad G, Haakestad MW. Eye-safe Fiber Laser for Long-Range 3D Imaging Applications. Appl Opt (2018) 57:6760–7. doi:10.1364/AO.57.006760
67. Machewirth DP, Wang Q, Samson B, Tankala K, O'Connor M, Alam M. Current Developments in High-Power Monolithic Polarization Maintaining Fiber Amplifiers for Coherent Beam Combining Applications. In: Proceeding of the Fiber Lasers IV: Technology, Systems, and Applications,SPIE Proceedings; February 2007; San Jose, CA, United States. San Jose, CA, United States: Spie Lase (2007). p. 64531F. doi:10.1117/12.712691
68. Wellmann F, Steinke M, Meylahn F, Bode N, Willke B, Overmeyer L, et al. High Power, Single-Frequency, Monolithic Fiber Amplifier for the Next Generation of Gravitational Wave Detectors. Opt Express (2019) 27:28523–33. doi:10.1364/OE.27.028523
69. Winkelmann L, Puncken O, Kluzik R, Veltkamp C, Kwee P, Poeld J, et al. Injection-locked Single-Frequency Laser with an Output Power of 220 W. Appl Phys B (2011) 102:529–38. doi:10.1007/s00340-011-4411-9
70. Theeg T, Sayinc H, Neumann J, Kracht D. All-Fiber Counter-propagation Pumped Single Frequency Amplifier Stage with 300-W Output Power. IEEE (2012) 24:201864–7. doi:10.1109/lpt.2012.2217487
71. Gu G, Kong F, Hawkins T, Parsons J, Jones M, Dunn C, et al. Ytterbium-doped Large-Mode-Area All-Solid Photonic Bandgap Fiber Lasers. Opt Express (2014) 22:13962–8. doi:10.1364/OE.22.013962
72. Limpert J, Schmidt O, Rothhardt J, Röser F, Schreiber T, Tünnermann A, et al. Extended Single-Mode Photonic crystal Fiber Lasers. Opt Express (2006) 14:2715–20. doi:10.1364/oe.14.002715
73. Theeg T, Sayinc H, Neumann J, Overmeyer L, Kracht D. Pump and Signal Combiner for Bi-directional Pumping of All-Fiber Lasers and Amplifiers. Opt Express (2012) 20:28125–41. doi:10.1364/OE.20.028125
Keywords: chirally coupled core fiber, fiber laser, high power, single mode transmission, mode coupling
Citation: Yuan M, Sui Y, Bai Z and Fan Z (2022) Recent Advances in Chirally-Coupled Core Fibers. Front. Phys. 10:950492. doi: 10.3389/fphy.2022.950492
Received: 22 May 2022; Accepted: 13 June 2022;
Published: 04 July 2022.
Edited by:
Xuezong Yang, University of Chinese Academy of Sciences, ChinaReviewed by:
Quan Sheng, Tianjin University, ChinaHuawei Jiang, Shanghai Institute of Optics and Fine Mechanics, (CAS), China
Copyright © 2022 Yuan, Sui, Bai and Fan. This is an open-access article distributed under the terms of the Creative Commons Attribution License (CC BY). The use, distribution or reproduction in other forums is permitted, provided the original author(s) and the copyright owner(s) are credited and that the original publication in this journal is cited, in accordance with accepted academic practice. No use, distribution or reproduction is permitted which does not comply with these terms.
*Correspondence: Zhenao Bai, YmFpemhlbmFvQGhvdG1haWwuY29t; Zhongwei Fan, ZmFuemhvbmd3ZWlAYW9lLmFjLmNu