- Department of Surface Engineering, Jozef Stefan Institute, Ljubljana, Slovenia
Surface functionalization of polymers by chemically reactive oxygen is a standard technique for increasing the surface energy of polymers. The technique, however, usually does not provide the desired surface finish of many polymers, including highly hydrophobic polymers like those rich in fluorine. The disappointing results are due to an incomplete understanding of the interaction between the plasma species and polymers on the atomic scale. The current state is presented, and recent advances in both theoretical and experimental descriptions of the surface mechanisms are illustrated. The scientific community faces challenges in both plasma characterization, separation of specific reactants, and detailed study of the interaction on the atomic scale. The effects of vacuum-ultraviolet radiation and its synergy with neutral reactive plasma particles are often neglected, but recent articles represent a pathway to the appropriate design of the experimental systems, which will enable systematic measurements of the evolution of surface functional groups versus the fluence of selected plasma species.
Introduction
The interaction of gaseous plasma with polymers, polymer blends, and polymer composites has attracted enormous attention from the scientific community in the past decades [1–3]. Most authors agree that the interaction of gaseous plasma with polymers causes at least two effects [4]: i–surface functionalization and ii–etching. Some authors also studied the modification of the sub-surface film and bulk polymers by gaseous plasma and found significant modifications [5].
Non-equilibrium gaseous plasma is sustained by an electrical discharge. Gas at equilibrium conditions is subjected to an electric field. Any charged particle will be accelerated in the electric field so it will gain significant kinetic energy. The charged particles will collide with neutral particles like molecules and molecular fragments. The elastic collisions between positively or negatively charged ions and neutral particles will lead to thermalization by sharing the kinetic energy of the charged particles before the collision between the charged and neutral particles after the collision. Opposite, the interaction of electrons with neutral particles will not lead to the effective transfer of the electron energy to the kinetic energy of the neutral particle kinetic energy because of the huge differences in the masses. Only a small fraction of electron energy will be transferred to the kinetic energy of the neutral particle, so the majority of the electron energy will be preserved. The electrons are therefore capable of accumulating the energy in the electric field and will be capable of causing inelastic collisions with neutral particles. The inelastic collisions will cause excitation of the rotational, vibrational, and electronic states of the neutral particles. Molecules will dissociate into fragments, and the neutral particles will be ionized at inelastic collisions with energetic electrons. The gas originally in thermal equilibrium will thus transform to a non-equilibrium state, which is characterized by a significant deviation of the excitation levels from those calculated from the presumed gas temperature. In fact, the gas temperature will not be defined anymore. Apart from the electron temperature, expressions like the rotational, vibrational, and excitation temperature of molecules have been used to describe the non-equilibrium state, providing that the distribution is reasonably close to Maxwell-Boltzmann. These temperatures are always higher than the gas temperature before the transition to the non-equilibrium. Electrons are also capable of dissociating molecules, and those from the high-energy tail of their distribution over kinetic energy will be capable of ionizing the gaseous molecules and molecular fragments.
The large temperatures (well above the kinetic temperature of the gas) and dissociation, as well as ionization fractions, are against the basic law of thermodynamics, so the non-equilibrium gas will spontaneously transform to its equilibrium unless the electric field persists. On the atomic or molecular scale, the transformation is related to collisions of gaseous particles with large kinetic or internal (rotational, vibrational, electronic, dissociation, ionization) energy with particles that are close to thermal equilibrium. The collisions are typically super-elastic: the decrease in the kinetic and/or the internal energy of energetic particles will increase the kinetic energy of particles close to thermal equilibrium. Therefore, the kinetic temperatures in the gas phase are usually higher than the ambient temperature. The thermalization may take place in the gas phase or on surfaces. As a general rule, the higher pressure will facilitate more extensive thermalization in the gas phase. In fact, the surface reactions are regarded as marginal at atmospheric pressure [6].
The accommodation, relaxation, recombination, and neutralization often occur on the surface of a plasma reactor. If plasma is sustained at the pressure below a few mbar, the surface reactions usually prevail. The heat is therefore dissipated predominantly on surfaces of materials facing the plasma. If materials are cooled, a non-equilibrium gaseous plasma of low kinetic temperature of neutral particles can be sustained rather uniformly in large volumes. In fact, many industrial-size reactors provide reasonable uniform plasma in volumes measured in cubic meters [7].
The exact values of the ionization and dissociation fractions of gaseous molecules, the distribution over electronic and vibrational states, and the energy distribution of electrons and the fluxes of all these particles as well as photons onto a sample exposed to plasma depend on the peculiarities of the plasma reactors, the types of gases and flows, the pressure, etc. A sample will be thus exposed to a flux of the following particles:
- Electrons (which will be retarded in the sheath next to the sample so that the flux will be much lower than that calculated from the temperature and density in plasma);
- Positively charged ions which will be accelerated in the sheath next to the sample, so their flux is best described as
- Neutral molecules and molecular fragments (which will not be affected by the sheath voltage, so their flux will be
- Radiation in a broad range of wavelengths between infrared (IR) and vacuum ultraviolet (VUV).
A scientifically spotless interaction of plasma particles with the materials facing plasma should involve the interaction of all these plasma particles with solid material on the atomic scale. Obviously, any description of the interaction should involve the known fluxes of the plasma particles. A vast majority of scientific reports, however, do not mention the fluxes, so the science of the interaction with many materials is still in its infancy. Furthermore, synergies of various particles are expected to play a significant role (dominant in some cases like reactive ion etching). Finally, the samples themselves may represent a source of gaseous particles, for example, because of the desorption of volatile molecules, including those synthesized as a result of plasma-sample interaction.
The variety of plasma particles and unknown fluxes makes interpreting the experimental results very difficult. An obvious simplification would be separating one single type of plasma particle (for example, either positive ions or neutral atoms in the ground state or photons) and studying the evolution of the surface properties versus the flux or fluence of the selected type of plasma particles. Such a study can be performed theoretically, but very few experimentalists adopted this praxis.
Surface Activation of Polymers
Let us focus on one of the most popular aspects of plasma-surface science: activation of polymers. Activation stands for increasing the surface energy or, more precisely, increasing the polar component of the surface energy. Polymers with low surface energy are hydrophobic, and those with high surface energy are hydrophilic. The terms “super-hydrophobic” and “super-hydrophilic” indicate extreme cases. The materials will exhibit the super-hydrophobic character if the contact angle of a water droplet is above 150°. Materials with a water contact angle below about 7° are super-hydrophilic. An example of a super-hydrophobic surface is a lotus leaf, while the super-hydrophilic surfaces don’t exist in nature. Namely, the very high surface energy is against the law of thermodynamics, so even if it happens that material is made super-hydrophilic, it will soon convert to a more stable state–moderate hydrophilicity. The return to such a state is called “hydrophobic recovery” and is observed for all polymers. The hydrophobic recovery was studied by several groups. For example, Mortazavi et al. [8] reported a model for diffusion-driven hydrophobic recovery in plasma-treated polymers. They found the driving force of hydrophobic recovery the gradients in the concentration of oxygen-containing functional groups. They considered both the diffusion and reorientation model and compared the theoretical results with experiments performed by other groups. The characteristic decay time was found of the order of 100 or 1,000 h.
Vandenbossche et al. [9] focused on the hydrophobic recovery of thin polymer films prepared by plasma polymerization (PP). They found polymer-like coatings prepared by PP more stable than organic coatings prepared by conventional chemical methods and explained the stability with the higher degree of crosslinking. They also attributed the hydrophobic recovery of plasma-treated polymers to the reorientation of polar groups and diffusion. They also considered the diffusion of low molecular weight chains toward the bulk of the polymer film as a reason for hydrophobic recovery. They also provided methods for suppressing this effect, for example, by a higher degree of crosslinking, deposition of an ultra-thin functional top-layer onto the polymer surface, or by covalent grafting of functional coating.
Pogreb et al. [10] used a three-exponential decay kinetic model to describe the mechanical and electret responses of different polymers to hydrophobic recovery. They found a correlation between the loss of surface charge and hydrophilicity. The charge induced by plasma treatment may be trapped by structural defects, impurity centers, or grain boundaries. The voltage caused by the trapped charge is of the order of mV, and the surface charge of the order of µC/m2 [11]. The hydrophobic recovery of selected polymers was accomplished within an hour at ambient conditions.
The high surface energy is obtained by grafting polar functional groups on the polymer surface. There are several options, but the widely used one is the formation of oxygen-rich functional groups. A straightforward method is the treatment of a polymer with oxygen plasma or plasma sustained in another gas with oxygen admixture. A schematic of the interaction between oxygen plasma and a polymer surface is shown in Figure 1.
Oxygen plasma is rich in the following particles:
- Free electrons
- Neutral molecules in the ground state (
- Neutral atoms in the ground state and metastable states (denoted as 1D and 1S with the radiation lifetime of 100 and 1 s, and potential energy of 1.967 and 4.189 eV respectively)
- Positively charged molecules and atoms in the ground electronic state (denoted as X2Ʃg and 4S 3/2 with the potential energy of 12.059 and 16.618 eV, respectively);
- Negatively charged molecules and atoms in the ground electronic state (denoted as 2пg and 2P );
- Radiation in the near infrared, visible and vacuum ultraviolet range of wavelengths (photon energies between about 1 and 10 eV).
The fluxes of all these particles are calculated as stated in the introduction, while the flux of photons depends on the excitation of the resonant levels. The fluence is simply a product of flux and exposure time. The free electrons, although their temperature is several 10,000 K, will have little influence on the polymer surface chemistry because they are retarded by the sheath voltage (unless a thin polymer sample is basked by an RF-powered electrode). The same applies to negative ions. The particles that should be taken into account in any attempt to study the polymer activation with oxygen plasma are thus neutral reactive species, positively charged ions, and radiation.
Radiation
The radiation arising from oxygen plasma is monitored by appropriate spectrometers. Standard inexpensive spectrometers will operate in the range of wavelengths from about 200 to 1,100 nm, and should be calibrated for the spectral response. If combined with photometers, they will measure the flux of photons. Typical spectra of oxygen plasma sustained by an inductively coupled radiofrequency discharge in a glass tube of diameter 40 mm as measured with a calibrated spectrometer are shown in Figure 2. Spectra were acquired at the same pressure of 20 Pa, but different absorbed powers of 30 W (plasma in the E-mode) and 300 W (H-mode). The integration time was 2000 ms (E-mode) and 2 ms (H-mode) and was taken into account in Figure 2. The radiation intensity is roughly 1000-times larger in the H-mode, but the spectral features are similar. The predominant lines are in the red and near-infrared parts at 777 and 845 nm and correspond to transitions of electronically excited atoms from high to lower states. The photon energy is only 1.6 and 1.5 eV, so much lower than the binding energy of atoms in the polymer. Correspondingly, the photons at 777 and 845 nm will cause little modification of the polymer surface except that they heat the surface film. No significant radiation is observed in the UV part of the spectrum in Figure 2.
The spectra shown in Figure 2 are far from representative for polymer modifications since the major radiation from O atoms in oxygen plasma arises from transitions between the resonant and the ground levels. The radiation can be simulated using the NIST codes [12]. Figure 3 represents simulated spectra radiated from neutral oxygen atoms at several electron temperatures in the range of wavelengths from 100 to 1,000 nm. The electron density was set to 1 × 1016 m−3, which is a typical value for weakly ionized non-equilibrium plasma useful for tailoring surface properties of polymers [13]. The most intensive line in plasma of electron temperature of 2 eV is at 130 nm, which corresponds to the transition 3S° − 3P. Some other lines in the VUV part of the spectrum are also much more intensive than those at 777 and 845 nm, especially at larger electron temperatures. Oxygen plasma is thus an extensive source of photons of energy 9.5 eV and above. This energy is much larger than any binding energy in the polymer, so the radiation will break bonds in the surface film of all polymers. Little work has been done on measuring the penetration depth of such photons in polymers, so such experiments represent a scientific challenge for experimentalists.
The treatment by VUV photons without the presence of other species in oxygen plasma can be performed by placing a VUV transparent window on the sample exposed to plasma. The flux should be measured with a calibrated VUV spectrometer. Little work has been published on such experiments. Among them, Iglesias et al. [14] measured the VUV radiation from oxygen plasma sustained at the pressure of 25 Pa by a microwave (MW) discharge and reported the flux of about 3 × 1019 m−2s−1. Woodworth et al. [15] also used MW discharge, but at the power of 300 W and pressure 2.7 Pa, and found the flux of VUV photons about 3 × 1019 m−2s−1. Lee et al. [16] probed inductively coupled RF oxygen plasma at the pressure of 1.3 Pa and the discharge power of 100 W and reported the VUV flux of 1.1 × 1019 m−2s−1. The values are not comparable because the authors used different discharge configurations, but the reported order of magnitude was always 1019 m−2s−1. The influence of such radiation on polymer surface properties has not been reported and remains a scientific challenge.
Positively Charged Ions
The positively charged oxygen ions are formed predominantly at inelastic collisions between plasma electrons at the high-energy tail of the distribution function. They are neutralized either in the gas phase or on the surfaces. The neutralization in the gas phase is predominant at atmospheric pressure and on surfaces at low pressures. The latter is very efficient: the neutralization probability is almost 100% for all materials. As a consequence, the density of ions is much lower than the density of other reactive particles in oxygen plasma. In a typical plasma useful for surface modification of polymers, the density of positively charged ions is of the order of 1016 m−3 [17–19]. The flux is, therefore, of the order of 1018 m−2s−1. It is not feasible to separate the ions from neutral reactive gaseous particles unless a complicated device is employed. The kinetic energy of ions impinging the surface of a material kept at floating potential is a few kTe (k is the Boltzmann constant, and Te is the electron temperature in plasma). The kinetic energy is thus about 10 eV for many oxygen plasmas. Such ions do not penetrate deep into the polymer material but cause surface modifications. The kinetic energy is large enough to break bonds between atoms in the polymer surface layer, so the interaction probability is large. No report about the details was published in the scientific literature because separating the ions from neutral reactive particles is not feasible. Still, some authors studied the interaction of positively charged ions with polyethylene exposed to weakly ionized air plasma. They found ions trapped by the CH2 groups [20]. The trapping occurred in a characteristic time of less than a second and did not depend much on the density of positively charged ions in gaseous plasma.
An alternative is the application of energetic positively charged ions, either by biasing the polymer (placing it on an electrode that is powered with an RF generator) or by using ion guns. The energetic ions will heat the surface film of the polymer, so they are not useful for practical applications.
Negatively Charged Ions
Oxygen is electro-negative gas, so slow electrons are likely to attach to neutral oxygen molecules in the ground electronic state, thus forming negatively charged ions [21]. Negative ions do not play a significant role in surface modification of polymers unless rarely used discharges are employed [22]. Namely, polymer samples are usually kept at a floating potential upon plasma treatment. The surface is negatively biased against the non-perturbed plasma in order to assure zero net currents, i.e., equal fluxes of electrons and positive ions onto the polymer surface. The electric field across the sheath will accelerate positively charged ions and reflect all negatively charged particles but those of the largest kinetic energy. The kinetic energy of electrons in unperturbed plasma (i.e., “electron temperature”) is usually a few eV, while the kinetic energy of negatively charged molecules is the same as the kinetic energy of all heavy particles (below 0.1 eV in non-equilibrium gaseous plasma useful for the treatment of polymers). A small fraction of electrons (those at the high-energy tail of the distribution function) will actually reach the surface. The negatively charged ions, On the other hand, will diffuse in the gas phase, but when they approach the surface of an object at a floating potential, they will be reflected back to the plasma, so they will not influence the polymers.
Neutral Reactive Particles
Neutral reactive species suitable for modification of surface properties of polymer materials abound in oxygen plasma. The reason is twofold: i–the excitation energy is always lower than the ionization energy of the molecules, so it is likely that the electrons will favorite excitation from ionization [23], and ii–the loss of neutral species on the surfaces may be suppressed by a careful design of the experimental plasma reactor [24]. As a consequence, the density of neutral reactive species in oxygen plasma sustained at low power density (discharge power divided with the volume of plasma) is orders of magnitude larger than the density of positively charged molecules [25]. Furthermore, neutral reactive particles can be separated from charged particles and photons, either by using pulsed discharges [26] or flowing afterglows [27]. In both cases, one benefits from the long lifetime of neutral particles compared to charged particles and radiation. The flowing afterglows are preferred as they assure a constant and high density of neutral plasma particles since the discharge operates continuously. The polymer samples are effectively screened from any radiation from gaseous plasma. A schematic of the experimental system useful for studying the interaction of neutral plasma particles with polymer materials is presented in Figure 4. The source gas is introduced into the discharge chamber, which should be made from a material of low coefficient for the surface loss of neutral reactive particles. Plasma is sustained in the discharge chamber to assure adequate production of both neutral and charged particles. The gas flow from the discharge to the afterglow chamber results from continuous pumping. There is a strong gradient in the tube between the discharge and afterglow chamber [28], enabling the gas velocity of about 100 m/s in the connecting tube. The length of the tube is often about 10 cm, so the residence time of the gas in the tube is about 1 ms. Such a short residence time and relatively large pressure (as compared to the afterglow chamber) within the connecting tube assure for minimal loss of neutral reactive particles by surface reactions. There is no electric field in the connecting tube, so the plasma electrons are not accelerated, and the charged particles neutralize on the surface of the connecting tube. On the other hand, the neutral reactive particles survive the path through the connecting tube, so their relative density is comparable to those in plasma [29]. The large speed of the gas is essential for preserving the high density of neutral particles; otherwise, strong gradients in the density of the neutral particles appear at the edge of the glowing plasma [30]. The connecting tube is bent to prevent any UV/VUV radiation at the position of the polymer sample.
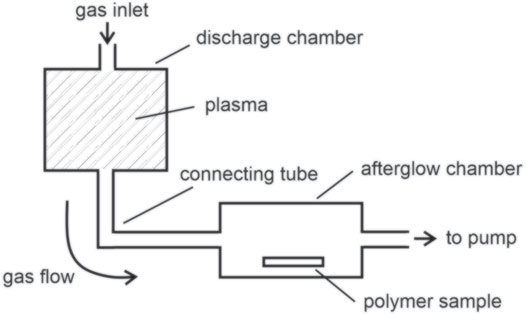
FIGURE 4. Shematic of an experimental system for treating polymer samples with neutral reactive particles only.
The setup shown in Figure 4 is, therefore, useful for studying the interaction of neutral reactive particles and polymeric materials. Both molecules and atoms in the ground state abound in the afterglow reactor, and the excited atoms of radiation lifetime below a few ms are supposed to relax on the way.
Metastable oxygen molecules of the lowest excitation energy are those in the singlet state. The kinetics of such molecules in the gas phase have been studied in detail [31], but the interaction with the polymer surfaces is yet to be studied. The major reactants in the afterglow chamber are neutral oxygen atoms in the ground state (hereafter: O-atoms).
Many authors concentrated their investigation on the interaction of O-atoms with polyolefins. One of the first theoretical and experimental articles was published as [32]. The authors predicted the subtraction of a hydrogen atom chemically bonded to a carbon atom and the formation of a dangling bond on the polymer surface. A similar reaction was proposed for plasmas sustained at atmospheric pressure [33]. The formation of dangling bonds on the surface is difficult to prove experimentally since the O-atoms will occupy them immediately. The interaction of O-atoms with polystyrene was recently studied in detail by the density functional theory study of oxygen adsorption on polymer surfaces [34]. The authors identified over ten binding sites for O-atoms, and the occupation of the binding sites was found to depend on the coverage of the polymer surface with chemically bonded oxygen. According to this theory [34], by far the most probable reaction is the substitution of the hydrogen atom on the surface with the hydroxyl groups. Once the surface is saturated with such groups, the degradation of the aromatic ring was proposed, followed by the formation of various surface functional groups.
The kinetics of surface functionalization of polystyrene upon exposure to O-atoms was also studied experimentally [35] using a system shown schematically in Figure 4. The O-atom density in the afterglow chamber was about 1017 m−3, and the authors studied the evolution of the surface functional groups as detected by X-ray photoelectron spectroscopy (XPS) versus the fluence of O-atoms. They confirmed the theoretical prediction about the rapid functionalization with the hydroxyl groups but were unable to determine the initial reaction probability. One obstacle is definitely the final escape depth of the photoelectrons when using XPS as the probing technique. Using perfectly smooth polymers and application of the angular resolved XPS should help interpret the results, but such experiments are time-consuming and are yet to be performed. The schematic of the surface functionalization of polyolefin with O-atoms is shown in Figure 5. The fluences of O-atoms required for initiation and saturation of the polystyrene surface with various functional groups are shown in Table 1.
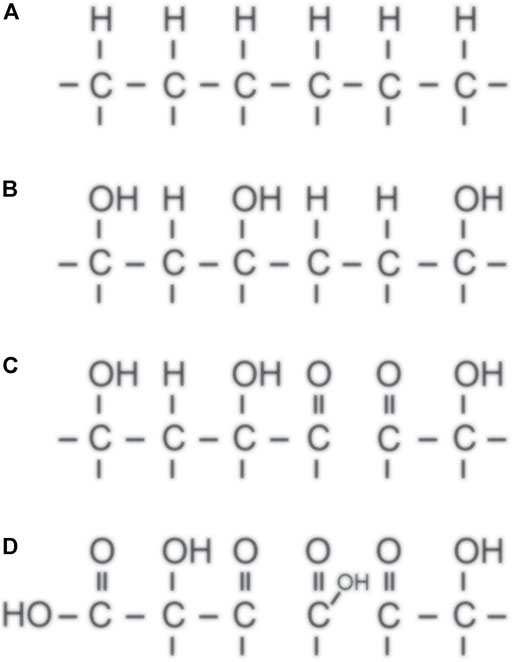
FIGURE 5. Shematic of the evolution of the surface functional groups on polyolefin as predicted by theory and confirmed experimentally. Untreated polyolefin (A) is first functionalized with OH groups (B), and treatment at larger O-atom fluences causes the formation of C=O (C) and O-C=O groups (D).
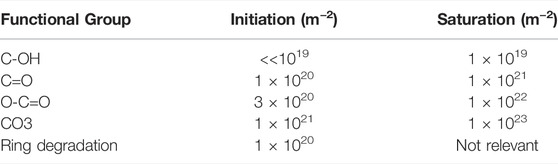
TABLE 1. Approximate fluences of neutral oxygen atoms in the ground state useful for the initiation of the functionalization of polystyrene with specific functional groups and saturation with the groups. The values were determined at room temperature.
Surface Activation of Fluorine-Containing Polymers
The considerations presented in the upper section are irrelevant for fluorinated polymers, particularly polytetrafluoroethylene (PTFE, known as Teflon). Several authors probed activation of PTFE by treating with oxygen plasma, and only a few reported improved wettability [36]. In some cases, the authors even reported an increase in the water contact angle (WCA), i.e. increased hydrophobicity instead of surface activation [37, 38]. The increased WCA by treatment with oxygen plasma has nothing in common with surface functional groups but is a consequence of roughening the PTFE surface because of the treatment with oxygen plasma. The WCA depends on two factors: i–the surface free energy and ii–the roughness on the nanometer scale. The treatment of PTFE and similar fluorinated polymers with a weakly ionized highly dissociated oxygen plasma obviously causes etching rather than substitution of surface fluorine atoms with oxygen atoms. According to [37], the major interaction products are CF2O molecules and CO and CO2. More generally, the reaction products are various carbon oxyfluorides, but little work has been performed on detailed characterization of the products desorbed from PTFE materials upon treatment with oxygen plasma. The characterization of the surfaces as well as volatile reaction products remains a scientific challenge.
According to the discussion in Radiation, the plasma species capable of breaking bonds between atoms in the surface film of polymers are energetic photons. Figure 3 reveals significant fluxes of VUV radiation from oxygen plasma, so one can conclude that the photons from oxygen plasma do cause the breaking of C-F bonds in PTFE. Fluorine atoms desorb from the polymer surface upon vacuum conditions, and the dangling bonds are occupied by oxygen atoms. The carbon oxyfluorides are then desorbed from the surface, so little oxygen remains on the PFTE surface upon treatment with oxygen plasma.
The formation of oxyfluorides is suppressed or even eliminated using plasma rich in VUV radiation but almost free from oxygen. Such plasma is sustained in hydrogen at low pressure and moderate discharge power density [39]. Other low-pressure plasmas are also powerful sources of VUV radiation [40].
The interaction between hydrogen plasma and PTFE is illustrated in Figure 6. Stoichiometric PTFE is exposed to VUV radiation, positively charged ions, and H atoms (a). The VUV radiation breaks C-F bonds, so an F atom is freed. The F atom will interact with H atoms from hydrogen plasma and form the HF molecule, which desorbs from the PTFE surface and is pumped away from the plasma reactor (b). The dangling bond is occupied by a hydrogen atom. As the process continues (c), more fluorine on the surface is substituted by H-atoms until a layer of polyolefine-like structure forms on the PTFE surface (d).
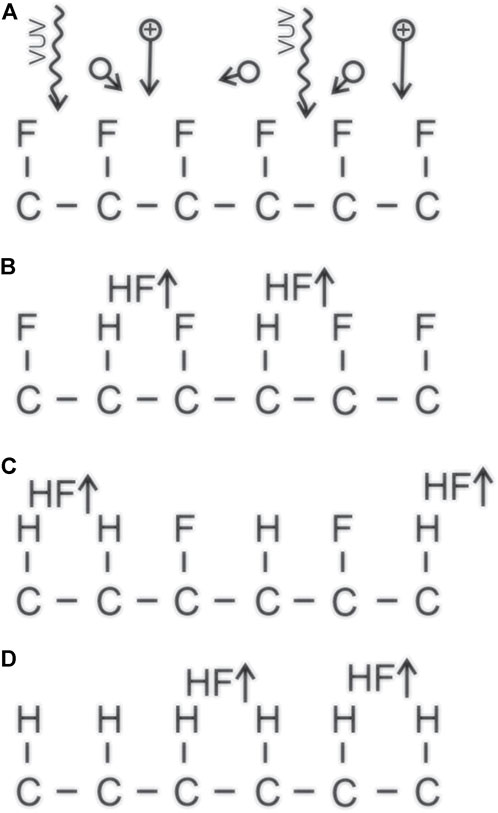
FIGURE 6. Illustration of the interaction between hydrogen plasma and surface of fluorinated polymers. (A) pristine polymer is exposed to VUV radiation, positively charged ions and neutral atoms; (B) some F atoms are substituted by H atoms, and an HF molecule is desorbed; (C) more F atoms are substituted with H atoms; (D) a polyolefin-like surface layer is formed.
Figure 6 is only an illustration. The penetration depth of VUV radiation is more than a few monolayers, so a thicker surface film is depleted of fluorine. Furthermore, the VUV photons also break C-C bonds, so it is likely that some etching occurs even in the case of hydrogen plasma treatment of PTFE. The molecules desorbed from the surface of PTFE upon treatment with hydrogen plasma may also be difluoroethylene, vinyl fluoride, and alike, but the etching products are yet to be studied. Whatever the products, the treatment of PTFE by hydrogen plasma causes the formation of a polyolefine-like surface film, which was confirmed by carefully designed experiments [41]. The thickness of the fluorine-free surface films obtained after the treatment with hydrogen plasma is a few monolayers only, as was demonstrated by angular resolved XPS measurements [41].
Once the fluorine-free surface film is obtained by treatment with hydrogen plasma, the functionalization of PTFE is rather easily achieved by brief exposure to oxygen atoms following the pathway illustrated in Figure 5. Such two-stage treatments have been elaborated for PTFE only, and the generalization of the method to other polymers which are inadequately functionalized by oxygen plasma represents a scientific challenge for future research.
Conclusion
The science of surface activation of polymer materials is still in its infancy, and tremendous progress is expected in the near future. There are only a handful of useful theories on the interaction between reactive plasma particles and deep UV radiation with polymer surfaces on the atomic scale. The experimentalists face several challenges. Apart from the trivial one, i.e. description of the surface modifications versus the fluxes and/or fluences of reactive plasma particles and radiation (and not versus the discharge parameters like the discharge configuration and power, gas pressure and flow, etc.) a challenge is separating one type of the reactive plasma species and study the evolution of the surface functional groups versus the fluence of the selected species. A few groups have already performed such experiments. The evolution of surface functional groups versus the fluence of O-atoms was already elaborated for polystyrene, while other polymers are yet to be tackled. The activation of fluorinated polymers by O-atoms is not feasible because any surface functionalization is accompanied by etching, so the wettability of such materials after treatment with oxygen plasma is inadequate. A useful method for surface functionalization of fluorinated polymers is exposure to VUV radiation in the absence of reactive oxygen species. The VUV radiation breaks C-F bonds and thus releases fluorine. The dangling C- bond can be occupied with oxygen by treatment with neutral oxygen atoms in the ground state. Both theoretical and experimental results confirm the functionalization of polyolefine films with O-atoms in the absence of other plasma species such as positively charged ions or radiation.
Data Availability Statement
The raw data supporting the conclusion of this article will be made available by the authors, without undue reservation.
Author Contributions
RZ and MM conceived the manuscript. RZ prepared the illustrations and simulations. Both authors wrote and reviewed the article.
Funding
This research was funded by the Slovenian Research Agency, project No. L2-2616 (Selective functionalization of polymer components) and core funding P2‐0082 (Thin‐film structures and plasma surface engineering).
Conflict of Interest
The authors declare that the research was conducted in the absence of any commercial or financial relationships that could be construed as a potential conflict of interest.
Publisher’s Note
All claims expressed in this article are solely those of the authors and do not necessarily represent those of their affiliated organizations, or those of the publisher, the editors and the reviewers. Any product that may be evaluated in this article, or claim that may be made by its manufacturer, is not guaranteed or endorsed by the publisher.
References
2.Non-Thermal Plasma Technology for Polymeric Materials. In: S Thomas, M Mozetič, U Cvelbar, P Špatenka, and KM Praveen, editors. Non-Thermal Plasma Technology for Polymeric Materials. Elsevier (2019).
3.Plasma Modification of Polyolefins. Plasma Modification of Polyolefins. In: PS Sari, T Vackova, and S Thomas, editors. Baneesh NS. Switzerland: Springer (2022).
4. Oehr C. Plasma Surface Modification of Polymers for Biomedical Use. Nucl Instrum Methods Phys Res Sect B Beam Interact Mater Atoms (2003) 208:40–7. doi:10.1016/s0168-583x(03)00650-5
5. Poncin-Epaillard F, Brosse JC, Falher T. Cold Plasma Treatment: Surface or Bulk Modification of Polymer Films? Macromolecules (1997) 30(15):4415–20. doi:10.1021/ma961585d
6.Plasma Applications for Material Modification. Plasma Applications for Material Modification. In: FL Tabares, editor. New Yourk. Jenny Stanford Publishing (2021).
7. Vesel A, Primc G, Zaplotnik R, Mozetič M. Applications of Highly Non-equilibrium Low-Pressure Oxygen Plasma for Treatment of Polymers and Polymer Composites on an Industrial Scale. Plasma Phys. control. Fusion (2020) 62(2):024008. doi:10.1088/1361-6587/ab5b50
8. Mortazavi M, Nosonovsky M. A Model for Diffusion-Driven Hydrophobic Recovery in Plasma Treated Polymers. Appl Surf Sci (2012) 258(18):6876–83. doi:10.1016/j.apsusc.2012.03.122
9. Vandenbossche M, Hegemann D. Recent Approaches to Reduce Aging Phenomena in Oxygen- and Nitrogen-Containing Plasma Polymer Films: An Overview. Curr Opin Solid State Mater Sci (2018) 22(1):26–38. doi:10.1016/j.cossms.2018.01.001
10. Pogreb R, Loew R, Bormashenko E, Whyman G, Multanen V, Shulzinger E, et al. Relaxation Spectra of Polymers and Phenomena of Electrical and Hydrophobic Recovery: Interplay between Bulk and Surface Properties of Polymers. J. Polym. Sci. Part B Polym. Phys. (2017) 55(2):198–205. doi:10.1002/polb.24260
11. Shapira Y, Chaniel G, Bormashenko E. Surface Charging by the Cold Plasma Discharge of Lentil and Pepper Seeds in Comparison with Polymers. Colloids Surfaces B Biointerfaces (2018) 172:541–4. doi:10.1016/j.colsurfb.2018.09.004
12. Kramida A, Ralchenko Y, Reader J, Team NISTASD. Nist Atomic Spectra Database. Gaithersburg, MD, USA: National Institute of Standards and Technology (2018). [updated 2022, March 8]. Available from: https://physics.nist.gov/asd.
13. Vesel A, Elersic K. Adsorption of Protein Streptavidin to the Plasma Treated Surface of Polystyrene. Appl Surf Sci (2012) 258(15):5558–60. doi:10.1016/j.apsusc.2011.12.131
14. Iglesias EJ, Mitschker F, Fiebrandt M, Bibinov N, Awakowicz P. In Situmeasurement of VUV/UV Radiation from Low-Pressure Microwave-Produced Plasma in Ar/O2gas Mixtures. Meas. Sci. Technol. (2017) 28(8):085501. doi:10.1088/1361-6501/aa7816
15. Woodworth JR, Riley ME, Amatucci VA, Hamilton TW, Aragon BP. Absolute Intensities of the Vacuum Ultraviolet Spectra in Oxide Etch Plasma Processing Discharges. J Vac Sci Technol A Vac Surfaces, Films (2001) 19(1):45–55. doi:10.1116/1.1335685
16. Lee J, Graves DB. The Effect of VUV Radiation from Ar/O2plasmas on Low-kSiOCH Films. J. Phys. D Appl. Phys. (2011) 44(32):325203. doi:10.1088/0022-3727/44/32/325203
17. Woo Seo M, Keun Bae M, Chung TH. Comparison of Various Interpretation Methods of the Electric Probe Measurements in Inductively Coupled Ar and O2 Plasmas. Phys Plasmas (2014) 21(2):023514. doi:10.1063/1.4867350
18. Tuszewski M. Particle and Heat Transport in a Low-Frequency Inductively Coupled Plasma. Phys Plasmas (1998) 5(4):1198–205. doi:10.1063/1.872649
19. Vassallo E, Pedroni M, Silvetti T, Morandi S, Brasca M. Inactivation of Staphylococcus Aureus by the Synergistic Action of Charged and Reactive Plasma Particles. Plasma Sci. Technol. (2020) 22(8):085504. doi:10.1088/2058-6272/ab8c32
20. Bormashenko E, Whyman G, Multanen V, Shulzinger E, Chaniel G. Physical Mechanisms of Interaction of Cold Plasma with Polymer Surfaces. J Colloid Interface Sci (2015) 448:175–9. doi:10.1016/j.jcis.2015.02.025
21. Gibson AR, Gans T. Controlling Plasma Properties under Differing Degrees of Electronegativity Using Odd Harmonic Dual Frequency Excitation. Plasma Sources Sci. Technol. (2017) 26(11):115007. doi:10.1088/1361-6595/aa8dcd
22. Sharma S, Linnane C, Gahan D, Daniels S, Hopkins MB. Formation of a Double Layer in Electronegative ${\rm O}_{2}$ Plasma. IEEE Trans. Plasma Sci. (2014) 42(10):2798–9. doi:10.1109/tps.2014.2313179
23. Alves LL, Coche P, Ridenti MA, Guerra V. Electron Scattering Cross Sections for the Modelling of Oxygen-Containing Plasmas*. Eur. Phys. J. D (2016) 70(6). doi:10.1140/epjd/e2016-70102-1
24. Guerra V. Analytical Model of Heterogeneous Atomic Recombination on Silicalike Surfaces. IEEE Trans. Plasma Sci. (2007) 35(5):1397–412. doi:10.1109/tps.2007.902028
25. Gibson AR, Foucher M, Marinov D, Chabert P, Gans T, Kushner MJ, et al. The Role of Thermal Energy Accommodation and Atomic Recombination Probabilities in Low Pressure Oxygen Plasmas. Plasma Phys. control. Fusion (2017) 59(2):024004. doi:10.1088/1361-6587/59/2/024004
26. Dedrick J, Schröter S, Niemi K, Wijaikhum A, Wagenaars E, de Oliveira N, et al. Controlled Production of Atomic Oxygen and Nitrogen in a Pulsed Radio-Frequency Atmospheric-Pressure Plasma. J. Phys. D Appl. Phys. (2017) 50(45):455204. doi:10.1088/1361-6463/aa8da2
27. Ricard A, Gaillard M, Monna V, Vesel A, Mozetic M. Excited Species in H2, N2, O2 Microwave Flowing Discharges and Post-discharges. Surf Coatings Technol (2001) 142-144:333–6. doi:10.1016/s0257-8972(01)01276-2
28. Primc G, Zaplotnik R, Vesel A, Mozetic M. Microwave Discharge as a Remote Source of Neutral Oxygen Atoms. AIP Adv (2011) 1(2):022129. doi:10.1063/1.3598415
29. Kutasi K, Zaplotnik R, Primc G, Mozetic M. Controlling the Oxygen Species Density Distributions in the Flowing Afterglow of O2/Ar-O2surface-Wave Microwave Discharges. J. Phys. D Appl. Phys. (2014) 47(2):025203. doi:10.1088/0022-3727/47/2/025203
30. Primc G, Balat-Pichelin M, Mozetič M, Vesel A. Oxygen Atom Density within the Interface between Glowing Oxygen Plasma and Equilibrium Gas. Vacuum (2017) 143:158–64. doi:10.1016/j.vacuum.2017.06.011
31. Annušová A, Marinov D, Booth J-P, Sirse N, da Silva ML, Lopez B, et al. Kinetics of Highly Vibrationally Excited O2(X) Molecules in Inductively-Coupled Oxygen Plasmas. Plasma Sources Sci. Technol. (2018) 27(4):045006. doi:10.1088/1361-6595/aab47d
32. Belmonte T, Pintassilgo CD, Czerwiec T, Henrion G, Hody V, Thiebaut JM, et al. Oxygen Plasma Surface Interaction in Treatments of Polyolefines. Surf Coatings Technol (2005) 200(1-4):26–30. doi:10.1016/j.surfcoat.2005.02.108
33. Bhoj AN, Kushner MJ. Continuous Processing of Polymers in Repetitively Pulsed Atmospheric Pressure Discharges with Moving Surfaces and Gas Flow. J. Phys. D Appl. Phys. (2007) 40(22):6953–68. doi:10.1088/0022-3727/40/22/016
34. Longo RC, Ranjan A, Ventzek PLG. Density Functional Theory Study of Oxygen Adsorption on Polymer Surfaces for Atomic-Layer Etching: Implications for Semiconductor Device Fabrication. ACS Appl. Nano Mat. (2020) 3(6):5189–202. doi:10.1021/acsanm.0c00618
35. Vesel A, Zaplotnik R, Mozetič M, Primc G. Surface Modification of Ps Polymer by Oxygen-Atom Treatment from Remote Plasma: Initial Kinetics of Functional Groups Formation. Appl Surf Sci (2021) 561:150058. doi:10.1016/j.apsusc.2021.150058
36. Primc G. Recent Advances in Surface Activation of Polytetrafluoroethylene (Ptfe) by Gaseous Plasma Treatments. Polymers (2020) 12(10):2295. doi:10.3390/polym12102295
37. Carbone EAD, Verhoeven MWGM, Keuning W, van der Mullen JJAM. Ptfe Treatment by Remote Atmospheric Ar/O2plasmas: A Simple Reaction Scheme Model Proposal. J. Phys Conf. Ser. (2016) 715:012011. doi:10.1088/1742-6596/715/1/012011
38. Ryu J, Kim K, Park J, Hwang BG, Ko Y, Kim H, et al. Nearly Perfect Durable Superhydrophobic Surfaces Fabricated by a Simple One-step Plasma Treatment. Sci Rep (2017) 7(1):1981. doi:10.1038/s41598-017-02108-1
39. Fantz U, Briefi S, Rauner D, Wünderlich D. Quantification of the Vuv Radiation in Low Pressure Hydrogen and Nitrogen Plasmas. Plasma Sources Sci. Technol. (2016) 25(4):045006. doi:10.1088/0963-0252/25/4/045006
40. Popović D, Mozetič M, Vesel A, Primc G, Zaplotnik R. Review on Vacuum Ultraviolet Generation in Low‐pressure Plasmas. Plasma Process Polym (2021) 18(9):2100061. doi:10.1002/ppap.202100061
Keywords: plasma, polymer, VUV, radicals, Teflon, hydrophilic, substitution
Citation: Zaplotnik R and Mozetič M (2022) Frontiers in the Interaction of Chemically Reactive Species From Gaseous Plasma With Hydrophobic Polymers. Front. Phys. 10:896219. doi: 10.3389/fphy.2022.896219
Received: 14 March 2022; Accepted: 19 April 2022;
Published: 03 May 2022.
Edited by:
Masaharu Shiratani, Kyushu University, JapanReviewed by:
Bormashenko Edward, Ariel University, IsraelJun-Seok Oh, Osaka Metropolitan University, Japan
Copyright © 2022 Zaplotnik and Mozetič. This is an open-access article distributed under the terms of the Creative Commons Attribution License (CC BY). The use, distribution or reproduction in other forums is permitted, provided the original author(s) and the copyright owner(s) are credited and that the original publication in this journal is cited, in accordance with accepted academic practice. No use, distribution or reproduction is permitted which does not comply with these terms.
*Correspondence: Miran Mozetič, bWlyYW4ubW96ZXRpY0BndWVzdC5hcm5lcy5zaQ==