- 1European Organization for Nuclear Research (CERN), Geneva, Switzerland
- 2Lawrence Berkeley National Laboratory, Berkeley, CA, United States
- 3Physics Department, Università di Milano, Milan, Italy
- 4LASA Laboratory, INFN-Milan, Segrate, Italy
- 5Fermi National Accelerator Laboratory (Fermilab), Batavia, IL, United States
The implications of accelerator magnet R&D towards future colliders are reviewed and discussed. It starts with a brief overview of the present and future accelerator facilities which rely on the significant advances and innovations in key technologies. Then advances and needs for present key projects and studies are expanded on specific examples. This provides the lead to discuss the recent progress in accelerator magnet R&D and the future plans. We conclude with a summary of our view of the major development drivers and future perspectives.
1 Introduction
The last century of extraordinary progress in fundamental physics, seeking answers to the most fundamental questions on the nature and structure of our Universe, has relied on experiments of increasing scale and complexity. Among those experiments, particle accelerators are an example of large-scale scientific endeavors, where several areas of science and engineering are seamlessly integrated into spectacular scientific instruments. One such example is the Large Hadron Collider (LHC) [1], a world’s premier facility presently in operation at the European Organization for Nuclear Research (CERN) near Geneva in Switzerland and uniting the efforts of the international High Energy Physics (HEP) community. The LHC was successful in discovering and measuring properties of the Higgs boson [2, 3], the particle completing the present view of the Standard Model (SM), concluding a chase that lasted half a century. And yet, it is well known that other physics phenomena Beyond the Standard Model (BSM) must exist to explain properties of the Universe not yet understood. Therefore, there is strong interest from the international HEP community in improving the measurement statistics and accessing energy scales well beyond the current reach of almost 14 TeV provided by the LHC. Several projects and studies are on-going in this direction. In this paper we review the implications for accelerator magnet R&D towards future colliders. We give first a brief overview of the future accelerator facilities which demand the most challenging advances in accelerator magnet technologies. On specific examples we expand advances and needs for a selected projects and studies. This provides the lead to discuss the recent progress in accelerator magnet R&D and the future plans. We finally conclude with a summary of our view of the major development drivers and future perspectives.
2 Overview of future accelerator facilities
Several facilities have been proposed, are in discussion and study, or are being constructed to extend the energy reach and explore BSM physics. This follows a process whereby progress in physics discovery and understanding feeds the strategy for future experiments and investments in new or enhanced facilities enabling new physics reach. In Europe, the community process occurs every five to 7 years through the proceedings of a European Strategy Group, resulting in a document on the European Strategy for Particle Physics (ESPP) that provides a shared roadmap and guides European investments in this area [4]. The last ESPP update was recently completed with the publication of an Accelerator R&D Roadmap in 2022 [5]. In the United States, the Strategy for High Energy Physics, including Particle Physics, is also reviewed and revised within eight to 10 years. The process involves a year-long community discussion period known as “The Snowmass Community Planning Exercise” [6]. Snowmass is then followed by a Particle Physics Project Prioritization Panel (P5) which is tasked with the final strategic planning that guides U.S. HEP investments. The last completed Snowmass process was in 2013, culminating in a community planning report, which was then followed by a P5 report in 2014 [7]. A new Snowmass process is going to its final phase as we write.
A concrete result of the European process is the High Luminosity upgrade of the LHC (HL-LHC), described later in more detail. The HL-LHC, which aims at improving the precision of the LHC measurements by a factor 3 or more, was initiated as a project at CERN in 2015, and involves the world-wide accelerator physics and technology community.
A second example, also considered in more detail later, is the Electron-Ion Collider (EIC), to be built at BNL over the 10–15 years as a U.S.-centered effort started in 2020. The Nuclear Science Advisory Committee (NSAC) recommended the EIC as the next major nuclear physics facility for the U.S. Nuclear physics program, with luminosity up to 1034 cm−2s−1 and center of mass (c.o.m.) energy in the range of 20–100 GeV initially, and highly spin polarized electrons, protons, and light ion beams. The EIC will use electrons to probe hadrons, protons and neutrons, and their interactions through the gluons that bind their constituents. It will provide unprecedented capability for understanding the interaction of elemental quarks and gluons that form the basic structure of atoms and nuclei.
Looking at the future of HEP, and beyond existing installations, particle colliders have a prominent role, being considered the most promising means for the next step in both precision and energy reach. A number of candidates are known and relatively well defined. The Future Circular Collider (FCC) proposed by CERN [8] foresees an integrated plan, with a first step, the FCC-ee, consisting of a 100 km lepton (e + e-) collider at c. o.m. Energies in the range of 90–350 GeV to probe with high precision the properties of the Z, W and H bosons, as well as the top quark. The electro-weak factory machine will then make place to a hadron machine, FCC-hh, in the same tunnel repeating the successful scheme of LEP/LHC. By strong high field on the level of 16 T, indeed, the FCC-hh can reach 100 TeV c. o.m. Energy with pp collisions, probing new physics well beyond the LHC reach. The FCC-hh will also provide ion-ion collisions and the possibility of precision physics by means of p-e or ion-e collisions.
The Institute of High Energy Physics (IHEP) of the Chinese Academy of Science (CAS) has a similar proposal of lepton and hadron machines [9] consisting of two steps. The Circular Electron-Positron Collider (CEPC) would first probe the SM heavy particles with precision measurements in the range of 90–240 GeV c. o.m. Energy, to be followed by a Super Proton-Proton Collider (SPPC) installed in the same tunnel, which will collide high energy hadrons in the range of 75 TeV (CDR) to 125–150 TeV (ultimate) c. o.m. Energy. The first step in both proposals foresees circular lepton colliders for precision physics.
Linear colliders are an alternative in the range of energy targeted for precision measurements. The International Linear Collider (ILC) is a 20 km long superconducting RF accelerator proposal, aiming at colliding leptons (e + e-) in the range of 250 GeV (TDR updated) to one TeV (upgrades) c. o.m. Energy, targeting precision measurements of heavy SM particles and their coupling. CERN has proposed a Compact Linear Collider (CLIC) that would serve both for precision measurements, as well as physics at the energy Frontier. The CLIC proposal is an attempt to bring lepton colliders to the energy Frontier, which is presently dominated by circular hadron colliders. The CLIC plans to use copper RF cavities to accelerate and collide leptons (e + e-) in three steps, from a c. o.m. Energy of 380 GeV, to one TeV, and eventually to 3 TeV. The accelerator length would grow correspondingly, in the three steps, from a minimum 11 km (380 GeV) to a maximum of 50 km (3 TeV).
Lepton colliders benefit from the fact that leptons are fundamental particles, and a head-on collision makes the full particle c. o.m. Energy available for a given physics process. By contrast, hadrons are composite particles, and only a fraction of the particle energy is available in each process “channel”, typically one-sixth to one-10th of the c. o.m. Energy. The downside of circular electron-positron colliders is synchrotron radiation, which severely limits the energy reach, as can be seen looking at the planned energy range of the proposed FCC-ee and CEPC. Linear electron-positron colliders avoid the synchrotron radiation problem but require the beam to be accelerated to full energy in a single passage, hence resulting in a very long accelerator and in a waste of energy for a given luminosity (most of the particle are lost without collisions). Scientific reasons (limited energy reach vs. circular hadron colliders, limited luminosity as Higgs and EW factory vs. circular e-e colliders) and energy consumption considerations are now disfavoring linear collider like CLIC, at least as energy Frontier machine. With the highest c. o.m. Energy of 3 TeV, even the final stage of the CLIC (necessitating of almost 600 MW of electric power) is not quite in reach of FCC-hh or SPPC at 100 TeV c. o.m. Energy.
A solution to this conundrum may be provided by a Muon Collider (MC). Muons are leptons, and profit from the same advantage of being point-like particles, but they mitigate the synchrotron radiation because their mass is over 200 times larger than that of electrons. This allows accelerating and colliding muon beams in rings and using technology developed for proton colliders. Since muons are not stable particles, the challenge is to do it within the lifetime of muons, i.e. 2.2 μs in the laboratory frame. Several options of MC were proposed and are being studied. The U.S. Muon Accelerator Program has produced a detailed study of a muon collider at a c. o.m. Energy of 3 TeV [10], while present activities are concentrating on higher c. o.m. Energies, from 6 TeV [11], to 10 TeV and beyond [12, 13].
The proposed layouts of the HL-LHC, EIC, FCC, CEPC/SPPC, and 3 TeV MC are shown in Figure 1 (not in scale).
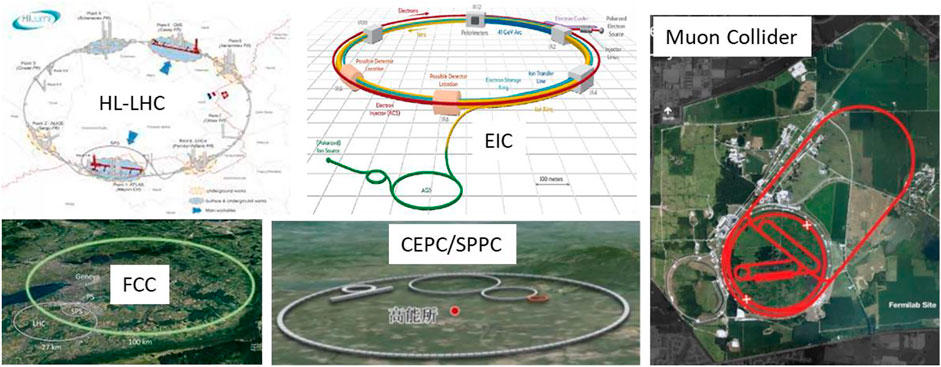
FIGURE 1. Proposed layouts of the HL-LHC (CERN), EIC (BNL), FCC (CERN), CEPC/SPPC (China) and Muon Collider (3 TeV version, FNAL).
Though rather cursory, the above review of ongoing collider projects and upcoming proposals gives a clear impression of a very active community, providing a strong and consistent pull. The implications are major and wide-ranging, in many fields beyond HEP, and especially on magnet technology. Accelerator magnets are the preferred means to form, shape and guide high-energy particle beams, and it is not surprising that accelerator magnet technology has gone hand in hand with the progress in particle colliders. Magnets are required in any type of accelerator, be it circular or linear. However, among the projects and proposals listed above, the challenges posed by future circular hadron and muon colliders are by far the most demanding. In return, progress in magnet technology is a vital ingredient to future colliders. In the following sections we review needs, advances and perspective for future accelerator magnets in direct connection with future colliders.
3 Superconducting magnets for HL-LHC
The High Luminosity LHC is the upgrade of the LHC aiming at the ultimate goal of increasing the collision rate by a factor of 7, in order to attain an integrated luminosity of more than 4,000 fb−1, as ultimate goal. These values are an order of magnitude larger than the similar ones in the LHC [14–16]. The cornerstone of the upgrade are the insertions magnets and especially the new Inner Triplet (IT), consisting of large-aperture low-β quadrupoles, which are designed to reach transverse betatron function at the ATLAS and CMS collision points of β* = 15 cm (the ultimate β* limit of approximately 10 cm). The IT consists of quadrupoles, dipoles and corrector magnets. Additional high-field dipoles are needed for the Dispersion Suppressor (DS), known as 11 T dipoles. A compilation of the cross-sections of the HL-LHC magnets is shown in Figure 2 while Table 1 summarizes the main parameters of the HL-LHC Nb3Sn magnets, the IT quadrupoles and DS (11 T) dipole, compared to the present Nb-Ti LHC inner triplet quadrupoles and the LHC main superconducting dipoles.
3.1 Nb3Sn magnets
To reach such small β*, a set of four quadrupoles (Q1, Q2a, Q2b and Q3) is placed left and right of the LHC high luminosity Insertion Regions, IR1 (ATLAS) and IR5 (CMS). The large 150-mm aperture of the new IT quadrupoles is the main ingredient to reaching small β*. It is much larger than the one in the present 70-mm LHC IT quadrupoles. The large aperture is necessary to accommodate the beam waist, while leaving sufficient space for a thick tungsten shield that decreases the radiation dose to the coils of the IT magnets by a factor of ten. At the same time, the IT quadrupole field gradient must be as high as practical to minimize the IT length. The chosen design gradient of 132 T/m generates a peak of magnetic field of nearly 11.5 T in the coils, which is by far above the reach of Nb-Ti and requires the use of Nb3Sn superconductor. The quadrupoles construction is a joint venture between a team of three U.S. laboratories and CERN. The U.S. laboratories (BNL, FNAL and LBNL) have collaborated for long time in the US-LARP program, especially devised for the R&D required for the LHC upgrade (2004-2018) [17]. Nowadays, those laboratories are federated under the U.S. HL-LHC Accelerator Upgrade Project (US-AUP) for magnet fabrication and test. CERN started R&D for the HL-LHC in 2010, after LHC entered operation, providing guidance as to the parameters necessary for the initial R&D phase in USA, and being the ultimate responsible of HL-LHC [18]. A second challenge of HL-LHC is the increased beam intensity, twice that of the LHC beam. This calls for additional collimators to be placed in the dispersion suppressor around the LHC Point 7 (DS7). To avoid loss-driven quench of the main dipoles around DS7. Due to the tight filling factor of LHC, it is very difficult to recover space by displacing the main dipoles (to do so one would need to remove and replace some 27 dipoles per side!) the only practical way to gain the space necessary for the collimators without a large change of the machine layout, is to remove one main dipole, which is worth of 8.33 T field over 14.3 m magnetic length, and then to replace it with an 11 T long dipole [16], to maintain the same bending strength of about 121 Tm at collision energy with a reduced magnetic length of 11 m. As for the IT quadrupoles, the new higher field value imposes to use Nb3Sn superconductor for the coil. An 11 T dipole unit is composed of two magnets of about 5.5 m length with an approximate 4 m space to place the collimator in between the two Nb3Sn magnets. The 11 T dipole R&D was launched at the start of the HL-LHC project, at the end of 2010 when FNAL and CERN initiated a collaboration. FNAL supported the construction of a few short model magnets and played a key role in transferring to CERN the experience in Nb3Sn technology [19, 20]. CERN, after the construction of a few models, proceeds to the construction of full size 11 T dipoles.
Both the IT quadrupoles and 11 T dipoles were designed and manufactured in accordance with some simple decisions taken at the project outset, adapted to the small series of magnets planned (few tens). Operation at 1.9 K was chosen as a reference condition, compatible with LHC cryogenics. The operating point was taken at 80% of critical conditions along the magnet load line, where the critical surface is defined by strand measurement extracted from the Rutherford cable used in the magnet. For Nb3Sn in the range of interest, this corresponds to a fraction of critical current of about 50%, and a temperature margin of at least 4.5 K. For the IT quadrupoles the operating point was reduced to increase margin and adjusted to 78% of the load line. The peak stress was limited to 150 MPa in nominal conditions at any location in the coil. This level of stress was found to be the upper limit for brittle Nb3Sn superconductor, based on experimental data on wires and cables [21, 22]. The maximum allowable hot-spot temperature was set at 350 K, considering all nominal and off-nominal protection scenarios (with up to two independent failures).
The coils are based on a cos (nϑ) layout with two layers and no grading. The benefits of not grading are the use of a one type of cable, made with one kind of wire, simplifying conductor procurement and coil technology, a reduction of stress and hot-spot temperature due to a lower engineering current density, and avoiding internal joints. The coils are manufactured using the wind-and-react technique by winding an insulated cable, heat treating, and impregnating the coils with epoxy resin. This is the only practical solution to wind the brittle conductor around the tight radius at the coil ends. The turn and ground insulations are made of a S2 glass, which is braided directly on the Rutherford cable (turn), or applied as sheets (ground), acting as a spacer during winding and heat treatment. The impregnation is performed after heat treatment using the epoxy resin CTD101K, casting the fragile strands in a composite with sufficient dielectric strength.
The main characteristic of the HL-LHC strands is the critical current density, that is specified to be 2450 A/mm2 at 12 T and 4.2 K. This value is significantly below the best performance achieved in short batches, of 3000 A/mm2 at 12 T and 4.2 K. This was done to limit the cost (the 3000 A/mm2 is not yet consolidated in production) and to cope with the fact that the HL-LHC IT quadrupoles and 11 T dipoles are the first large application of Nb3Sn superconductor in accelerator magnets. A second important remark is on the filament diameter Deff which is relatively large, within 40–70 μm. The magnetization associated with such values is large, and the conductor is prone to flux jumps at low fields. For the IT quadrupoles, field quality is important only at high energy, where the magnetization is forcibly small. For the 11 T dipoles, the planned number of magnets is small (2–4 units at most), and the expected perturbation to the beam due to a sextupole error of about 20 units at injection can be corrected by the regular correction system. For these reasons, a relatively large Deff, a more readily scaled for production with the requisite quality control, was considered sufficient.
The work on HL-LHC conductor has focused on industrial yield and production homogeneity, with piece lengths above 1 km. In total, about 25 tons of Nb3Sn strands have been produced for the HL-LHC magnets. While highly successful, the magnet experience has confirmed that the mechanical properties and limits of the brittle Nb3Sn wires are key parameters in the wire optimization. For a given wire, the irreversible longitudinal strain was found to depend on the heat treatment schedule. It is evident that these results, and the performance degradation experienced in some short model and long prototype magnets, point to the need to revisit the Nb3Sn wires design and manufacturing for a next step in accelerator magnet R&D.
The coil layout of the IT quadrupoles was mainly optimized with the goal of meeting the severe field quality (FQ) requirement at collision energy when the beam size reaches its maximum. The coil has an aperture of 150 mm. It is wound with an insulated cable consisting of 40 strands, 0.85 mm in diameter, an average dimension of bare cable of 1.59 mm by 18.36 mm, and a keystone angle of 0.4°. Two magnetic lengths are employed: 4.2 m to be assembled in a doublet, making a cold mass of nearly 10 m, and 7.15 m. The overall current density at the operating temperature and nominal peak field of 11.5 T in the coil is 460 A/mm2, while stored energy is 1.2 MJ/m. For comparison the stored energy in the LHC dipole is 0.5 MJ/m over the two magnetic channels. The nominal peak stress is 110 MPa.
The 11 T coil design is based on an insulated cable consisting of 40 strands, 0.7 mm in diameter, with average dimension of 1.25 mm by 14.7 mm, and a keystone angle of 0.8°. The coil is wound with an aperture of 60 mm and magnetic length of 5.21 m. The overall current density at operating temperature and nominal field of 11.3 T in the coil is 600 A/mm2, while stored energy is 0.9 MJ/m. The nominal peak stress is 130 MPa.
The wound coil is placed in a steel mold and the Nb-Sn precursors are reacted by heating to 650°C in Argon atmosphere to form superconducting Nb3Sn phase. Superconducting filaments expand by about 4–5% in volume once the Nb3Sn compound is formed. Transverse clearance and mobile parts with gaps are used to avoid straining the coils during the cool-down from 650°C.
The reacted coil is spliced to flexible Nb-Ti leads and instrumented. In this phase the quench protection heaters, made of steel-copper thin strips encapsulated between layers of polyimide, are placed over the coils and covered with glass sheets. The coil is then impregnated under vacuum with CTD101 K epoxy resin, which can withstand about 30 MGy of radiation dose.
The 11 T dipoles use the classical collar structure inside the cold yoke surrounded by thick stainless-steel skin (Figure 3, left) to give to the coil a pre-stress sufficient to balance the effect of electromagnetic loads, as in the Tevatron, HERA and LHC magnets. The coils of the twin-aperture 11 T dipole are collared as separate single units to reduce the manufacturing risk. For the IT quadrupoles, the structure is based on the novel “bladders and keys” (B&K) principle [23], originally developed specifically to address concerns of overstressing brittle superconductors during magnet fabrication. The prestress is applied during assembly at room temperature by opening gaps in the components of the yoke structure, loading an external Al shell using the bladders, and locking the mechanical load state using keys (see Figure 3, right). The pre-stress at room temperature is further increased during cool down due to the shrinking of the external cylinder around the yoke, reaching the desired design value at the operating temperature.
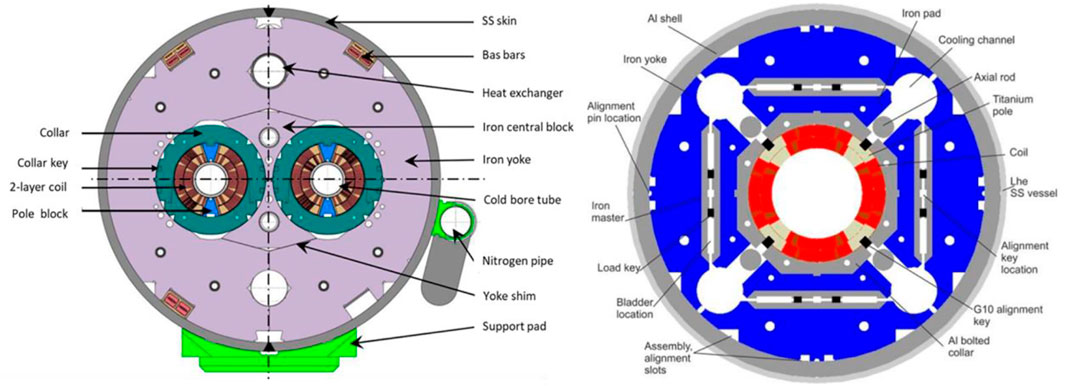
FIGURE 3. Left: cross-section of the 11 T dipole cold mass. Right: cross-section of the MQXF cold mass. The two cross-sections are not in scale.
Both 11T dipole and IT quadrupole have large and comparable engineering current density, though also in this respect the 11 T dipole is more pushed. Also, the values of stored energy per unit length and per coil mass, owing to the combination of high field and large aperture, are decidedly larger than in previous LHC magnets (see Table 1). For both magnets, the basic concept of protection is the same as in the LHC, relying on firing heaters positioned at the coil outer surfaces all along their length, the outer quench heaters (QH). A consequence of the high current density is that quench detection needs to be significantly faster than in the LHC dipoles (10 ms vs. 20–30 ms), and that the active quench protection needs to act within 20 ms. The QHs are subdivided into multiple circuits for redundancy. Bank of capacitors are kept charged and then discharged into the QH, when required, which is in principle sufficient for the protection. Due to the requirement of redundancy for the IT quadrupoles and their critical position, highly exposed to radiation, it has been decided to add the recently developed Coupling Loss Induced Quench (CLIQ). This system provides heating by forcing an oscillating current through the coils, via the AC loss mechanism in the conductor. CLIQ is then an independent protection system and can compensate the loss of one or two OQH circuits [24]. Although the HL-LHC magnet protection is much more demanding than in the LHC magnets as concerning detection and actively induced quench propagation, the solution is very reliable.
In the various projects for the LHC luminosity upgrade, form LARP to HL-LHC, about 20 short model magnets and more than 10 long magnets have been manufactured and tested. The training to the nominal field takes 5 to 10 quenches, whereas the ultimate values, set at 8% above the nominal value, are reached with 10–20 quenches, which is almost three times more than for the LHC main dipoles. However, the training memory is very good, even better than LHC magnets made of ductile Nb-Ti. Once an ultimate quench value is reached, the first quench after a thermal cycle is usually consistently not below the last reached value which makes long magnet training acceptable.
The results of the most recent 11 T dipole magnets have shown that performance retention through powering and thermal cycles is still an issue. It is likely related to changes in the stress and strain state of the coil over the entire cycle of construction and operation. Indeed, this seems to be the main issue for both the 11 T dipoles and IT quadrupoles. This is a topic where future development is necessary. Also, operation at 1.9 K only yields a few % increase in quench current, well short of the expected 10% in accordance with critical current scaling. Although the benefits He-II are clear, and, in particular, the superior heat transport as well as the operating margin in a radiation environment, this choice could be questioned, especially for impregnated magnets for future developments.
A recent review of the design and performance of the Nb3Sn IR magnets for High Luminosity LHC can be found in [25].
3.2 Nb-Ti magnets
Besides the IT quadrupoles, the HL-LHC upgrade involves a significant change in the configuration of the whole magnetic system at the IR. A description of all these magnets and their performance can be found in [25].
The six resistive dipoles that separate the beams at the IT, just in front of the IT quadrupoles, with an aperture of 70 mm and a total length of 18 m are replaced by a new single Nb-Ti D1 magnet, 7 m long, 150 mm aperture, and generating a field of 6 T. The D1 magnets are being produced under the responsibility of KEK (Japan). The existing recombination dipole D2, with 90 mm aperture, is replaced by a two-in-one Nb-Ti dipole with two apertures of 105 mm. As the field in the two apertures in D2 has the same direction, causing strong iron saturation, this calls for an innovative design with a small left-right asymmetry in the coils to correct the field errors, similar to what was done in the single aperture J-PARC superconducting beam line for neutrino generation designed by KEK. The HL-LHC D2 magnets are produced under the responsibility of INFN (Italy).
A sizeable number of Nb-Ti corrector magnets of large aperture are also being designed and manufactured for HL-LHC, all with characteristics that will likely be used in future colliders. A very large, single aperture magnet combines vertical and horizontal dipole field corrector; it is built as two nested dipole coils of 150- and 200-mm inner diameter, each generating up to 4.5 Tm integral bending strength. These correctors are being produced under the responsibility of CIEMAT (Spain). The development of high order (HO) corrector magnets based on a Super Ferric (SF) design with 150 mm aperture, suitable for the generation of modest multipole field and compatible with a high radiation environment, is also under way. The HO correctors are being produced under the responsibility of INFN (Italy). A Canted Cos-Theta (CCT) dipole corrector magnet has been developed to be assembled in the cold mass of the D2 separation dipole, with an aperture of 105 mm, with a rated integral bending strength of 5 T.m. The CCT correctors are produced under the responsibility of IHEP (China).
4 Superconducting magnets for Electron-Ion Collider
Brookhaven National Laboratory (BNL) in the U.S. has started construction of the Electron-Ion Collider (EIC) with the c. o.m. Nominal energies within ∼20–100 GeV with a future upgrade to ∼140 GeV, and a luminosity of up to 1034 cm−2 s−1 for electron-proton collisions [26]. The decision to proceed with the project was taken in January 2020 with the goal to prepare it for commissioning and operation by the end of 2030. The EIC will advance the present particle colliders and enable the U.S. and international nuclear physics community to progress towards fundamental understanding of the nature of matter, providing a more clear picture of how quarks and gluons interact to form the basic structure of atoms and nuclei. The EIC design concept substantially leverages existing infrastructure from the RHIC accelerator complex at BNL. Nevertheless, significant new infrastructure has to be added to provide polarized electrons and strong hadron cooling.
All the magnets in the EIC electron storage ring (ESR) are water-cooled iron-dominated electromagnets. RHIC and its injector complex remain, for the most part, unchanged. The arcs with the Nb-Ti superconducting magnets are the same.
The EIC interaction region includes superconducting low-beta quadrupoles and spectrometer dipoles near the detector, and both normal-conducting and superconducting magnets for the matching section into the arcs of the electron and hadron storage rings, respectively. The IR layout is shown in Figure 4. It requires fifteen new unique superconducting magnets. Cross-sections of the key IR superconducting magnets are presented in Figure 5 and their parameters are summarized in Table 2. All the magnets are based on traditional Nb-Ti technology. Most of them operate at a temperature of 4.2 K, except for three magnets (Q1APF, Q1BPF and Q2PF) which due to the relatively high magnetic field need to be cooled to 1.9 K. Nine of the new superconducting magnets will be made with direct wind method and equipment previously developed at BNL [27]. This technology provides excellent field quality for a variety of magnets. Four magnets (B0aPF, Q1APF, Q1BPF, Q2PF and B1PF) are based on the collared cos-theta superconducting coils made of a Rutherford cable as the RHIC magnets.
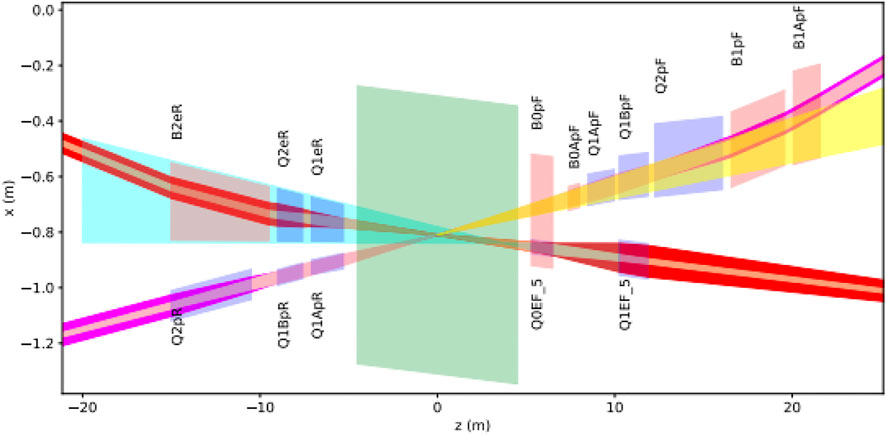
FIGURE 4. IR1 layout. The central detector is shown in light green, the hadron and electron beams are shown in magenta and light red respectively. Magnet apertures are shown in light red for dipoles or light blue for quadrupoles. The forward neutron cone is shown in yellow.
Some of the IR magnets are twin-aperture magnets with separate coils for the hadron and electron beams inside a common iron yoke. A main challenge for these magnets is the small crossing angle, which leads to a small separation of the magnet apertures. It is vital for this design to diminish magnetic crosstalk, which is achieved by using soft iron between the two apertures and avoiding its saturation. It is helpful in this context to taper some of these magnets, thus increasing the amount of iron between the apertures to minimize its saturation.
In tapered magnets without special provisions field components change along the magnet length. Since the gradient change is not desirable, a novel winding concept has been developed to address this problem [28]. The winding pattern which allows the coil radius to change while preserving the gradient is based on a coil concept known since the 1960s [29] as double-helix or canted cosine-theta coils. A unique feature of double-helix coils is in their flexibility that allows smoothly varying field components along the coil axis as needed. Tapered coils are foreseen for Q1ER, Q2ER, B2ER and Q1APR magnets. Practice tapered coils have been made using the direct wind method and tested to prove the concept.
Discussions in the nuclear physics community show that there is a significant interest in a complementary second IR. The complementarity is provided through the energy range where the second IR is optimized to have the highest luminosity and through the detection capabilities that are alternative and complementary to the first IR. Consistently with user needs and the complementarity approach, the second IR is optimized to provide the highest luminosity at a lower CM energy than the first IR. This enables leveling of the EIC luminosity curve over a wider energy range. The second IR can also provide a different acceptance coverage than the first IR.
Technical feasibility of all the second IR magnets is being studied. All the second IR magnets meet the nominal requirement of their fields being less than ∼5 T at the maximum beam energy. However, some of the quadrupole and dipole magnets may still present engineering challenges due to their large apertures. There are also certain advantages of increasing the magnetic field of some IR2 quadrupoles and dipoles to the level of 8–10 T which would require using brittle Nb3Sn superconductor. The stress and strain sensitivity of this superconductor in combination with high magnetic field and large coil aperture will require using stress management techniques [30, 31]. These approaches are being studied by the US-MDP (see section 6.2.1 below).
5 SC magnets for future hadron colliders
Two major hadron machines are under consideration–the Future Circular Collider (FCC) in Europe and the Super Proton-Proton Collider (SPPC) in China. Brief descriptions of machine and magnet parameters are presented below.
5.1 Future circular collider
The main goal of the FCC hadron collider, FCC-hh, is to advance the energy and intensity limits of particle colliders to search for new physics, aiming at proton collision c. o.m. Energies of 100 TeV, which is about seven times of those at the LHC. The hadron collider would be placed in a tunnel of approximately 100 km length that would initially host the lepton collider FCC-ee, the first step in the integrated FCC plan. Although the project is centered around CERN, an international collaboration of more than 150 universities, research institutions and industrial organizations from all over the world have joined in the development of this circular collider.
Superconducting magnets are among the grand challenges of the FCC-hh. Given the tunnel dimensions and the target beam energy, the arc dipoles, approximately 15 m long, need to generate 16 T in an aperture of 50 mm [32]. The present lattice design is based on the extrapolation of the LHC and HL-LHC lattices, and uses arc quadrupoles of 7.2 m length generating 360 T/m in the same bore as the dipoles [33], with peak field in the coil of about 10.5 T. The Interaction Region (IR) also calls for quadrupoles of high field gradient and large aperture, capable of withstanding the energy deposition and dose generated by the collision debris. The range of gradients in the IR quadrupoles is from 106 to 130 T/m, with respective apertures in the range of 210 to 164 mm, corresponding to a peak field in the coil of about 11 T.
The values of peak field have been the motivation for choosing Nb3Sn as the baseline superconductor for the majority of the FCC-hh magnets, as for the HL-LHC IR quadrupoles and 11 T dipoles. Still, this is a significant extrapolation of magnet technology beyond the work presently on-going for the HL-LHC, and especially for the dipoles. The peak field in the quadrupoles is close to the values of the HL-LHC IR quadrupoles, but larger apertures imply larger forces and stresses, thus exacerbating the issues of coil mechanical support, not to mention the increasing difficulty of magnet protection. For the dipoles, on the other hand, achieving a 16 T bore field at operation implies significant development of conductor [34], as well as magnet design and technology [35].
The most relevant advancement within the FCC magnet R&D program was brought by the exploratory effort to identify the magnet design with best properties in terms of performance, efficiency of material usage and cost. Four coil design layouts were examined in detail: cos-theta at INFN, block-type at CEA, common-coil at CIEMAT, canted cos-theta at PSI (Figure 6). A key aspect of this work is that all designs were produced using the same conductor parameters such as the critical current density Jc of 1500 A/mm2 at 4.2 K and 16 T consistent with the conductor R&D target, the copper matrix RRR of 100, the maximum strand diameter of 1.2 mm, and the minimum Cu/nonCu ratio of 0.8:1. A critical assumption was to take the operation margin of 14% along the load-line which is below the value assumed for HL-LHC. A nominal field in aperture of 16 T corresponds to a short sample bore field of 18.6 T. For the quench protection parameters, the assumptions were an allowable time window for detection and quench initiation (i.e. the time it takes for the quench heater or CLIQ to induce a widespread transition) of 40 ms, maximum hot spot temperature of 350 K, and maximum voltage to ground of 2.5 kV. Finally, the four designs were developed using identical coil mechanical properties and, in general, the parameters of the structural materials used in the magnet.
The main results of this study are that compact configurations, based on cos-theta and block coils, are very close to each other in terms of operating margin and material cost. This is because the ratio of coil width to diameter is relatively large. Their stress distribution is very different, though. While cos-theta coils have peak stress at high field locations, at the midplane, in block coils the peak stress is generally located in moderate field region, on the outer boundary, which may be an advantage given the strain sensitivity of Nb3Sn. Common coils appear to offer benefits of simpler end geometry, and a definite premium if a react-and-wind manufacturing procedure can be proven. The price is an increase of material, and corresponding cost, by about 20%. The canted-cos-theta is in a similar situation, offering stress management (not yet fully proved) at the price of increased material cost, in this case of about 25%.
The second major advance of the magnet R&D driven by FCC-hh is the construction of small-scale demonstration magnets to explore performance boundaries. Two configurations were produced and tested within the scope of the FCC magnet R&D program called the extended Racetrack Model Coil (eRMC), and Racetrack Model Magnet (RMM). These magnet models are assembled out of racetrack coils wound with cables of size representative of the FCC-hh magnet design, although at reduced performance. eRMC and RMM have shown that in this simplified configuration a Nb3Sn coil can achieve operating fields in the range of 16 T, as discussed later.
5.2 Super proton-proton collider
To achieve the 75–150 TeV c. o.m. Energy, SPPC needs thousands of accelerator magnets with nominal magnetic fields within 12–24 T and apertures of 40–50 mm to guide and focus the high-energy proton beams. These magnets have to provide the field uniformity on the level of 10−4 in ∼65% of their aperture. As the LHC magnets, these magnets have two separate apertures with opposite field direction inside the common iron yoke to minimize the magnet transverse size and reduce its cost. The aperture separation in the main dipoles is presently estimated on the level of 200–300 mm. In the final magnet design this parameter will be optimized to achieve the acceptable crosstalk between two apertures and minimize the overall magnet cross-section. The outer diameter of the arc dipole and quadrupole cold masses is limited by 900 mm to be installed inside vacuum vessels with an outer diameter of 1.5 m. The total magnetic length of the main dipoles is around 65.4 km out of the total collider ring circumference of 100 km. For the dipole length of about 15 m, approximately 4,360 dipole magnets will be needed [36, 37].
SPPC magnets with magnetic fields up to 15–16 T consider using advanced Nb3Sn superconductors. To provide nominal operation fields up to 24 T High Temperature Superconducting materials are needed. These materials should have acceptable cost and be capable of operating in high fields at large mechanical stresses. Special attention at the present time is being paid to Iron Based Superconductors (IBS) discovered in 2008 and promising high Jc at high fields.
Conceptual design studies of twin-aperture 12 T dipole magnets for the SPPC based on the IBS technology are being performed to achieve the SPPC target parameters. The design studies are based on the high level of current density, about 10 times higher than its present level, assuming that it will be achieved within the next 10 years. In addition to the significant increase of the current carrying capability, it is also anticipated that the IBS will have better mechanical properties than the present high field superconductors such as Nb3Sn, REBCO or Bi2212, and substantially lower cost.
Cross-sections of the two design options under consideration for the 12 T SPPC dipole based on the IBS conductor are shown in Figure 7. The coil aperture in both cases is 45 mm and the nominal field in the two magnet apertures is 12 T with the relative geometrical field errors smaller than 10−4. The coil layout uses the common-coil configuration due to its simple structure compatible with the tape-type conductor and simplicity for fabrication. Two types of coil ends based on soft-way bending and hard-way bending are being studied and compared with respect to the field quality and structure design and parameters. The magnet design details and parameters are reported in [38].
5.3 High energy muon collider
Muon colliders (MC) have emerged in the past few years as potential game changers, with the promise of unique opportunities for particle physics applications. They can offer various options and possibly a staged approach towards the next step in HEP. The main difficulty of muon colliders is the short muon lifetime of 2.2 μs, which, however, increases thanks to relativistic dilation when muons are accelerated to high energies. The decay of a muon beam within a storage ring can yield pure, intense, and well controlled beams of neutrinos for neutrino-oscillation studies on short- and long-baseline. The muon mass is approximately 207 times larger than that of electrons and positrons, hence, the beams are not subject to the stringent synchrotron radiation and bremsstrahlung limits of e-p colliders. Therefore, in principle muon beams can be accelerated to high energies, in circular colliders and stored in rings for a time sufficiently long to produce high luminosity collisions. Indeed, TeV-class muon colliders are considered as the most effective path for a high-luminosity lepton collider.
The concept of the muon collider was first proposed by G.I. Budker in 1969 [39]. Since then, innovative MC concepts have been developed in the framework of several design studies and R&D programs in the U.S., such as the Neutrino Factory and Muon Collider Collaboration (NFMCC) [40] followed by the Muon Accelerator Program (MAP) [41], and now the work will continue by the recently-created International Muon Collider Collaboration (IMCC) [42] to form the foundation for the next steps of High Energy Physics.
The muon collider requires several types of superconducting magnets with various geometries, apertures, field level and configuration, powering mode, etc. To provide the required field parameters in each area. Concepts for MC solenoids, devised for muon production and cooling, and dipole and quadrupoles concepts, developed for muon acceleration, storage, and final focus, are shown in Figures 8, 9.
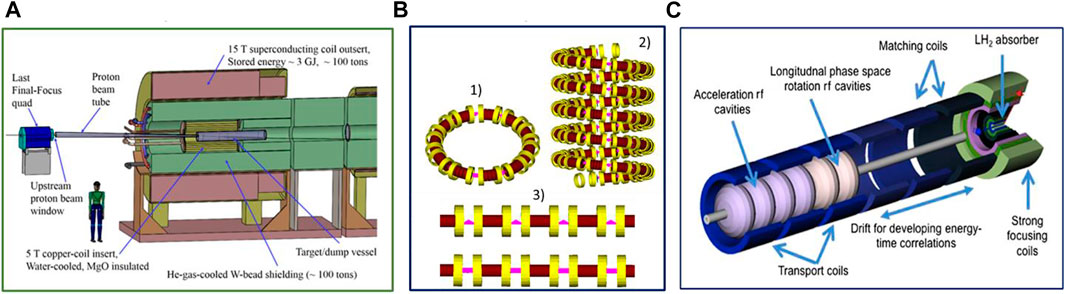
FIGURE 8. MC solenoid concepts: (A) muon production solenoid; (B) various possible solenoid configurations in 6D muon cooling system; (C) final cooling system with high-field solenoids.
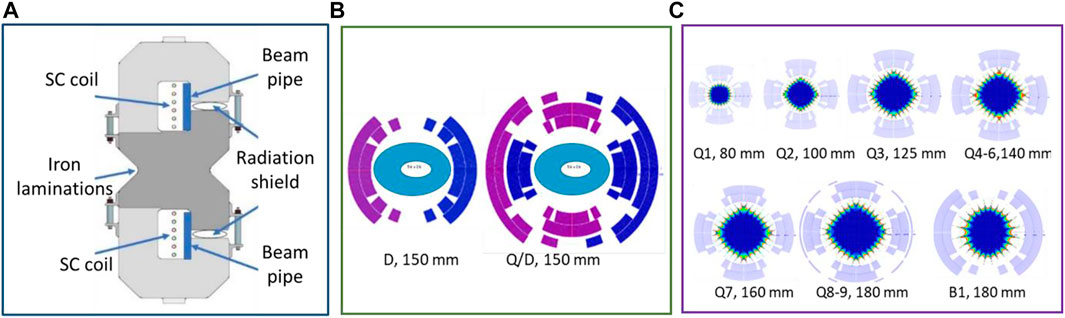
FIGURE 9. MC acceleration and SR magnets: (A) fast-cycling super-ferric dipole magnet with HTS coil, (B) collider dipole and combined function dipole-quadrupole magnets with thick elliptical absorbers in the apertures, (C) IR quadrupole and dipole magnets (good field quality areas in the apertures are shown in blue color).
The MC target area and the front end consist of a series of large-aperture high-field solenoids [43]. The MAP study has devised a target area consisting of a 20 T solenoid which is composed of an assembly of a 15 T superconducting outsert with 2 m aperture and a 5 T normal conducting insert with 0.3 m aperture. This high-field solenoid captures the pions produced by the impact of an intense proton beam on a target (Figure 8A). It followed by the 12 m long decay channel made of solenoids with tapered apertures from 2 m to ∼0.6 m and field from 20 T to ∼2 T. Based on the field level, the outer superconducting part of capture solenoid and the decay channel solenoids have been designed using Nb3Sn and Nb-Ti coils.
Then the muon beam enters the beam cooling system which consists of six-dimensional (6D) cooling channel and the final cooling stage. In this system the 6D phase space volume of muon beams is reduced before further acceleration and injection into the storage ring. Several designs of the 6D cooling channel have been proposed and studies [44] (Figure 8B). In the 6D beam cooling area, large-aperture 10–20 T solenoids with normal RF cavities inside or in-between are considered. The field level in the 6D cooling solenoids can be achieved using contemporary Nb-Ti or Nb3Sn superconductors. The final cooling stage (Figure 8C) consists of a series of 50-mm aperture solenoids with high magnetic field ideally in the range of 50–60 T [45]. These final cooling solenoids could be devised based on experience with the record field 32 T hybrid solenoid at NHMFL and high-field solenoids in Europe, which are using hybrid coils with HTS inner and LTS outer sections.
The accelerator concept is based on a sequence of linacs, recirculating linacs and rapid cycling synchrotrons (RCS). The RCS are designed as hybrid system, consisting in interleaved resistive fast-cycling magnets [46], and high-field steady-state superconducting magnets. The advantage of this configuration is that it reduces the length of the RCS and, thus, the time lost accelerating the muon beams. In the first RCS, the fast-ramping dipole magnets for the muon acceleration has to provide a field variation within ±2 T during 0.4 ms in a rectangular aperture of 80 mm by 40 mm, which corresponds to a field ramp rate of 10 kT/s. These magnets will use traditional fast ramping magnet technology based on resistive coils and warm iron yoke. The last RCS requires a similar swing of ±2 T in about 10 ms, or a field ramp rate of 400 T/s. Higher field swing would be welcome. These magnets can use superconducting coils (Figure 9A).
The last major part of the MC complex is the collider Storage Ring (SR) and Interaction Region (IR). A specific feature of a muon collider is the neutrino flux resulting from muon decay, taking place continuously along the storage ring. One of the measures to reduce the resulting radiation flux is to use combined function magnets in the arc, thus avoiding straight sections as would be the case if separate arc quadrupoles were used. The MAP baseline 3 TeV collider is based on 150-mm aperture dipoles and combined function magnets with a nominal dipole field of 10.5 T and a field gradient of 85 T/m in the arc [47]. The IR uses focusing quadrupoles with field gradients up to 250 T/m and apertures from 80 to 180 mm, and 180-mm aperture 8 T dipoles [48]. Magnet protection from radiation in the collider ring arc is achieved by using thick Tungsten absorbers in magnet apertures and by masks in between magnets. The cross-sections of collider ring dipoles and combined quadrupole/dipole magnets with inner absorbers, and IR quadrupoles and dipoles designed within the scope of the MAP study are shown in Figures 9B,C. All the magnets are based on Nb3Sn superconductor operating at 4.5 K which provide sufficient temperature margin for the radiation heat load.
A 6 TeV IR design, and on-going studies for a MC at 10 TeV, assume HTS technology to achieve nominal operation fields up to 20 T, and possibly a further increase of magnet aperture to accommodate for even larger shielding. Hybrid HTS/LTS coils with larger operating fields and margins as well as curved magnets are also being considered.
6 Superconducting accelerator magnet R&D
6.1 Achievements
Having reached the limit of Nb-Ti magnet technology, all the above projects and studies have been turning towards advanced superconducting materials and novel magnet technologies, targeting increasing fields. The main motivations for a long-term program are the following:
- Development of high-field magnets is an activity with long lead times. Time scales up to a decade are required to master new technology and bring novel ideas into application. This stresses the importance that R&D runs in parallel with the study of new accelerator concepts and options, so that specific magnet technology is available for a HEP realization, anticipating the demand for the moment when the decision of construction is taken.
- SC magnet technology for future HEP colliders, in particular high field magnets, requires large scale infrastructure and considerable investment. Best use of such infrastructure is made if an R&D program is run as a continuous progression.
- Similar to the research infrastructure, the development of high field magnets requires stable proficient teams with broad competencies, spanning over many fields of science, often assembled in collaborations ranging from academia to industry. Building one such research team needs considerable investment, and continuity is an asset.
The present state-of-the-art in high-field magnets for accelerators is the result of a coordinated efforts that has spanned the past 30 years. The largest effort was put in the development of Nb3Sn conductor and magnet technology. A strong focus was provided by the US-DOE programs devoted to Nb3Sn conductor and magnet development, initiated at the end of 1990’s. The resulting program, a broad collaboration among the US-DOE accelerator Laboratories and associated Institutions, is now continuing under the auspices of the U.S. Magnet Development Program [49, 50]. European-wide activities in high-field accelerator magnets took form under the EU-FP6 initiative for Coordinated Accelerator Research in Europe (CARE) [51]. The Next European Dipole Joint Research Activity (NED-JRA) [52], which enfolded from 2004 to 2009, was followed by the EU-FP7 EuCARD [53]. The above activities were instrumental to the development of the HL-LHC Nb3Sn magnet technology, presently used in the QXF quadrupoles and 11 T dipoles described earlier, and demonstrating the importance of a continuous R&D.
In parallel, the interest in the exceptional high-field potential of High-Temperature Superconductors (HTS) has spurred much excitement for accelerator magnets. Although copper oxide compounds containing rare-earths (REBCO) and bismuth (BSCCO) are only in a stage of early technical maturity, laboratories and industry have shown that HTS are capable to produce fields in excess of 28 T in commercial NMR solenoids to record values of 45.5 T in small experimental solenoids. This is an area where we expect to see fast progress. Activities are in various EU laboratories are following the program initiated with the EuCARD [53] and EuCARD2 [54] collaborations, now fostered by the on-going I-FAST [55] EU project. In the US, HTS accelerator magnet development is an integral part of the US-MDP.
6.1.1 Nb3Sn magnets
Progress in the development of Nb3Sn magnets for accelerators can be appreciated by the suggestive presentation in Figure 10, where the steady increase of field produced by dipole magnets over the past 4 decades is shown. The plot contains results from short demonstrator magnets, built with racetrack coils with the main purpose to test field reach, short model magnets with most final features but reduced length in the range of one to 2 m, as well as full-size accelerator magnets containing all final features for operation in an accelerator.
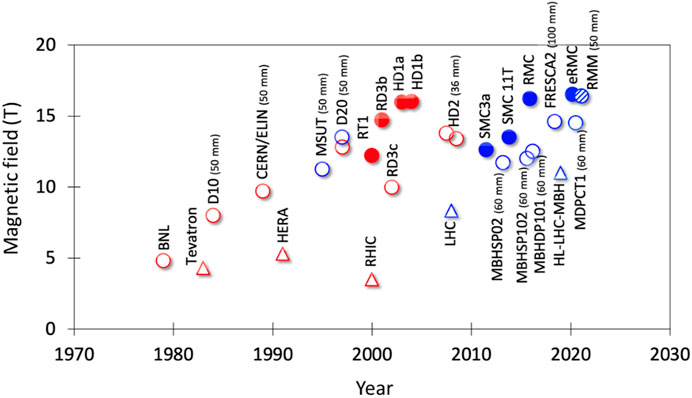
FIGURE 10. Progression of fields reached by Nb3Sn dipole magnets of various configurations and dimensions. Open symbols represent magnets with a free bore, solid symbols are for demonstrators built with racetracks and no free bore. The hatched symbol (RMM) is a magnet with a free cavity. Tests were performed in liquid helium (4.2 K, red symbols) or superfluid helium (1.9 K, blue symbols). Superconducting collider dipole magnets are shown as triangles for comparison.
The first significant results can be traced back to the 1980’s, at BNL and LBNL [56]. This work eventually led to the achievements of D20 in the 1990’s, and the 16 T field attained with the demonstrator HD1 at LBNL in the 2000’s. Fields in the same range were reached at CERN in 2015 and exceeded as of 2020 as a result of the push provided by FCC-hh. As we mentioned earlier, work in the 1990’s and 2000’s has laid the foundations for the HL-LHC Nb3Sn magnets. And yet, the R&D program itself was largely funded by HEP in the US, as well as initiatives in Europe, i.e., essentially independent of a specific HEP project.
Figure 10 demonstrates that the timeline for progress in Nb3Sn magnet technology is slow. It took about a decade for CERN and collaborators to reproduce the results obtained in the U.S., going from conductor R&D to the 16.2 T dipole field obtained with RMC03 in September 2015. This gives a good benchmark for the time scale necessary to enter into the field, including the required infrastructure as well as design and manufacturing skills. The final result of this work is the record magnet FRESCA2, built in collaboration between CERN and CEA, and generating a field of 14.6 T in an aperture of 100 mm diameter [57]. This field level has been achieved also at FNAL by a high-field model dipole built within the scope of the US-MDP program [58] as a step towards the highest field that can be attained with a cos-theta coil configuration. Figure 11 shows the 100-mm aperture block-type FRESCA2 and 60-mm aperture cos-theta MDPCT1 14.6 T record dipole magnets.
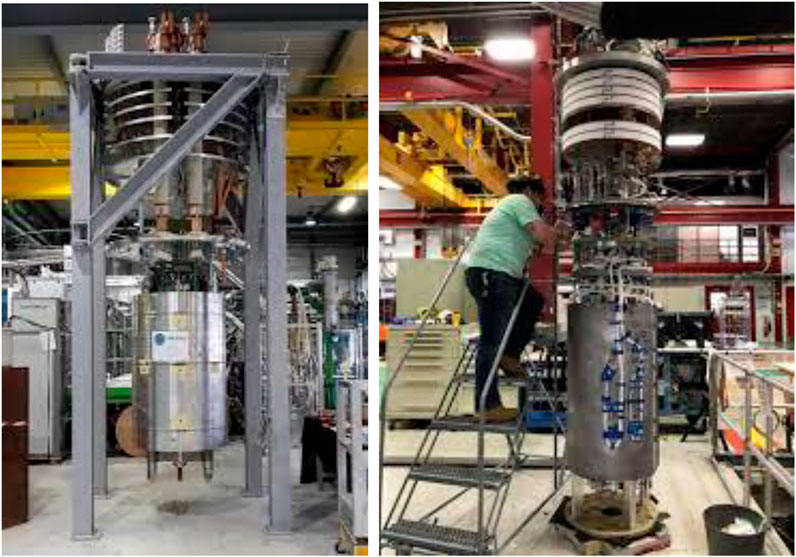
FIGURE 11. The 14.6 T record dipole magnets: 100-mm aperture block-type FRESCA2 (left) and 60-mm aperture cos-theta MDPCT1.
The progression is not yet complete, as demonstrated by the last point in the plot, corresponding to the 16.4 T reached at CERN in 2021 in a special Racetrack Model Magnet or RMM. RMM reproduces the 2D cross-section of a block-coil magnet but has flat ends. It results in a cavity in the center of the magnet, which is the case of the RMM is 50 mm, the nominal aperture for FCC-hh.
Figure 10 also shows the remarkable achievement of the Nb3Sn MBH 11 T dipole for HL-LHC. This dipole was built at CERN in collaboration with industry (GE-Alstom) [59]. Also here, we remark that the time scale of the project, from inception in 2010 to the test of the first accelerator unit in 2019, is a decade. The first such magnet, MBHB002, was tested in July 2019 and also detains the performance record for its class. As we mentioned earlier, the 11 T dipole program has pointed out that there are still questions to be resolved beyond magnet performance, on long-term reliability and robustness. These questions are being addressed so that Nb3Sn can be used in an operating accelerator.
6.1.2 HTS magnets
HTS accelerator magnets are expected to be the next step in SC accelerator magnet technology. As reported in the graph of Figure 12, the HTS technology is making it first trials for accelerator-class magnets.
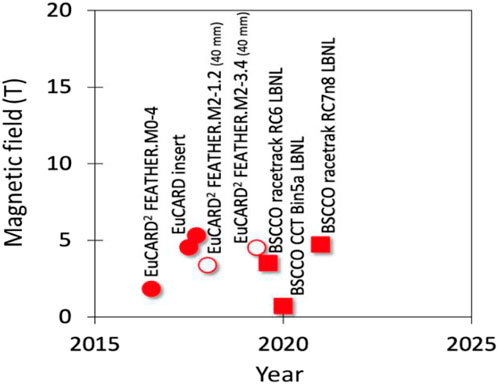
FIGURE 12. Maximum fields obtained with demonstrator HTS short magnets, producing a transverse field of dipole type. All reported maximum value were reached in liquid helium (4.2 K). Solid symbols are coils with no bore (e.g., racetracks), while open symbols are real magnets, i.e., with a usable bore. Round symbols refer to coils built with REBCO, while square symbols refer to BSCCO-2212 coils.
The general interest in the potential of this class of material with spectacular performance coagulated at about the same time in the mid of the 2000’s in the U.S. and Europe. On the U.S. side, efforts were coordinated by the US-DOE sponsored Very High Field Superconducting Magnet Collaboration [60], which targeted BSCCO-2212 as HTS conductor suitable for high field. This activity is part of the scope of the US-MDP [61] now addressing both BSCCO-2212 and REBCO in various cables (Rutherford and CORC™) and magnet (racetracks, cos-theta and canted cos-theta) configurations. As anticipated, in the EU the first seeds initiated already with the EU-FP7 EuCARD collaboration and were pursued intensely with the follow-up EU-FP7 EuCARD2 [62] and EU-H2020 ARIES [63] programs.
In Europe the HTS conductor activity was directed to REBCO, whose selection was mainly driven by the perceived potential and by consideration of relatively easy coil fabrication technology with a conductor that does need heat treatment of the whole coil [64]. After the attempt of flat racetrack with simple stacked tape with a fairly good amount of copper as used in EuCARD insert, EuCARD2 had the ambition to make to test HTS in a real accelerator magnet configuration. So EuCARD2 dipole magnets were designed with an open bore and wound with a cable. A multi-tape cable, rather than a simple tape, was selected as conductor, in order to operate the magnet in the10 kA range, very much like the usual LTS high field dipoles, to limit inductance to cope with quench protection and in order to profit of current sharing between cable sub-elements. In addition, transposed topology for the cable has been used, ruling out the use of simple stacked tape [54]. This allowed to explore the potential of Roebel cable whose potential for HTS tape composed cable was just being demonstrated [65]. The result of these activities are small demonstrator magnets. A simple flat coil, M0-4 was wound and assembled to check windability and behavior of Roebel cable. It reached almost 13 kA at the minimum temperature of 15 K (test at higher amperage was not possible due to limitation of the test station). Then two real 35 mm open bore (coil gap of 40 mm) dipoles, M2-1-2 and M2-3-4, were manufactured that reached 3.5 T and 4.5 T, respectively, at 5 K in stand-alone mode [66]. This last result is a very encouraging figure, just 10% short of the design field of 5 T, probably due to damage to the leads outside the coils. However, the use of Roebel cable proved to be cumbersome and the use of coil technology with non-insulated conductor, as well as new considerations on possible futility of transposition with HTS tapes, open the way to a single tape or a stacked tape cable as conductor for magnet winding. This might change completely the direction to go.
Meanwhile at LBNL in the U.S., two lines continue to be developed. The first one is pursuing BSCCO-2212 conductor in form of Rutherford cable, first with race-track coil geometry and then with CCT coil geometry [67]. The other line is pursuing REBCO round cable (so-called CORC™) in a CCT layout coil [68]. Recently R&D activities on the HTS insert coils have started also at FNAL. The work is focused on both REBCO [69] and BSCCO-2212 [70] conductor and shell-type coils with stress management. The R&D is progressing steadily, though slowly, with results similar to those achieved in Europe. Figures 13, 14 show the cables, coil cross-section and pictures of these small-scale pioneering HTS dipole coils. As mentioned above, the collection of the main results recently achieved in Europe and the U.S. in Fig. 6.1.2-1 shows only the initial part of a path that will hopefully lead to similar, or even better, results obtained for Nb3Sn.
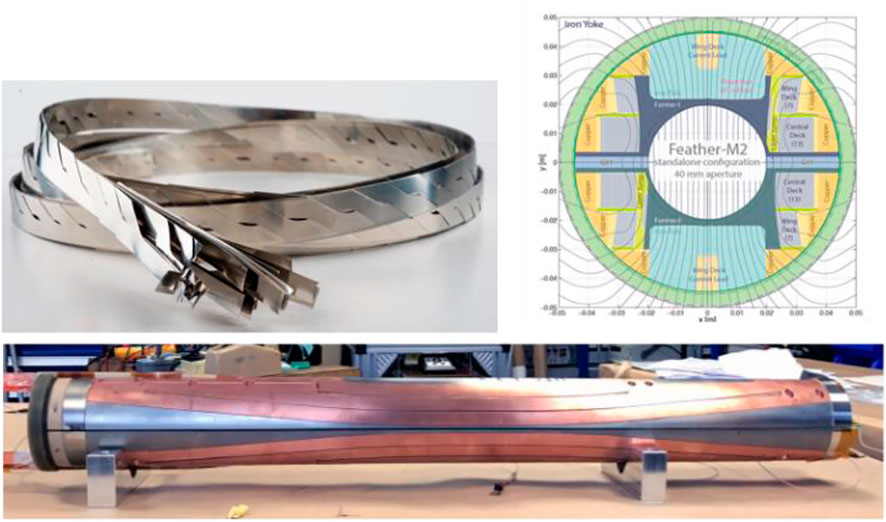
FIGURE 13. EuCARD2 Roebel cable (top left), aligned block dipole sketch (top right), and first real insert coil before test at CERN (bottom).
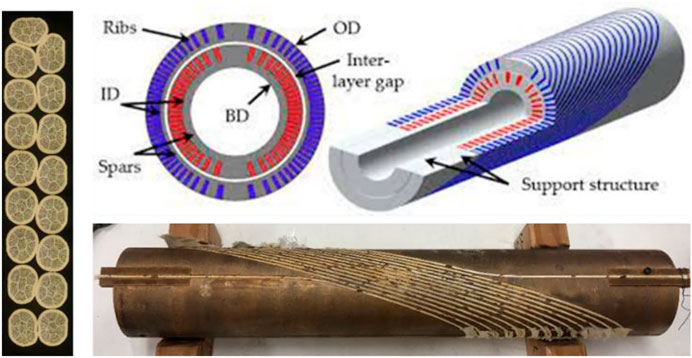
FIGURE 14. BSCCO-2212 Rutherford cable (side left), CCT coil cross-section and 3D view (top right), and initial CCT BSCCO-2212 insert coil at LBNL (bottom right).
The next step beyond the further advance of the HTS conductor and coils technology is to study the insertion of these small-size demonstrators in large bore, LTS background magnets. This is to increase the central field and demonstrate the ability to break the barrier of LTS magnet performance, while at the same time exploring this new range of field. One EuCARD2 dipole, after an attempt to be powered in a background field underwent a severe degradation, whose origin is not yet clear.
6.3 Regional magnet development programs
6.3.1 US magnet development program
The US Magnet Development Program (MDP), formed by the US DOE Office of High Energy Physics in 2015 [49], following the 2013 “Snowmass” decadal community planning process, is composed of researchers from leading DOE Laboratories and Universities focused on developing accelerator magnets for colliders.
The overarching goals of the program include 1) exploration the performance limits of Nb3Sn accelerator magnets, focusing on minimizing the required operating margin and significantly reducing or eliminating magnet training; 2) development and demonstration of HTS accelerator magnets with a self-field of 5 T or greater, compatible with operation in a hybrid HTS/LTS magnet for operation fields beyond 16 T; 3) investigation of fundamental aspects of magnet design and technology that can lead to substantial performance improvements and magnet cost reduction; and 4) performing Nb3Sn and HTS conductor R&D with clear targets of increasing performance, understanding present performance limits, and reducing the cost of accelerator magnets.
There are many paths and opportunities for high-field accelerator magnet development. To provide focus, the MDP has centered its current research around specific themes. First, the program explores stress-managed structures as a means to enable high-field accelerator magnets. By intercepting the large azimuthal and radial forces that accumulate in the magnet midplane area in conventional accelerator magnet designs, the stress-managed structures have the potential to mitigate degradation to the strain-sensitive Nb3Sn and HTS superconductors in high-field environments. Second, it explores the use of hybrid HTS/LTS magnets for high field accelerator magnets. The use of HTS opens a path to high fields beyond that achievable with LTS materials, and by using LTS material in the low-field region the technology seeks to optimize the cost and size of the overall system. Third, it strives to improve our understanding and control of magnet technology and magnet performance for accelerators through the development of advanced diagnostics and modeling tools. Integrating these into the magnet design and testing provides critical feedback for further improvements and refinement, mitigating risk and ultimately leading to more cost-effective magnet. Fourth, to inform further promising avenues for magnet development, design studies are underway on high-field accelerator magnet concepts. Finally, in support of the high-field magnet needs, the program seeks to further develop superconductors, focusing on improving characteristics critical to accelerator applications such as enhanced transport current, improved production quality, and ultimately a reduction in material cost.
The themes described above are aligned with the goals established when the MDP was founded in 2016. The program has made progress towards its goals in advancing superconductors, developing core HTS magnet technologies, and demonstrating record Nb3Sn accelerator magnet performance. The MDP roadmap has been updated in 2020, the updated plan is detailed in [50]. The program is structured around the themes described above, and has four components: Nb3Sn magnets, HTS magnets, technology, and SC materials.
The development of Nb3Sn magnet technology is a core element of the program. The successful four-layer cosine-theta magnet [71], designed and built by the FNAL team as part of the MDP, serves as the foundation and motivation for an effort to investigate and develop stress-managed magnet concepts, where the magnetic body forces (Lorentz forces) - that in traditional magnet designs accumulate in the dipole midplane area - are instead captured locally and transmitted to a mechanical structure. Two complementary approaches to stress-management are being pursued. The first, known as the Canted Cos-Theta (CCT) [72], is effectively the limiting case, where each turn in the coil is captured and in principle no force accumulation occurs. The second, known as the stress-managed cosine-theta (SMCT), is somewhat similar to a traditional cosine-theta dipole layout, but where the magnetic force acting on groups of turns are transferred to a support structure, leading to a more efficient use of conductor as compared to the CCT. The CCT approach is currently under rapid development using subscale prototypes that allow for systematic development and testing of design details. Similarly, the SMCT concept is being developed using the mirror-structure concept, which enables a single coil to be tested in a realistic magnet environment for more rapid development of the concept. By pursuing both concepts in parallel, the program can most efficiently explore the potential of stress-managed structures for accelerator magnet application. We note that in traditional dipole magnet designs the mechanical stress scales with field and radius; the stress-managed structures seek to break that scaling, enabling access to higher magnetic field and to larger bore magnets.
A second major thrust of the MDP is the development of HTS magnet technology. The program is pursuing the use of both REBCO and BSCCO-2212; each has advantages and disadvantages. Since both materials are strain-sensitive and prone to damage under mechanical stress, the stress-managed mechanical concepts described above are highly relevant and are being adapted and applied for the HTS magnets. Significant progress has been made over the last few years, both in developing the basic HTS magnet technology and in fabricating and testing prototype HTS dipole magnets, and the program expects to achieve a 5 T stand-alone HTS dipole demonstration in the near future. In parallel, the program is preparing for first tests of hybrid magnets wherein an HTS insert dipole is positioned inside a Nb3Sn outsert. Such an approach is anticipated to be the most efficient and effective means of achieving dipole fields above 16 T. To accomplish these goals, the MDP leverages the expertise and facilities available at all the partner institutions.
A third major element of the program is the development of fundamental magnet technologies, encompassing critical elements such as advanced modeling techniques, novel diagnostics, the exploration and characterization of novel magnet materials, and new data analysis techniques. Tightly integrated with the first two thrusts of the program, this element is central to advancing our communities understanding of magnet behavior and performance limitations. This knowledge is used to explore future paths for the program, and in particular is being applied to scope out paths towards ∼20 T hybrid magnet designs.
Finally, at the heart of high field accelerator magnets is the superconductor. The MDP invests in commercial conductors, working closely with industry to make sure the material properties are matched to accelerator magnet needs. Investments are also made in conductor R&D, where new superconductor architectures tailored to the accelerator magnet communities’ needs are explored. The development of new BSCCO-2212 powder manufacturing, which, when coupled with overpressure-processing, has led to dramatic increase in overall current density achieved in BSCCO-2212 magnets over the last decade, is one example. Similarly, reductions in REBCO substrate thickness, coupled with improved pinning via Zr doping, has led to major advances in REBCO cable performance. In the Nb3Sn realm, novel concepts that introduce vortex-pinning enhancement through doping with ZrO2 particles and/or Hf [73] have led to significant increases in current density at high fields, further expanding the potential reach of Nb3Sn. There have also been very intriguing developments in the use of high heat capacity (“high-Cp”) materials either internal to Nb3Sn wires [74] or as part of the cable [75], with the potential to significantly improve Nb3Sn magnet training.
A 10-year roadmap for the US-MDP aligned with the US High Energy Physics community planning process is shown in Figure 15.
6.3.2 European high field magnet program
Present and future demands from HEP, as discussed above, were included in the process of updating the European Strategy for Particle Physics (ESPP). The ESPP consultation and synthesis process started with the Open Symposium of Granada in May 2019 and completed with the endorsement of the ESPP update by the CERN Council, in June 2020 [76, 77]. The references quoted contain strong statements supporting R&D activities on high-field accelerator magnets. The above statements have been translated in the two long-term technical goals of the HFM R&D program:
Goal 1. The search for the maximum practical operating field that can be separated from the development of accelerator technology, defining two sub-goals:
I. Quantify and demonstrate Nb3Sn ultimate field, with a projected target of 16 T, developing conductor and magnet technology towards ultimate Nb3Sn performance. The target field may be revised based on the performance of demonstrators and model magnets, as well as practical considerations of operating margin and cost.
II. Develop Nb3Sn magnet technology for a large-scale production, simplifying manufacturing and striving for cost reduction. This is done taking HL-LHC with an ultimate field in the range of 12 T and a relatively small production as suitable benchmark. Nb3Sn magnets of this class should be robust from manufacturing, through test, installation and commissioning up to accelerator operation. This development will be measured against the performance of long demonstrator magnets.
Goal 2. Develop HTS magnet technology for accelerators, providing a proof-of-principle of beyond the reach of Nb3Sn. This program breaks the evolutionary changes of LTS magnet technology, calling for a number of significant innovations. The target dipole field is set for 20 T, well above the reach of Nb3Sn. HTS should be considered for applications where not only high field is sought, but also higher operating temperature than liquid helium, large operating margin, and radiation tolerance. Finally, for HTS the possibility of full HTS magnets to operate the accelerator at 14–16 T, i.e., the same field that Nb3Sn, but at much higher temperature of 15–20 K will be explored. The main driver of this study is the possibility to operate the full accelerator at higher temperature with significant saving factor on the electric power needed by cryogenics.
A suggestive graphical representation of the main objectives above is shown in Figure 16, where the total length of magnets produced with a certain technology plotted vs. the maximum field reached. Note that the HL-LHC quadrupoles QXF are included in the plot. The line passing through the points on the plot defines a boundary of the state-of-the-art and goes from the large-scale end of the nearly 20 km of Nb-Ti LHC double-aperture magnets in the range of 9 T ultimate field, to the high-field end of single model magnets, each about 1 m long, and reaching 14.5 T maximum field. The HL-LHC magnets represent intermediate field, about 12 T, and total length scale in the range of 100 m. The objectives of the European HFM R&D program correspond to an extension of the field reach by moving the boundary along the horizontal axis (magnetic field), profiting from advances in both Nb3Sn and HTS magnet technology, and an extension of the production capability by moving the boundary along the vertical axis (magnet length), through the development of robust design and manufacturing processes.
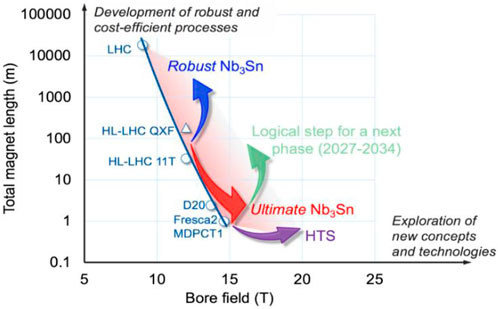
FIGURE 16. Schematic representation of the objectives of the European HFM R&D program, intended to advance in synchronism on the front of maximum field (red for Nb3Sn, purple for HTS) as well as large-scale production (blue). The possible evolution for the longer term is represented in green.
The target represented by the graphical representation of Figure 16 only defines a first step in the R&D, which should enfold in the 2021-2027 period. The parallelism in the development is necessary to provide significant advances within 5 years and feed the discussion for the next iteration of the European Strategy for Particle Physics with crucial deliverables. Advancing on both fronts of maximum field and large-scale capability will provide options for a decision on magnet technology towards the next hadron collider. A follow-up can already be imagined, depending on the successful outcome of the first phase. This should be dedicated to prototyping the new generation of high field magnets. This is represented by the green arrow in Figure 16, although the choice of the field level and magnet length to be realized are only indicative, and will depend on the results of the first phase of the R&D.
The R&D targets formulated above respond directly to the demands of the principal stakeholders. For Nb3Sn magnets, the target field and large-scale capability is driven by the demands of an FCC-hh [78], and the staged approach is compatible with the allotted development time of the integrated FCC program [79].
Even if the main focus of the European HFM R&D is on dipole magnets for an FCC-hh, an R&D on high-field Nb3Sn and HTS magnets along the lines outlined above will be highly relevant to develop suitable design and technology solutions also for other collider options, and in particular a muon collider. Specific examples are HTS conductor and coil winding technology, exploring partial- and no-insulation windings, relevant for the target and cooling solenoids, and the study of stress management in Nb3Sn magnets, with impact on the muon collider ring and IR magnets. Also, very important is considering HTS magnet operation at temperatures above liquid helium, which is relevant to understanding the operating margin in the high heat load and radiation environment of a high-energy muon collider ring.
The structure of the present European program towards high-field superconducting accelerator magnets, fruit of the consultation process driven by the LDG [80] is represented graphically in Figure 17. The work is divided in three focus areas, in foreground, supported by three cross-cutting R&D activities, in the background. The focus areas are: 1) Nb3Sn magnets; 2) HTS magnets; and 3) Nb3Sn and HTS conductors. Activities in the focus areas will directly provide elements for critical decisions for the field reach of a given magnet technology or for superconductor performance specifications. The cross-cutting R&D activities are grouped into 1) structural and composite materials, cryogenics and thermal management, and modeling; 2) powering and protection; and 3) infrastructure for production and test as well as instruments for diagnostics and measurement. These activities are intended to provide the background research needed to respond to the challenges identified in the focus areas.
6.3.3 Muon collider driven magnet R&D
The updated European Strategy for Particle Physics, produced by the Laboratory Directors Group (LDG) under the auspices of the CERN Council, includes a strong recommendation towards the development of muon beams, in particular in view of a high-energy, high luminosity Muon Collider. The focus is on two energy ranges, around 3 TeV and above 10 TeV. The International Muon Collider Collaboration (IMCC) is focusing on the development of a Muon Collider design and address the associated technical challenges. The collaboration aims at establishing feasibility of a muon collider to develop the concept and technology to a level of maturity that allows committing to its construction and to assess the physics reach.
The full conceptual design of a Muon Collider poses grand challenges and is expected to demand a considerable effort. In particular, the challenges for the key magnet systems of the complex are well beyond the reach of present technology. New concepts, demonstrators, models and prototypes will be needed to prove construction and performance in the extraordinary operating conditions expected. The MAP study produced a consistent baseline set of requirements and technology options [81]. The work of IMCC magnet working group will evolve from these configurations. Clearly, the hope is to profit from the advances in magnet technology in the past 10 years, and in particular HTS.
6.3.3.1 Muon target, capture and cooling solenoids
The challenges identified in this part of the muon collider complex can be represented well by the first and last magnets, namely the target solenoid and the final cooling solenoid. In the scheme considered at present, the target solenoid requires a field of 20 T in a 150 mm bore. The magnet needs a large bore, driven by shielding requirement, and has substantial residual heat load, resulting from the nuclear interactions of the protons in the target. Mechanics, magnet protection, and the ability to sustain and remove a high heat influx are the aspects that dominate the design. This may require the development of conductor reinforcements, e.g. inspired by work done in large detector magnets or pulsed solenoids for fusion. HTS may be an interesting option to reduce the magnet size and increase the operating temperature. Indeed, recent developments and achievements towards the use of large HTS cables for fusion makes this option particularly interesting.
The solenoid at the end of the cooling chain directly drives the final emittance of the muon beam, which is inversely proportional to the bore field. The highest bore field is hence required in the range of 40 T (minimum) to 60 T (target) in a 50 mm bore. The best technology for such a magnet is an all-superconducting coil, which has significant advantages on footprint, investment and operating costs if compared to a superconductive/resistive hybrid option. An ultra-high field solenoid of this class has not been built yet, and the challenges span from the choice of the HTS material, possibly in the form of a cable, the extraordinary forces and stresses, quench management at large stored energy, field stability (in case of non-insulated or partially insulated winding technology is adopted), and the integration of LTS and HTS windings (in case the magnet is built as an LTS/HTS hybrid). Going well beyond available technology, this solenoid will require considerable R&D and demonstration, including studies and measurements of effects that are specific to ultra-high fields, such as the internal forces developed in tapes hosting shielding currents, or the helium phase separation in large magnetic field gradients. It must be noted that development of solenoids with such performance is of high interest also for other fields of science and societal applications, such as the high magnetic field science and the NMR spectroscopy.
6.3.3.2 Dipoles and quadrupoles for muon acceleration, storage and collision
R&D for muon acceleration magnet will be focused primarily on reducing and managing the stored energy of the accelerator ring which for the dipole circuit is of the order of several tens of MJ. At the required acceleration rate, a full magnet swing takes a fraction of millisecond, which corresponds to the peak power in the range of tens of GW. The solution envisaged is to power the magnets using power converters relying on energy storage (e.g. capacitor banks), and only replenish the energy lost during one full cycle (mainly) due to the coil resistance and hysteresis losses in iron, Alternatives such as flux-pumps with high Q-factor may be considered to improve on the energy efficiency.
The use of HTS for the fast pulsed magnets is an option considered, not so much for the potential energy saving, but rather towards higher fields and faster acceleration, with end benefits on the collider integrated luminosity. Pioneering work is being performed at FNAL, where configurations are devised to achieve exceptional performance, shown in Figure 18. The most recent results with a bore field of approximately 0.3 T at field ramp-rate of 300 T/s have shown that the ramp-rate target for the last RCS in the acceleration chain on the level of 400 T/s is within reach, albeit at reduced field [82].
While it is obvious that a strong focus is necessary on the control of AC loss at the exceedingly high ramp-rate, a particular advantage of HTS would be to increase the field swing beyond the reach of resistive magnets, which would reduce the accelerator length and increase the muon survival through the acceleration process. Further priority development topics are quench detection and protection in high voltage conditions, as well as fatigue and ageing in cyclic operation. R&D on magnets and powering systems for a muon accelerator will profit RCS for nuclear physics, as well as accelerator driven systems for radioisotopes transmutation and fission. We also expect that fast and precise management of large electric power at the GW level is an R&D where connections to several technologies can be found beyond accelerator magnets.
The challenges for dipole and quadrupole magnet technology associated with the demands stemming from the Storage Ring arc and Interaction Region are driven by the need of a high field, to keep the collider as compact as possible, and a large aperture, to accommodate shielding and large beam size in the IR magnets to achieve high luminosity. Indeed, the high level and distribution of radiation in the collider ring calls for dipole magnets with aperture much larger than a typical hadron collider. This is needed to accommodate a thick high-Z absorbers to protect the SC coils. As an alternative, open midplane configurations can be devised to provide a path for the decay electrons to high-Z external absorbers, thus sparing the coils and reducing the direct heat load. However, studies of open midplane dipoles revealed several challenges. In addition to the management of the large vertical forces, heat deposition spreads beyond the midplane due to the vertical deflection of the decay electrons. Further studies are planned to see what the most adapted magnet configuration is. For MC with c. o.m. Energies up to ∼3 TeV magnet requirements are marginally within the reach of Nb3Sn technology. For a higher energy MC, the combination of high magnetic field, large aperture and high heat flux may be resolved by devising the storage ring and IR magnets as hybrid of LTS and HTS coils. A large benefit would be obtained also by operating the HTS at higher temperature, absorbing the relatively large heat and radiation load with improved cryogenic efficiency. An important issue to be addressed is the stress management in high-field large-aperture magnet coils to avoid degradation and damage of the brittle Nb3Sn and HTS superconductor. To control the mechanical stresses in brittle Nb3Sn and HTS coils, a stress management approach has been proposed and needs to be developed and demonstrated. It is being experimentally studied by the US-MDP (see section 6.2.1).
6.3.4 High field accelerator magnet R&D in China
The Institute of High Energy Physics of Chinese Academy of Science (IHEP-CAS) is carrying out an R&D on high field accelerator magnets in partnership with research organizations working on the superconductivity and on the advanced HTS materials. This R&D program addresses the following key issues related to high-field superconducting magnet technology:
1. Development and test of new HTS materials with superior performance for application in accelerator magnets; investigation of key factors influencing material current-carrying capabilities on the microstructure and vortex dynamics level; development of advanced technologies of HTS wires with high critical current density for high field applications and high mechanical strength.
2. Development of high-current HTS cables and significantly reduce their costs; exploration of innovative structures and fabrication processes of high-field superconducting magnets based on advanced superconducting materials and helium-free cooling procedures.
3. Exploration of novel methods of stress management and quench protection for high-field superconducting accelerator magnets, particularly for high-field HTS insert coils.
4. Development of a prototype of accelerator magnet with high operation field and accelerator-quality field, building the foundation for using advanced HTS technology in high-energy particle accelerators.
The R&D of the high-field magnet technology and related advanced superconducting materials has been funded by the Chinese Academy of Sciences and the Ministry of Science and Technology on the level of 60M$ for 2018-2024.
As the first step, a 12 T subscale common-coil dipole magnet LPF1 with two apertures and graded coil was designed, fabricated, and tested. With two Nb3Sn racetrack coils inside and four Nb-Ti racetrack coils outside, the design field of this hybrid two-aperture dipole magnet is 12 T at 6100 A current at 4.2 K with the margin of 17% for the design current and field. LPF1 was tested at 4.2 K. The magnet reached quench plateau around 10.2 T after the thirteen quenches. The parameters of the design, the process of the fabrication and the test performance of LPF1 are reported in [83].
A series of 35-mm diameter IBS (Iron Based Superconductor) coils including single pancake coils (SPC) and double pancake coils (DPC) were designed and wound using the seven-filament Ba122 (Ba1-xKxFe2As2) tape. The tape was produced by the Institute of Electrical Engineering of the Chinese Academy of Sciences (IEE-CAS). The tests of the IBS coils were carried out first at 4.2 K in the 10 T background field. The highest quench current of the coils at 10 T was 68.4 A, which is about 79% of the quench current at self-field and about 90% of the short sample limit. Then two SPCs were selected and tested at 4.2 K in 24 T background field [84]. The highest quench current of the coils at 24 T was 25.6 A, which is about 39% of the coil quench current at self-field. The details of coil tests will be published in SUST. These results suggest that the iron-based superconductors are promising materials for applications in high-field accelerator magnets. These studies continue.
6.3.5 Superconducting accelerator magnet R&D in Japan
R&D on superconducting accelerator magnets has been conducted at KEK in Japan for more than 40 years. One of the major accomplishments is the development and construction of the IR quadrupoles MQXA for the LHC at CERN. KEK has also developed superconducting magnets for beam line of the T2K neutrino experiment and solenoids for muon experiments COMET and g-2/EDM. At the present time KEK is responsible for the development of the large-aperture beam separation dipole D1 for HL-LHC (see section 3.2 above). All these magnets are using Nb-Ti superconductor. The R&D works on A15 (Nb3Sn and Nb3Al) and HTS advanced superconductors are also performed. Nb3Sn conductor development for the Future Circular Collider (FCC-hh) is conducted in collaboration with CERN, Tohoku University, Tokai University, NIMS, and two Japanese industrial partners [85]. The development of HTS materials for high-field and high-radiation environment applications is performed by the US-Japan collaboration formed by KEK, Kyoto University, LBNL, and BNL [86].
As an extension of the abovementioned collaborative works, the next KEK mid-term goal is the development of radiation-hard high-field magnet technologies for future energy Frontier hadron colliders and high-intensity proton drivers. One of the R&D targets could be the 12 T 100-mm aperture beam separation dipole D1 for FCC-hh. Due to large aperture and high operation field of this magnet, mechanical stress management in the magnet structure and reduction of stress-strain sensitivity of the Nb3Sn conductor are important R&D tasks. R&D of the mechanically strengthened Nb3Sn conductor will be performed in collaboration with Tohoku University. Since the magnet will work in a relatively high radiation area, radiation hardness of the magnet structural materials, such as epoxy and insulations, have to be also studied. The ultimate goal of this R&D is to construct and test a Nb3Sn dipole model.
For HTS development, a near term goal for KEK is the development of radiation hard HTS magnet technologies for a high-intensity muon production solenoid. At J-PARC Material and Life Science Facility (MLF), the construction of the second target station is being discussed. In this target station a muon production solenoid of about 1 T central field is directly attached to the 1 MW target that produces both muons and neutrons. The facility aims to produce 50 to 100 times more muons than the current MLF muon source that results in world leading intense muon source. The muon production solenoid requires high radiation hardness and high reliability on quench protection. The current US-Japan collaboration is planned to be involved in this project. The ultimate goal of this R&D is the realization of the second target station.
In the long term, the above 2 R&D programs can be extended to the R&D of 16–20 T accelerator magnets, by combining the 12 T Nb3Sn large-aperture dipole with 4–8 T HTS or Nb3Sn insert coils. For the HTS part, the development of high current cable conductor is needed. The current US-Japan collaboration already included the study on the high-current cable development and the effort will continue. Extensive studies have been performed in the collaboration with Kyoto University of shielding currents in HTS tape conductors to achieve the accelerator field quality in HTS magnets. Since the current study on both Nb3Sn and HTS magnet technologies include the radiation hardness studies, the results of the R&D will lead to high-field, high-radiation hard accelerator magnet technology that is required for FCC insertion quadrupoles.
7 Summary and next steps
Superconducting accelerator magnets are the key enabling technology for present and future particle accelerators in modern high energy physics. All the present accelerator magnets have used for decades the Nb-Ti superconductor. The practical performance limit of this technology in accelerator magnets is limited by 8–9 T. This field level was realized in the Tevatron, HERA, RHIC and now in various LHC magnets.
This review shows that the present of superconducting accelerator magnets for high energy physics is highly dynamic, and they will remain an exciting field in the future thanks to the challenges and perspective developments driven by next generation colliders presently under study. The development of a new generation of accelerator magnets based on Nb3Sn superconductor with operation fields up to 15–16 T has shown in the last 2 decades a good progress in the U.S., Europe and Asia. On the short term, within the next 5 years, this technology will be implemented in the HL-LHC by using large-aperture high-gradient final-focus quadrupoles in ATLAS and CMS experiments. On the longer term, dipole and quadrupole magnets with nominal operation fields up to 16 T are planned for FCC-hh and 3 TeV MC. Several key technological issues important for the performance of these magnets need to be resolved. They are in the focus of national magnet R&D programs in the U.S. and Europe.
The HL-LHC promises a technology breakthrough with the introduction of Nb3Sn in the palette of superconducting materials for accelerators. Having surpassed Nb-Ti in terms of performance with characteristics that broadly match the strict beam requirements, the present focus is on the production of the first Nb3Sn magnets to be installed and operated in a running accelerator. Demonstrating performance retention throughout the whole magnet life, mastering electro- and thermo-mechanical loads, and achieving a considerable simplification of manufacturing will be not only necessary to the exploitation of Nb3Sn on large scale, but also a useful contribution for the next step in magnet technology based on HTS materials.
More ambitious R&D work towards 20 T and even higher-field magnets, which are considered for SPPC and high-energy MC and based on cost-effective HTS/LTS coils has also been started recently. Indeed, we see HTS as the upcoming technology breakpoint for future colliders. This is obviously because of the extraordinary field reach, which will be necessary to achieve manageable infrastructure scale and cost, but not only. New experiments at the scale of the colliders described here will have to produce a business case that includes environmental impact and sustainability. HTS materials promise higher fields and improved energy efficiency through operation at higher cryogenic temperature than liquid helium, or helium free configurations. Much work needs to be done to exploit the potential of HTS materials, starting from their basic conductor characteristics, through magnet design and technology, finally including considerations of operation and interaction with beam optics. The field is rich and bears connections and implications to other fields of research and societal applications, which makes it even more exciting.
Author contributions
All authors listed have made a substantial, direct, and intellectual contribution to the work and approved it for publication.
Conflict of interest
The authors declare that the research was conducted in the absence of any commercial or financial relationships that could be construed as a potential conflict of interest.
Publisher’s note
All claims expressed in this article are solely those of the authors and do not necessarily represent those of their affiliated organizations, or those of the publisher, the editors and the reviewers. Any product that may be evaluated in this article, or claim that may be made by its manufacturer, is not guaranteed or endorsed by the publisher.
References
2.ATLAS Collaboration. Observation of a new particle in the search for the standard model Higgs boson with the ATLAS detector at the LHC. Phys Lett B (2012) 716:1. doi:10.1016/j.physletb.2012.08.020
3.CMS Collaboration. Observation of a new boson at a mass of 125 GeV with the CMS experiment at the LHC. Phys Lett (2012) B716:30. doi:10.1016/j.physletb.2012.08.021
4.The European Strategy Group. Deliberation document on the 2020 update of the European strategy for particle physics. Geneva, Switzerland: CERN-ESU (2020).
5. Adolphsen M, Angalakalini D, Arndt M. In: N Mounet, editor. European strategy for particle physics - accelerator R&D roadmap. CERN (2022).
6.Sowmass (2021). Available from: https://snowmass21.org/.
7.Building for Discovery (2018). Available from: https://www.usparticlephysics.org/wp-content/uploads/2018/03/FINAL_P5_Report_053014.pdf.
8.CEEN. Future circular collider (2020). Available from: https://home.cern/science/accelerators/future-circular-collider.
9.CEPC. Circular electron positro collider (2012). Available from: http://cepc.ihep.ac.cn/intro.html.
11. Wang M-H, Noschkov J-P, Cai Y. Design of a 6 TeV muon collider. IOP Publishing for Sissa Medialab (2016). JINST 11 P09003.
12. Boscolo M, Delahaye Y, Palmer M. The future prospects of muon colliders and neutrino factories. Rev Accl Sci Tech (2019) 10(1):189–214. doi:10.1142/s179362681930010x
13. Adolphsen M, Angalakalini D, Arndt M. European strategy for particle physics – accelerator R&D roadmap (2022). arXiv:2201.07895 [physics.acc-ph].
14.B Oliver, and L Rossi, editors. The high luminosity large hadron collider. In: Advanced series on direction in high energy physics, 24. World Scientific publisher (2015).
15. Rossi L, Brüning O. Progress with the high luminosity LHC project at CERN”. Melbourne: IPAC (2019). p. 17. doi:10.18429/JACoW-IPAC2019-MOYPLM3
16. Brüning O, Gray H, Klein K, Lamont M, Narain M, Polifka R, et al. The scientific potential and technological challenges of the High-Luminosity Large Hadron Collider program. Rep Prog Phys 85:046201. doi:10.1088/1361-6633/ac5106,:
17. Kephart R, Lamm MJ, Limon P. The U.S. LHC accelerator research program: A proposal (2003). Available from: https://www.uslarp.org/LARP_Proposal.pdf.
18. Bottura L, de Rijk G, Rossi L, Todesco E. Advanced accelerator magnets for upgrading the LHC. IEEE Trans Appl Supercond (2012) 22:4002008–3. doi:10.1109/TASC.2012.2186109
19. Zlobin AV. N3Sn 11 T dipole for the high luminosity LHC (FNAL). In: D Schoerling, and AV Zlobin, editors. Nb3Sn accelerator magnets. Springer (2019). doi:10.1007/978-3-030-16118-7_8
20. Bordini B. Nb3Sn 11 T dipole for the high luminosity LHC (CERN). In: . V Zlobin, editor. Nb3Sn accelerator magnets. Springer (2019). p. 223–58. doi:10.1007/978-3-030-16118-7_9
21. Barzi E, Wokas T, Zlobin A. Sensitivity of Nb/sub 3/Sn Rutherford-type cables to transverse pressure. IEEE Trans Appl Supercond (2005) 15:1541–4. doi:10.1109/tasc.2005.849162
22. Ferradas Troitino J, Bagni T, Barth C, Bordini B, Ferracin P, Gamperle L, et al. Effects of the initial axial strain state on the response to transverse stress of high-performance RRP Nb3Sn wires. Supercond Sci Technol (2021) 34(3):035008. doi:10.1088/1361-6668/abd388
23. Hafalia RR, Bish PA, Caspi S. A new support structure for high field magnets. LBNL-47796 SC-MAG 738.
24. Ravaioli E, Ambrosio G, Bajas H, Duda M, Ferracin P, Troitino JF, et al. Quench protection studies for the high luminosity LHC Nb3Sn quadrupole magnets. IEEE Trans Appl Supercond (2021) 31:1–5. doi:10.1109/tasc.2021.3055160
25. Todesco E, Bajas H, Bajko M, Ballarino A, Bermudez SI, Bordini B, et al. The High Luminosity LHC interaction region magnets towards series production. Supercond Sci Technol (2021) 34:053001. doi:10.1088/1361-6668/abdba4
26. Ranjbar V. The RCS design status for the electron ion collider”, IPAC’21. Brazil: Campinas, SP (2021).
27. Parker B, Escallier J, Ghosh A, Jain A, Marone A, et al. BNL direct wind superconducting magnets. IEEE Trans Appl Supercond (2012) 22:4101604. doi:10.1109/tasc.2011.2175693
28. Witte H, Parker B, Palmer R. Design of a tapered final focusing magnet for eRHIC. IEEE Trans Appl Supercond (2019) 29:1–5. doi:10.1109/tasc.2019.2902982
29. Meyer D, Flasck R. A new configuration for a dipole magnet for use in high energy physics applications. Nucl Instr Methods (1970) 80:339–41. doi:10.1016/0029-554x(70)90784-6
30. Caspi S, Arbelaez D, Brouwer L, Gourlay S, Prestemon S, Auchmann B. Design of a canted-cosine-theta superconducting dipole magnet for future colliders. IEEE Trans Appl Supercond (2017) 27:1–5. doi:10.1109/tasc.2016.2638458
32. Schoerling D. The 16 T dipole development program for FCC and HE-LHC. IEEE TAS (2019) 29(5):4003109. doi:10.1109/TASC.2019.2900556
33. Lorin C, Fleiter J, Salmi T, Schoerling D. Exploration of a two-layer Nb3Sn designs of the future circular collider main qaudrupoles. IEEE Trans Appl Supercond (2019) 29(5):1. doi:10.1109/tasc.2019.2892814
34. Ballarino A, Bottura L. Targets for R&D on Nb3Sn conductor for high energy physics. IEEE Trans Appl Supercond (2015) 25(3):1–6. doi:10.1109/tasc.2015.2390149
35. Perez JC, Bajko M, Bourcey N, Bordini B, Bottura L, Troitino SF, et al. Construction and test of the enhanced racetrack model coil, first CERN R&D magnet for the FCC. IEEE Trans Appl Supercond (2022) 32:1–5. doi:10.1109/tasc.2022.3163064
36.CEPC. The CEPC conceptual design report, Vol. I. Accelerator (2018). arXiv: 1809.00285 Available from: http://cepc.ihep.ac.cn/CDR_v6_201808.pdf.
38. Kong ES, Wang C, Wang L, Wang X, Cheng D, Zhang K, et al. Conceptual design study of iron-based superconducting dipole magnets for SPPC. Int J Mod Phys A (2019) 34:1940003. doi:10.1142/s0217751x19400037
40.The U.S. Neutrino factory and muon collider collaboration (NFMCC) (2010). Available from: http://www.cap.bnl.gov/mumu/mu_home_page.html.
41.The U.S. Muon accelerator program proposal (2010). Fermilab-TM-2459-APC Available from: https://map.fnal.gov/.
42. Schulte D. The international muon collider collaboration. Proc 12th Int Part. Acc Conf Ipac- (2021) 21:3792–5. Available from: http://muoncollider.web.cern.ch.
43. Weggel RJ. Design of magnets for the target and decay region of a Muon Collider/Neutrino Factory target, IPAC2013. Shanghai, China (2013). p. 1514.
44. Stratakis D, Palmer RB. Rectilinear six-dimensional ionization cooling channel for a muon collider: A theoretical and numerical study. Phys Rev ST Accel Beams (2015) 18:031003. doi:10.1103/physrevstab.18.031003
45. Kashikhin VV, Barzi E, Lamm M, Sadovskiy Y, Zlobin A. Study of high field superconducting solenoids for muon beam cooling. IEEE Trans Appl Supercond (2008) 18:928–32. doi:10.1109/tasc.2008.921946
46. Summers DJ. Hybrid rings of fixed 8T superconducting magnets and iron magnets rapidly cycling between −2 T and +2 T for a muon collider. In: Proc. Of the 1996 DPF/DPB summer study on new directions in high-energy physics. Stanford Linear Accelerator Center (1996). p. 238.
47. Kashikhin VV, Alexanin Y, Mokahav Nv. High-field combined function magnets for a 1.5×1.5 TeV MC storage ring. New OrleansUSA: IPAC2012Louisiana (2012). 3587.
48. Kashikhin VV, Alexanin Y, Mokahav Nv. Magnets for interaction regions of a 1.5×1.5 TeV muon collider,” IPAC2012. LouisianaUSA: New Orleans (2012). 3584.
49. Gourlay S. The U.S. Magnet development program plan (2016). Available from: https://escholarship.org/uc/item/5178744.
50. Prestemon S, Amm k, Cooley L. The 2020 updated roadmaps for the us magnet development program. (2020). Available from: https://arxiv.org/abs/2011.09539.
51. Aleksan R (2004). Coordinated accelerator research in Europe. Final report Available from: https://cordis.europa.eu/project/id/506395/reporting.
52. Devred A, Baudouy B, Baynham D, Boutboul T, Canfer S, Chorowski M, et al. Status of the next European dipole (NED) activity of the collaborated accelerator research in Europe (CARE) project. IEEE Trans Appl Supercond (2005) 15(2):1106–12. doi:10.1109/TASC.2005.849506
53. de Rijk G. The EuCARD high field magnet project. IEEE Trans Appl Supercond (2012) 22:4301204. doi:10.1109/TASC.2011.2178220
54. Rossi L, Chiuchiolo A, Dhalle M, Durante M, Fazilleau P, Fleiter J, et al. The EuCARD2 future magnets program for particle accelerator high-field dipoles: Review of results and next steps. IEEE Trans Appl Supercond (2018) 28:1–10. doi:10.1109/TASC.2017.2784357
55.IFAST Project Office. Innovation fostering in accelerator science and technology (I.FAST). H-2020 Proposal 101004730 To Eu Call Infrainnov-04-2020.(2020). Available from: https://ifast-project.eu/(last accessed November 2, 2021).
56.D Schoerling, and A Zlobin, editors. Nb3Sn accelerator magnets. Particle acceleration and detection series. Springer (2019). doi:10.1007/978-3-030-16118-7
57. Rochepault E, Ferracin P. CEA–CERN block-type dipole magnet for cable testing: FRESCA2. In: Nb3Sn accelerator magnets. Particle acceleration and detection series. Springer (2019). doi:10.1007/978-3-030-16118-7_12
58. Zlobin AV, Novitski I, Barzi E, Kashikhin VV, Carmichael J, Caspi S, et al. Development and first test of the 15 T Nb3Sn dipole demonstrator MDPCT1. IEEE Trans Appl Supercond (2020) 30(4):1–5. doi:10.1109/TASC.2020.2967686
59. Devred A. Status of the 11 T dipole and CERN magnet programs beyond HiLumi. In: Presented at the 9th HL-LHC Collaboration Meeting (2019). Fermilab Available from: https://indico.cern.ch/event/806637/contributions/3487461/.
60. Tollestrup A, Larbalestier D. Very high field superconducting magnet collaboration. In: Presented at EUCARD HTS magnet program meeting. CERN (2011). Available from: https://indico.cern.ch/event/148320/contributions/1386701.
61. Prestemon S, Amm k, Cooley L. The 2020 updated roadmaps for the US magnet development program. Available from: https://arxiv.org/abs/2011.09539.
62.Eucard. EuCARD-2: Enhanced European coordination for accelerator research & development (2017). Available from: https://eucard2.web.cern.ch/eucard2/welcome.html.
63.ARIES (2017). Available from: https://aries.web.cern.ch/.
64. Bottura L, Rossi L. Technical and economical comparison YBCO/Bi-2212 magnets. Milestone MS68 report, EC-FP7-Eucard2 program Available from: https://edms.cern.ch/ui/#!master/navigator/document?D:1779412637:1779412637:subDocs.
65. Goldacker W, Nast R, Kotzyba G, Schlachter SI, Frank A, Ringsdorf B, et al. High current DyBCO-roebel assembled coated conductor (RACC). J Phys : Conf Ser (2006) 43:901–4. doi:10.1088/1742-6596/43/1/220
66. Rossi L, Senatore C. HTS accelerator magnet and conductor development in Europe. Instruments (2021) 5(8):8. doi:10.3390/instruments5010008
67. Shen T, Li P, Ye L. Heat treatment control of Bi-2212 coils: I. Unravelling the complex dependence of the critical current density of Bi-2212 wires on heat treatment. Cryogenics (2018) 89:95–101. doi:10.1016/j.cryogenics.2017.11.006
68. Wang X, Caspi S, Dietderich DR, Ghiorso WB, Gourlay SA, Higley HC, et al. A viable dipole magnet concept with REBCO CORC® wires and further development needs for high-field magnet applications. Supercond Sci Technol (2018) 31:045007–4. doi:10.1088/1361-6668/aaad8f
69. Kashikhin VV, Lombarado V, Velev G. Magnet design optimization for future hadron colliders, Melbourne, Australia: IPAC19(2019). paper THPTS084.
70. Zlobin AV, Novitski I, Barzi E. Conceptual design of a HTS dipole insert based on Bi2212 Rutherford cable. Instruments (2020) 4(29). doi:10.3390/instruments4040029
71. Zlobin AV, Novitski I, Barzi E. Reassembly and test of high-field Nb3Sn dipole demonstrator MDPCT1, 31. IEEE TAS (2021)
72. Caspi S, Borgnolutti F, Brouwer L, Cheng D, Dietderich DR, Felice H, et al. Canted–cosine–theta magnet (CCT)—a concept for high field accelerator magnets. IEEE Trans Appl Supercond (2014) 24(3):1–4. doi:10.1109/tasc.2013.2284722
73. Balachandran S, Tarantini C, Lee PJ, Kametani F, Su YF, Walker B, et al. Beneficial influence of Hf and Zr additions to Nb4at%Ta on the vortex pinning of Nb3Sn with and without an O source. Supercond Sci Technol (2019) 32.4:044006. doi:10.1088/1361-6668/aaff02
74. Xu X, Zlobin AV, Peng X, Li P. Development and study of Nb3Sn wires with high specific heat. IEEE Trans Appl Supercond (2019) 29:1–4. doi:10.1109/tasc.2019.2892325
75. Barzi E, Novitsky I, Rusy A, Turrioni D, Zlobin AV, Peng X, et al. Test of superconducting wires and Rutherford cables with high specific heat. IEEE Trans Appl Supercond (2021) 31:1–8. doi:10.1109/tasc.2021.3069047
76.The European Strategy Group. Update of the European strategy for particle physics”. CERN-ESU-013 (2020).
77.The European Strategy Group. Deliberation document on the 2020 update of the European strategy for particle physics”. CERN-ESU-014 (2020).
79. Benedikt M. Future circular collider the integrated programme (FCC-int) (2018). Available from: https://fcc-cdr.web.cern.ch/reports/EPPSU18_FCCint.pdf.
80. Adolphsen M, Angalakalini D, Arndt M. European strategy for particle physics – accelerator R&D roadmap. CERN Yellow Rep Monogr (2022) 1:1–270. doi:10.23731/CYRM-2022-001
82. Piekarz H, Claypool B, Hays S. Fast cycling HTS based superconducting accelerator magnets: Feasibility study and readiness demonstration program driven by neutrino physics and muon collider needs (2022). arXiv:2203.06253.
83. Wang CT, Cheng D, Zhang K, Wang Y, Kong E, Zhang Z, et al. Electromagnetic design, fabrication, and test of LPF1: A 10.2-T common-coil dipole magnet with graded coil configuration. IEEE Trans Appl Supercond (2019) 29(7):1. doi:10.1109/tasc.2019.2902983
84. Wang DL, Zhang Z, Zhang X, Jiang D, Dong C, Huang H, et al. First performance test of a 30 mm iron-based superconductor single pancake coil under a 24 T background field. Supercond Sci Technol (2019) 32:04LT0. doi:10.1088/1361-6668/ab09a4
Keywords: accelerator magnet, collider, magnetic field, R&D, superconductor
Citation: Bottura L, Prestemon S, Rossi L and Zlobin AV (2022) Superconducting magnets and technologies for future colliders. Front. Phys. 10:935196. doi: 10.3389/fphy.2022.935196
Received: 03 May 2022; Accepted: 29 August 2022;
Published: 12 October 2022.
Edited by:
Alessandro Tricoli, Brookhaven National Laboratory (DOE), United StatesReviewed by:
Akira Yamamoto, High Energy Accelerator Research Organization, JapanBernhard Auchmann, Paul Scherrer Institut (PSI), Switzerland
Copyright © 2022 Bottura, Prestemon, Rossi and Zlobin. This is an open-access article distributed under the terms of the Creative Commons Attribution License (CC BY). The use, distribution or reproduction in other forums is permitted, provided the original author(s) and the copyright owner(s) are credited and that the original publication in this journal is cited, in accordance with accepted academic practice. No use, distribution or reproduction is permitted which does not comply with these terms.
*Correspondence: Alexander V. Zlobin, emxvYmluQGZuYWwuZ292