- 1Aerospace Information Research Institute, Chinese Academy of Sciences, Beijing, China
- 2Center for Advanced Laser Technology, Hebei University of Technology, Tianjin, China
We demonstrate a high-power Nd: YVO4 picosecond laser amplifier that is capable of generating 51.5 W of average output power at a wavelength of 1,064 nm, with a repetition rate of 70 MHz and a pulse duration of 8.5 ps. This system encompasses three stages of laser diode end-pumped Nd: YVO4 amplification including two double-pass amplifiers and a single-pass amplifier. Laser output with near-diffraction-limited beam quality (M2 < 1.1) was maintained throughout the entire power scaling range of the laser. The system exhibited very high output power stability with a root-mean-square amplitude fluctuation of less than 0.2% over a period of 15 h of continuous operation.
Introduction
Lasers characterized by different duration of emission have shown different advantages in respective fields [1–5]. Short pulse lasers with high output power and high repetition rates have found significant application in fields such as nonlinear optical frequency conversion [6–8], precision materials processing [9–11], satellite ranging [12, 13], gas detection [14], and optoelectronic countermeasures [15]. The ultrashort pulse duration [typically in the range of picoseconds (ps)] of these lasers is shorter than the electron-lattice relaxation time of many materials [16, 17], allowing a significant reduction in heat transfer between the laser pulse and the material, during the interaction between the laser photons and the material molecules. As such, laser pulses in the ps-range are often used in applications such as precision laser machining, biomedicine, and laser cleaning (particularly of precious and historical artifacts). While femtosecond lasers offer even shorter pulse durations, it is typically easier to design and construct lasers which output ps-pulses with high pulse energy and high power output. As such, picosecond lasers are a powerful tool in the study of the interaction between light and matter [18].
One of the most commonly used methods to generate ps-pulse duration laser outputs is mode-locking. Mode-locking is capable of producing laser pulses down to ∼10 ps, however, this is typically accompanied by extremely low pulse energy (∼nJ) and low average power (∼mW). This therefore limits the range of applications of these laser sources. To address this shortcoming, laser amplifiers are often applied to increase the power scaling capabilities of these systems. These amplifiers are mainly divided into two categories: regenerative amplifiers and traveling-wave amplifiers. Regenerative amplifiers have the advantages of high gain, high stability, and low seed laser power requirements, and the amplification of a pulsed laser can be regulated by the pressurization time of the electro-optical crystal. However, due to its resonant cavity design, the amplification of spontaneous radiation can occur which reduces the pulse contrast. Furthermore, the electro-optical crystal needs to be driven using a high-frequency and high voltage signal which matches the seed laser frequency. Also, for high repetition rates (in the MHz-range), a frequency selection module also needs to be added [19] and this greatly increases the complexity of the overall system. Other deleterious effects which may occur during amplification include multiplicative period bifurcation effects and gain narrowing [20, 21]. In comparison, traveling wave amplifiers are more compact, comprising fewer optical components which also introduce fewer optical losses. Although the regenerative amplifier can achieve an amplification factor of over 106 times, high-power picosecond lasers based on the traveling-wave amplification process offers the advantages of higher stability and robustness, and is therefore more suitable for industrial applications. At present, Nd:YAG, Nd:YLF and Nd:YVO4 are commonly used gain media for 1 μm picos amplifiers. Among them, Nd:YVO4 has a large emission cross-section, which facilitates the gain of the system and thus achieves high optical conversion efficiency during the amplification.
In recent years, there have been numerous reports on picosecond amplifiers using traveling-wave configurations. In 2018, Cheng et. al. [22] demonstrated ps-pulsed output with an average power of 11.34 W using single-pass amplification of a self-developed picosecond seed source with a repetition rate of 3.2 GHz and an average power of 1.38 W. In 2019, Liu et. al. [23] demonstrated laser output with a maximum power of 16.19 W and an optical-optical conversion efficiency of 51.07% by double-pass amplifying a single pulse from a ps-pulsed seed laser. The seed laser had a repetition rate of 500 kHz and pulse energy of 7.68 μJ. Compared with regenerative amplification techniques, which are limited by the need for high-frequency voltage drivers, traveling-wave amplification techniques can directly amplify lasers with repetition rates from the kHz to GHz range. In 2020, Dong et. al. [24] demonstrated a two-stage amplification system to amplify the output from a seed laser with a pulse width of 10.2 ps and a repetition rate of 18.9 MHz. One of the most appealing advantages of the traveling wave amplification technique is that it can be used in a multi-pass and/or cascaded manner in order to make up for its shortcomings of low single-pass and double-pass amplification.
In this brief report, we demonstrate a stable picosecond laser amplifier that utilizes three fiber-coupled LD directly pumped Nd:YVO4 amplifier stages. Key to the efficient operation of this multi-stage amplification setup is the precise matching of the spatial modes of the pump laser and seed laser spatial profiles, and this is achieved by using a lens set for pump and seed light shaping, resulting in high pumping efficiency and high beam quality output. We observe that thermally-induced effects have little impact on the output laser beam quality, and thus high beam quality is maintained with efficient amplification. We use this design to amplify a seed laser which has an average power of 320 mW, a pulse duration of 8.2 ps and a repetition rate of 70 MHz to an output with an average power of 51.5 W, and a pulse duration of 8.5 ps.
Experiment and Results
The setup of the experimental laser system is shown in Figure 1. It consists of a laser oscillator, two double-pass amplifiers and a single-pass amplifier. The seed laser (FPS200-MO-70MHZ; EKSPLA, Lithuania) had an output wavelength of 1,064 nm, a repetition rate of 70 MHz, a pulse width of 8.2 ps and an average power of 320 mW. The seed was collimated and focused using lens sets F1 [radius of curvature (ROC) of 15.5 mm] and F2 (ROC = 93 mm), then passes a half-wave plate (HWP) to adjust its polarization angle. It then entered the first double-pass pre-amplification stage through an optical isolator consisting of a polarizing beam splitter and a Faraday rotator (HPDFSI-06-100-5-H-H-P; EMGO-TECH, ZhuHai, China), which serves to protect the optical components from being damaged by the amplified laser pulse. The first stage amplifier used a 0.7 at. % doped Nd:YVO4 crystal as the gain medium (with dimensions 4 mm × 4 mm × 30 mm). The crystal is wrapped by indium foil and then dissipated through a copper heat sink with cooling water temperature of 25°C. The crystal was end-pumped with a fiber-coupled LD pump source (NLIGHT; United States) with a central wavelength of 808 nm and a spectral width of 1.5 nm. The pump laser was collimated and focused by passing through a plano-convex lens set to achieve mode matching of the pump laser to that of the laser beam under amplification, thus maximizing the amplification efficiency. M3 and M4 are two 1,064 nm high-reflection (HR) convex mirrors with R = 1,500 mm. M3 also has anti-reflective (AR) coating at 808 nm wavelength. The seed laser was reflected from the polarizing beam splitter of the optical isolator after passing through the gain medium twice, at which point the laser was S-polarized. The S-polarized laser was then re-shaped and passed through the HWP, changing the polarization state of the laser to P-polarized. The P-polarized laser beam then passed through the optical isolator of the second stage amplifier and entered the second double-pass amplification stage. The structure of the second amplifier stage was the same as that of the first stage. The pre-amplified laser was amplified twice again in the second stage before being reflected by the polarizing beam splitter of the second optical isolator into the final (third-stage) single-pass amplifier. This single-pass amplification stage also used a 4 mm × 4 mm × 30 mm Nd:YVO4 crystal as the gain medium and was also end-pumped with a fiber-coupled LD pump source with a central wavelength of 808 nm. After the third single-pass amplification stage, the laser was collimated by a convex lens and then output.
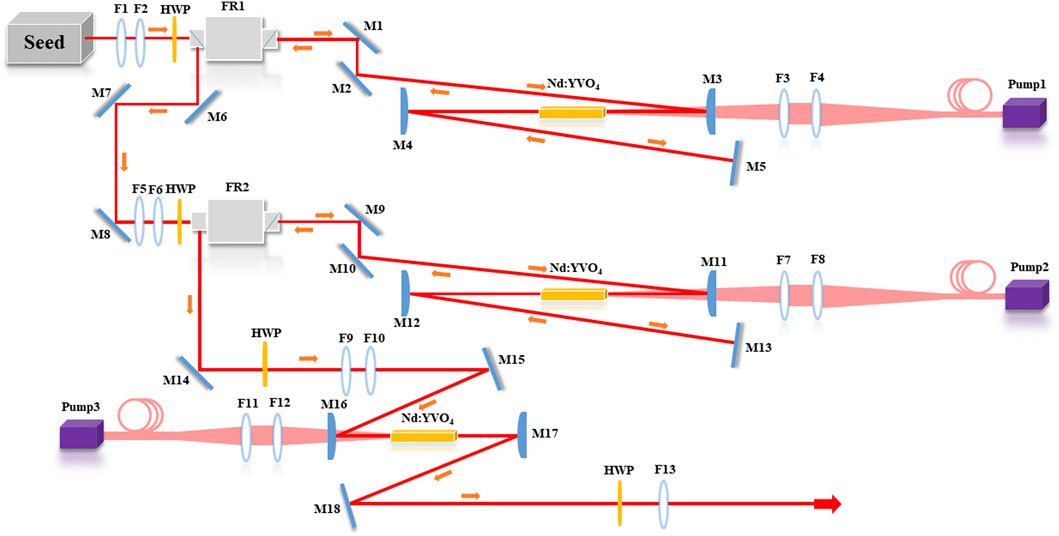
FIGURE 1. Schematic showing the layout of the high-power, high-repetition rate picosecond laser amplifier system. HWP, half-wave plate; FR, Faraday rotator; M, mirrors; F, focus lens.
In our experiments, the injection pump and amplified beam in each stage were shaped through the lens to achieve optimum mode matching. The spot diameter of the output seed is 1.2 mm. After shaping by the lens set at each stage, the spot diameter at the center of the crystal is set to be 1.3 mm, then is adjusted to be 1.4 mm at one-third of the crystal. In each amplification stage of the system, the Nd:YVO4 crystal was pumped with an incident diode pump power of 110 W. We also observed that the gain crystal absorbs ∼83% of the pump laser field. In the first amplifier stage, the power of the seed laser was amplified to ∼2.1 W in a single pass and then to ∼10 W in a double pass through the gain medium. We attribute the relatively low photo-optical conversion efficiency to the low power of the seed laser. Following amplification in the second stage double-pass amplifier, the optical power increased to ∼35 W. After amplification in the third stage, single-pass amplifier, the average output power rose to 51.5 W. The photo-optical conversion efficiency was ∼30.9%.
Shown in Figures 2A,B, the pulse characteristics of the amplified output. A photodiode (PulseCheck SM1600; Ape, Germany) was used to measure the pulse train. It can be seen that the frequency of the laser output after final amplification was 70.2 MHz, and the pulse width (as measured using a autocorrelator and normalized) was slightly broadened to 8.5 ps. We attribute the slight broadening of the output to the effects of dispersion and gain narrowing which occurs during the amplification process. The wavelength spectrum of the amplified output (as measured using a spectrometer and normalized) is shown in Figure 2C; the central wavelength was 1,064.4 nm, and the measured linewidth was 0.22 nm. Figure 2D shows the focusing characteristics of the output beam and the inset shows the near-field spatial profile of the output. The output beam had a radius of 1.2 mm and a beam pointing stability of 2.8 μrad. The beam quality factor (M2) of the output was measured to be 1.08 and 1.09 along the x and y axis directions, respectively. The experimental results indicate that thermally-induced effects do not appear to manifest in these amplifiers, both in the case of single-pass and double-pass amplification. Figure 2E shows a plot of the power stability of the laser output as measured over a period of 15 h (with the system at room temperature). This plot shows that the system had excellent output stability with a root-mean-square (RMS) power fluctuation of less than 0.2%. The polarization ratio of the output beam was measured to be 220:1. These results serve to demonstrate that through the application of a three-stage amplification setup, high average power, high repetition rate picosecond-pulse laser output with very high stability can be achieved.
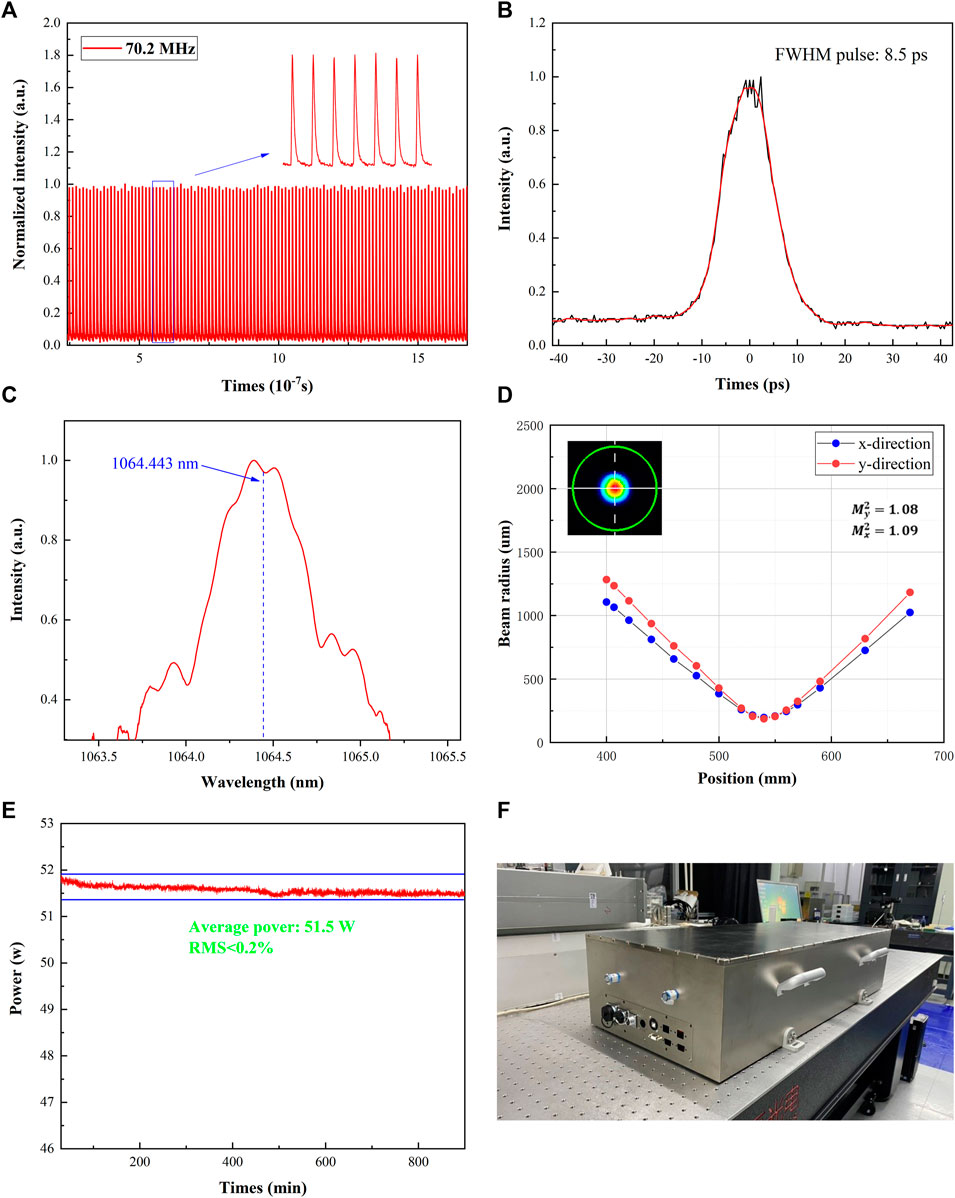
FIGURE 2. Compilation of output characteristics of the amplified ps-pulse duration laser beam. (A) Normalized pulse characteristics of the output with a close-up shown inset; (B) temporal characteristic of a single output pulse with an autocorrelation trace; (C) normalized wavelength spectrum of the amplified laser output; (D) plot of the focusing characteristics of the output laser beam along with the near-field spatial profile (inset); (E) plot tracking the stability of the output power over a period of 15 h; and (F) photograph of the packaged picosecond laser amplifier.
Conclusion
In this study, a cascaded, picosecond laser amplifier utilizing both double-pass and single-pass configurations was constructed and characterized. This amplifier design was used to amplify a beam produced by a mode-locked laser which had an average power of 320 mW, a pulse duration of 8.2 ps and a repetition rate of 70 MHz. The resultant amplified output had an average power of 51.5 W, a pulse duration of 8.5 ps, a spectral width of 0.22 nm, and a repetition rate of 70.2 MHz. Notably the amplifier design ensured that the beam quality of the output was maintained (with a measured M2 value of 1.08 being recorded) and the output power was very stable, exhibiting the RMS fluctuation of less than 0.2%.
We believe that the results demonstrated in this work are of great significance for the future realization of practical and industrially-applied, high power, high repetition rate, high beam quality and stable picosecond pulsed laser sources. Furthermore, the moderate single pulse energy (∼μJ) of this system also makes it an ideal source for applications in nonlinear optics, such as second-order nonlinear frequency conversion [25, 26], and high-power crystalline-based wavelength convertors using the stimulated Raman/Brillouin scattering process [27–31].
Data Availability Statement
The raw data supporting the conclusions of this article will be made available by the authors, without undue reservation.
Author Contributions
All authors listed have made a substantial, direct, and intellectual contribution to the work and approved it for publication.
Funding
This work was supported by the Guangdong Key Research & Development Program (2018B090904003).
Conflict of Interest
The authors declare that the research was conducted in the absence of any commercial or financial relationships that could be construed as a potential conflict of interest.
Publisher’s Note
All claims expressed in this article are solely those of the authors and do not necessarily represent those of their affiliated organizations, or those of the publisher, the editors and the reviewers. Any product that may be evaluated in this article, or claim that may be made by its manufacturer, is not guaranteed or endorsed by the publisher.
References
1. Zervas MN, Codemard CA. High Power Fiber Lasers: a Review. IEEE J Select Top Quan Electron. (2014) 20(5):219–41. doi:10.1109/jstqe.2014.2321279
2. Li S, Wang Q, Song R, Hou F, Gao M, Gao C. Laser Diode Pumped High-Energy Single-Frequency Er:YAG Laser with Hundreds of Nanoseconds Pulse Duration. Chin Opt Lett (2020) 18(3):031401. doi:10.3788/col202018.031401
3. Song J, Wang Z, Wang X, Lü R, Teng H, Zhu J, et al. Generation of 601 fs Pulse from an 8 kHz Nd:YVO4 Picosecond Laser by Multi-Pass-Cell Spectral Broadening. Chin Opt Lett (2021) 19(9):093201. doi:10.3788/col202119.093201
4. Ma Y, Hu Y, Qiao S, Lang Z, Liu X, He Y, et al. Quartz Tuning forks Resonance Frequency Matching for Laser Spectroscopy Sensing. Photoacoustics (2022) 25:100329. doi:10.1016/j.pacs.2022.100329
5. Lang Z, Qiao S, Ma Y. Acoustic Microresonator Based In-Plane Quartz-Enhanced Photoacoustic Spectroscopy Sensor with a Line Interaction Mode. Opt Lett (2022) 47(6):1295–8. doi:10.1364/ol.452085
6. Li K, Zhang L, Xu D, Zhang G, Yu H, Wang Y, et al. High-power Picosecond 355 nm Laser Based on La2CaB10O19 Crystal. Opt Lett (2014) 39(11):3305–7. doi:10.1364/ol.39.003305
7. Bai Z, Yuan H, Liu Z, Xu P, Gao Q, Williams RJ, et al. Stimulated Brillouin Scattering Materials, Experimental Design and Applications: A Review. Opt Mater (2018) 75:626–45. doi:10.1016/j.optmat.2017.10.035
8. Chen L, Bai Z, Pan Y, Chen M, Li G. Two-millijoule, 1-kHz, 355-nm Picosecond Laser Pulse Generation in LiB3O5 crystal. Opt Eng (2013) 52(8):086107. doi:10.1117/1.oe.52.8.086107
9. Shen D, Qian J, Wang C, Wang G, Wang X, Zhao Q. Facile Preparation of Silver Nanoparticles in Bulk Silicate Glass by High-Repetition-Rate Picosecond Laser Pulses. Chin Opt Lett (2021) 19(1):011901. doi:10.3788/col202119.011901
10. Du K, Brüning S, Gillner A. High-power Picosecond Laser with 400W Average Power for Large Scale Applications. In: Laser-Based Micro-and Nanopackaging and Assembly VI, Proceedings SPIE 8244, San Francisco, California, United States, 21-26 January 2012. (2012). p. 82440. doi:10.1117/12.915676
11. Weber R, Graf T, Freitag C, Feuer A, Kononenko T, Konov VI. Processing Constraints Resulting from Heat Accumulation during Pulsed and Repetitive Laser Materials Processing. Opt Express (2017) 25:3966–79. doi:10.1364/oe.25.003966
12. Zhang H, Long M, Deng H, Cheng S, Wu Z, Zhang Z, et al. Developments of Space Debris Laser Ranging Technology Including the Applications of Picosecond Lasers. Appl Sci (2021) 11(21):10080. doi:10.3390/app112110080
13. Bai Z, Chen H, Gao X, Li S, Qi Y, Bai Z. Highly Compact Nanosecond Laser for Space Debris Tracking. Opt Mater (2019) 98:109470. doi:10.1016/j.optmat.2019.109470
14. Leffler T, Brackmann C, Ehn A, Kaldvee B, Aldén M, Berg M, et al. Range-resolved Detection of Potassium Chloride Using Picosecond Differential Absorption Light Detection and Ranging. Appl Opt (2015) 54:1058–64. doi:10.1364/ao.54.001058
15. Kalisky O, Kalisky O. The Status of High-Power Lasers and Their Applications in the Battlefield. Opt Eng (2010) 49(9):091003. doi:10.1117/1.3484954
16. Bai Z, Bai Z, Sun X, Liang Y, Wang K, Jin D, et al. A 33.2 W High Beam Quality Chirped-Pulse Amplification-Based Femtosecond Laser for Industrial Processing. Materials (2020) 13(12):2841. doi:10.3390/ma13122841
17. Je G, Malka D, Kim H, Hong S, Shin B. A Study on Micro Hydroforming Using Shock Wave of 355 nm UV-Pulsed Laser. Appl Surf Sci (2017) 417:244–9. doi:10.1016/j.apsusc.2017.02.146
18. Sugioka K, Cheng Y. Ultrafast Lasers-Reliable Tools for Advanced Materials Processing. Light Sci Appl (2014) 3(4):e149. doi:10.1038/lsa.2014.30
19. Bai Z, Bai Z, Kang Z, Lian F, Lin W, Fan Z. Non-Pulse-Leakage 100-kHz Level, High Beam Quality Industrial Grade Nd:YVO4 Picosecond Amplifier. Appl Sci (2017) 7(6):615. doi:10.3390/app7060615
20. Wu Y, Yani G, Yilin H, Qingnan X, Quan Z, Weixin M, et al. Progress in High Energy All-Solid-State Regenerative Amplifier. High Power Laser Part Beams (2020) 32(11):76–86. doi:10.11884/HPLPB202032.200089
21. Bai C, Tian W, Wang G, Zhen L, Xu R, Zhang D, et al. Progress on Yb-Doped All-Solid-State Femtosecond Laser Amplifier with High Repetition Rate. Chin J Lasers (2021) 05:82–97. doi:10.3788/cjl202148.0501005
22. Cheng M, Wang Z, Cao Y, Meng X, Zhu J, Wang J, et al. High Power Diode-Pumped Passively Mode-Locked Nd:YVO4 Laser at Repetition Rate of 3.2 GHz. Chin Phys. B (2019) 28(5):054205. doi:10.1088/1674-1056/28/5/054205
23. Liu X, He H, Song Y, Wang C, Wang Z. 500-kHz Level High Energy Double-Pass Nd:YVO4 Picosecond Amplifier with Optic–Optic Efficiency of 51%. Appl Sci (2019) 9(2):219. doi:10.3390/app9020219
24. Dong X, Li P, Li S, Wang D. High Gain Fiber-Solid Hybrid Double-Passing End-Pumped Nd: YVO4 Picosecond Amplifier with High Beam Quality. Chin Phys B (2020) 29:054207. doi:10.1088/1674-1056/ab8218
25. Chen H, Bai Z, Wang J, Zhang B, Bai Z Hundred-watt green Picosecond Laser Based on LBO Frequency-Doubled Photonic crystal Fiber Amplifier. Infrared Laser Eng (2021) 50(11):20200522. doi:10.3788/IRLA20200522
26. Chu Y, Zhang X, Chen B, Wang J, Yang J, Jiang R, et al. Picosecond High-Power 213-nm Deep-Ultraviolet Laser Generation Using β-BaB2O4 crystal. Opt Laser Techn (2021) 134:106657. doi:10.1016/j.optlastec.2020.106657
27. Duan Y, Sun Y, Zhu H, Mao T, Zhang L, Chen X. YVO4 Cascaded Raman Laser for Five-Visible-Wavelength Switchable Emission. Opt Lett (2020) 45(9):2564–7. doi:10.1364/ol.392566
28. Duan Y, Zhou Y, Zhu H, Li Z, Jin X, Tang D. Selective Frequency Mixing in a Cascaded Self-Raman Laser with a Critical Phase-Matched LBO crystal. J Lumin (2022) 244:118698. doi:10.1016/j.jlumin.2021.118698
29. Warrier AM, Lin J, Pask HM, Mildren RP, Coutts DW, Spence DJ. Highly Efficient Picosecond diamond Raman Laser at 1240 and 1485 nm. Opt Express (2014) 22(3):3325–33. doi:10.1364/oe.22.003325
30. Bai Z, Zhang Z, Wang K, Gao J, Zhang Z, Yang X, et al. Comprehensive thermal Analysis of diamond in a High-Power Raman Cavity Based on FVM-FEM Coupled Method. Nanomaterials (2021) 11(6):1572. doi:10.3390/nano11061572
Keywords: high-power, high repetition rate, picosecond, laser amplifier, Nd:YVO4
Citation: Bai Z, Zhang Y, Lin W, Yan X and Fan Z (2022) High Stability Industrial-Grade Nd: YVO4 Picosecond Laser Amplifier With High Average Output Power. Front. Phys. 10:876222. doi: 10.3389/fphy.2022.876222
Received: 15 February 2022; Accepted: 01 April 2022;
Published: 27 April 2022.
Edited by:
Yufei Ma, Harbin Institute of Technology, ChinaReviewed by:
Haiyong Zhu, Wenzhou University, ChinaChaitanya Kumar Suddapalli, The Institute of Photonic Sciences (ICFO), Spain
Copyright © 2022 Bai, Zhang, Lin, Yan and Fan. This is an open-access article distributed under the terms of the Creative Commons Attribution License (CC BY). The use, distribution or reproduction in other forums is permitted, provided the original author(s) and the copyright owner(s) are credited and that the original publication in this journal is cited, in accordance with accepted academic practice. No use, distribution or reproduction is permitted which does not comply with these terms.
*Correspondence: Zhenao Bai, YmFpemhlbmFvQGhvdG1haWwuY29t; Zhongwei Fan, ZmFuemhvbmd3ZWlAYW9lLmFjLmNu