- 1Department of Physics “Ettore Pancini”, University of Naples Federico II, Naples, Italy
- 2National Institute for Nuclear Physics, Division of Naples, Naples, Italy
- 3Accelerators and Applied Superconductivity Laboratory—LASA, National Institute for Nuclear Physics, UdR Milan, Milan, Italy
- 4Department of Physics, University of Milan, Milan, Italy
- 5Institute of Applied Sciences and Intelligent Systems, National Research Council, Milan, Italy
- 6University of Rome Sapienza, Rome, Italy
Free-Electron Lasers are a rapidly growing field for advanced science and applications, and worldwide facilities for intense field generation, characterization and usage are becoming increasingly popular due to their peculiarities, including extremely bright, coherent, wide band tunable ultra-short pulses which are not achievable with other techniques up to now. In this review we give a thorough survey of the latest advances in the Free-Electron Laser-based field generation and detection methodologies and then present the main characteristics of a future THz/IR source, named TerRa@BriXSinO, based on a superconducting linear accelerator. The foreseen source is strongly monochromatic, with a bandwidth of 1% or smaller, highly coherent both transversally and longitudinally, with extreme versatility and high frequency tunability. After introducing the most recent and novel FEL-assisted scientific investigations, including fundamental explorations into complex systems and time-dependent interactions and material dynamics, we present our vision on the potential use of the TerRa facility and analyze some possible applications, ranging from non-linear physics under extreme conditions to polarization sensitive imaging and metamaterial-based sensing.
Introduction
Free-Electron Lasers (FELs) physics and related technology is a rapidly growing field for advanced science and applications and currently represents a major subject of research worldwide. In the last 20 years FELs have demonstrated a great potential for tackling global issues in energy, transport, medicine, and bio-matter areas. Their widespread use in a desirably near future will be creating a range of novel opportunities achieving significant advances and extending the boundaries of different fields, from life sciences to novel investigations into condensed matter. FELs in fact combine unique capabilities and peculiarities with short pulse length, high brightness, monochromaticity and spatial coherence. The radiation produced by FELs show properties similar to optical laser light together with an ultra-broad frequency tunability, covering from X-rays to the microwave regime. A major advantage is that FEL emission consists of extremely intense, ultra-short, monochromatic radiation pulses allowing atomic-level detection of structural and functional properties with exceptional time resolution. Therefore, radiation produced by FELs in the X-ray, Ultra-violet (UV), visible (VIS), infrared (IR) and Terahertz (THz) region presents characteristics inherently different from the mainly used techniques such as synchrotron sources, Fourier Transform InfraRed (FTIR) systems and laser-based THz emission sources. As such, the development of FEL-assisted experiments, and the possible breakthrough in many scientific challenges of multi-disciplinary interest, requires a combined expertise in accelerator and laser science and related technologies to cover the various zones of the electromagnetic spectrum. In the X-ray range the pulse length can reach the limiting value of about 10–14 s and less, which is more than 103 shorter than the synchrotron generated radiation, while achieving a peak intensity (brightness) 109 greater. Due to the extremely high brightness and the ultra-short pulse durations, the measurements are unaffected by atomic motion, allowing a non-invasive material investigation. Data acquisition in fact can be completed in fs periods of irradiation, eliminating the radiation damages even under high irradiances. This becomes an important advantage for spectroscopic and imaging analysis of radiation (invasive) sensitive protein structures, soft tissues, and biological material. Apart from the ultra-high brightness, the capability of coherent detection and wide tunability obtained with FEL sources gives incomparable advantages upon well studied FTIR spectroscopy. For example, changes in atomic structure during protein function occurs at nano to femto-second timescale which is far short to achieve with conventional methods. Similarly, terahertz science, which keeps the peculiar properties of many materials in the spectral band of interest, benefits from both the high intensity electric field generation and the ultra-wide spectral tunability achieved by using FEL based generation methodologies. In standard THz spectroscopy based on photoconductive antenna (PCA) or nonlinear crystal emission, the most common methods used potentially covers the range 0.1–10 THz, but indeed the bandwidth is upper limited to six THz for the most part of optical instrumentation. Whereas, using FEL assisted techniques, the peak THz wavelength can be tuned over a range larger than 40 THz, allowing a stronger bridge between the photonic and electronic approaches. An extremely important feature of THz FELs is due to the operation with superconducting (SC) linear accelerators (LINACs) which can deliver high quality beams during all the acceleration time. The temporal structure of electron beams is characterized by a continuous train of pulses, and a repetition rate that can reach values of few hundreds of MHz. FEL generated beams are ideal to conduct diverse types of ‘pump-probe’ experiments, including THz pump/X-ray probe (or vice versa X-ray pump/THz probe), when operated together with an independent X-ray source as, for instance, an Inverse Compton Scattering (ICS) source alimented by the same electron beam. Moreover, the given electron beam manipulation techniques make it possible to tailor the THz band characteristics (narrow-band or broad-band) to be used for specific THz-pump/THz-probe measurements. The flexibility of combining diverse radiation sources is extremely beneficial for a multitude of experimental scenarios where an element-specific external (X-ray, IR and THz) excitation is needed combined with a corresponding surface/interface-sensitive suitable detection. In combination with the given radiation types, it is also possible to conduct time resolved pump-probe experiments with atomic level spatial resolution and femtosecond time resolution. As such, FEL assisted radiation exhibits superb spatial coherence, opening new perspectives for imaging techniques. For example, high-resolution 3D imaging of bio-systems and even single-molecule imaging by using polariton chemistry is a challenging objective which is prone to an enormous scientific impact. The study of exotic states of matter, characterization of materials under extreme conditions (deformation mechanisms, phase change dynamics, crystallization and polymorphic transitions) and observation of their evolution at ultra-short time scales, mapping the internal details of viruses and bacteria, decoding the molecular composition of cells and bio-materials, real time monitoring of chemical reactions, are just a few other examples to name in order to show the ground-breaking potential of FEL science.
In this paper, we aim to show some examples of free electron laser-assisted spectral and imaging applications in the THz/IR range that can be pursued using the future superconducting BriXSinO source. After this brief introduction, we summarize in Section 2 the possible THz radiation (with extension in the Far Infrared Region) mechanisms putting a special emphasis on the undulator based techniques. In Section 3, the FEL source Terahertz Radiator (TerRa) in the framework of the BriXSinO superconducting accelerator, TerRa@BriXSinO, is described. In Section 4 several possible detection systems are analyzed. Then, in Sections 5, 6, 7 and 8 a selected list of interesting and novel applications using the TerRa radiation is presented. A last session with conclusions closes the paper.
Radiation Generation
The THz frequency range lies between the radio and infrared bands, combining the photonic and electronic approaches within the broad electromagnetic spectrum. With the growing technological advances in THz science, this frequency window has been extended to include part of the IR spectrum. The recent “Terahertz Science and Technology Roadmap” broaden a prior definition of THz band (0.1–10 THz) setting its frequency range up to 30 THz [1].
THz pulses with ultra-strong electromagnetic field are of increasing interest, due to their growing potential for probing and controlling a variety of complex phenomena ranging from fundamental processes of molecules and nanostructures to phase transitions in solids and dynamical interactions in biomaterials. Laboratory scale laser-based sources with moderate frequency band (<10 THz) and mid-range pulse energies (<µJ) are widely used in the broad scientific community due to the ease of use, cost effectiveness and commercial availability. Nevertheless, new scientific attention is given to large-scale accelerator-based sources with ultra-high pulse power (MW) coupled with a wide tuning range (<60 THz) and extremely fast repetition rates. Being prone to many novel scientific explorations through photon-matter interactions, extremely bright, coherent, widely tunable ultra-short, monochromatic pulses open new possibilities to fundamental physics of complex systems and to material structural analysis, including time-dependent interactions, nonlinear effects, and dynamics of different compounds. In this context, the THz science becomes a valid tool including the infrared and Raman spectral analysis but with superior properties due to the high intensity and coherency properties.
There is a fast-growing demand for FEL facilities due to their tunability and high spatial and temporal resolution. There are more than 30 FEL facilities actively used worldwide for scientific research, many of them including various user stations operating in different frequency regimes ranging between X-rays and THz waves. A detailed list of FELs and their characteristics are summarized by Neyman et al. [2]. A more recent status with the technical specifications of these facilities can be found at the Virtual Library web link [3] maintained by Ramian et al. from the University of California, Santa Barbara. Moreover, a frequently updated list is edited by Klopf et al. from the Helmholtz-Zentrum Dresden-Rossendorf [4].
Regarding specifically the THz band regime, an up-to-date list of operational facilities covering THz/IR band and main characteristics of interest (repetition rate, maximum micro-pulse energies, minimum pulse width) are given in Table 1. From the table, one can deduce that FELs operating in the high THz frequency band (>6 THz) are limited. In the following subsections, among all current THz sources, we will concentrate on high-field THz sources briefly summarizing the most common generation methodologies and comparing their scientific and technological capabilities with undulator based THz facilities.
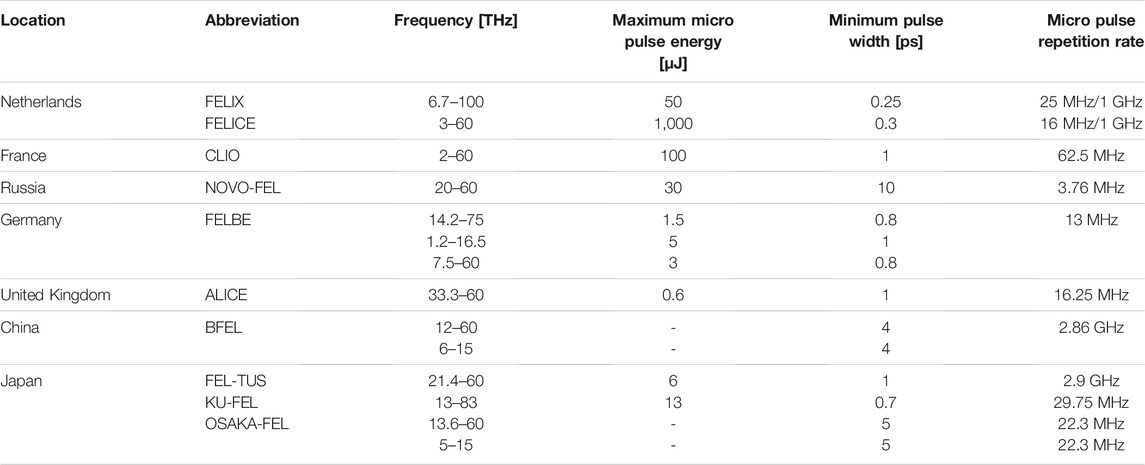
TABLE 1. THz/IR FEL sources worldwide. Parameters taken from [4].
Conventional Terahertz Sources
Since the very early stages of THz technologies, femtosecond laser-based techniques have been widely used to generate coherent pulses, enabling Time Domain Spectroscopy (TDS). Photoconductive switches [5–7] and optical rectification [8, 9] are the most widely used techniques due to commercial availability, cost effectiveness and easy adaptation to different experimental schemes. On the other hand, to satisfy the demand on wide band, high-intensity THz pulse generation, a large variety of techniques has been developed following the technological advancements in femtosecond laser systems. Some major sources to name are Schottky diode mixers [10, 11], backward wave oscillators [12–14], quantum cascade lasers [15, 16], nanosecond gas lasers [17] and gas plasmas assisted THz generation [18–20]. The main limitations of these laser driven THz sources stem from the emission intensities (<µJ) and/or the reduced frequency bandwidth (<10 THz) related to the intrinsic absorption characteristics of the nonlinear conversion media used. In this review the laser driven generation/detection methodologies and applications based on the mid-band THz radiation are not covered. The interested readers can find a detailed review on the metrology techniques in [19, 21] and on the broad mid-band applications in [1, 22–25].
Accelerator-based sources generate coherent high-field THz radiation from ultra-short relativistic electron bunches. Linear accelerators are used to generate electron bunches that can radiate a fully coherent (super-radiant) THz radiation in proportion with the number of electrons emitting radiation in phase. The super-radiant and the high field THz emission in these sources require ultrashort electron bunches or density-modulation at THz frequencies [26]. Some major examples of this type of interactions are radiation produced by undulator [27, 28], magnetic dipole [29], transition [30, 31], diffraction [32], Cerenkov [33, 34] and Smith–Purcell [35, 36] effect. One of the greatest benefits for novel experimentation using electron bunch triggered radiation is that the intense THz emission becomes self-synchronized with all radiation types (from IR to X-ray) emitted with the same electron bunch [37]. This also allows the use of coherent THz pulses for electron beam diagnostics and to reconstruct the charge distribution of the electron bunch [45]. Generating short electron bunches is the most essential point for achieving a high peak THz power. Coherent Transition Radiation (CTR) with high pulse energies reaching mJ levels [31, 38] can be generated via colliding the ultra-relativistic electron bunches with a metal screen [39–41]. Electric field magnitudes exceeding 10 MV/cm level and covering a very broad spectral windows can be reached with this technique. TELBE, FLUTE, FLASH, FERMI, LCLS, and SPARC are some examples of large facilities using this type of CTR modules [42]. A similar mechanism for high field THz emission is the edge radiation [43, 44] generated by directing electrons through a longitudinal magnetic field gradient. Edge radiation-based THz generation attains energies up to 10 μJ, giving a peak electric field up to 3 MV/cm. Even if the edge radiation keeps similar field properties, with the CTR based generation mechanism the THz signal can be completely synchronized and made phase stable with an accelerator-based X-ray source, as both sources are generated by the same electron bunch. This allows a convenient combination of THz/X-ray pump/probe experiments. A similar technique has been implemented at FLASH, reaching pulse energies around 1 μJ with electric field intensities up to 900 kV/cm [42].
Free-Electron Laser Based Terahertz Sources
Another mechanism for THz generation is the FEL emission by an electron beam driven through an undulator. With this technique pulse energies up to mJ range can be obtained. The first demonstration was held at FLASH [45] by using a nine-pole electromagnetic THz undulator. Fully synchronized and phase stable (fs levels) THz radiation is emitted reaching pulse energies up to 100 μJ with peak electric fields up to 1 MV/cm [37]. The THz FELs can operate in various kind of configurations: seeded THz FEL amplifiers, Self-Amplified Spontaneous Emission (SASE) THz FELs, short-pulse super-radiant THz facilities and multi-pass oscillators.
Mainly, IR, Far-IR (FIR) and THz FELs are designed to operate as oscillators. Examples of IR and FIR oscillators are FELIX, ELBE FELs, FLARE, ALICE and Novo-FEL. The advantages of this operational mode are the compactness, relaxed requirements for the quality of electron bunches, and the fact that oscillators are suitable for SC-LINACs, enabling the generation of powerful quasi-Continuous Wave (CW) light. Differently to other schemes, FEL oscillators require a stable repetition rate of electron bunches with very low jitter.
A comparison of the main beam characteristics grouped for different type of sources is given in Table 2.

TABLE 2. Beam characteristics comparison for various sources (modified from [42]).
The BriXSinO Project
The BriXSinO project is developed by INFN (Istituto Nazionale di Fisica Nucleare), Section of Milan in collaboration with INFN, Section of Naples and Italian National Institutions and Universities, and it will be located at LASA (INFN and University of Milan, Italy). The nominal characteristics foreseen at BriXSinO for the electron beam and for the undulator are given in Tables 3 and 4.
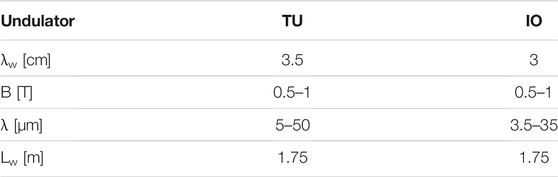
TABLE 4. TerRa@BriXSinO parameters of the undulators with permanent magnets, variable gaps, and linear polarization. First module is the Terahertz Undulator (TU). Second module is the Infrared Oscillator (IO). λw undulator period, B peak on-axis magnetic field, λ emission wavelength, Lw length.
The BriXSinO project was primarily intended as a demonstrator for the physics of the two-pass two-way acceleration scheme using SC cavities [46, 47]. After being accelerated up to 22–40 MeV, the 50–200 pC electron beam is sent into an arc, whereby it retraces the accelerator in the opposite direction. Depending on the entrance phase, the electron beam can give the energy back to the SC as an Energy Recovery Linac (ERL) or being further accelerated. In this way, the energy deposited by the electron beam to the dump can be limited and controlled. In the zero dispersion zones of the arc, the electron beam finds on one side a Fabry-Peròt assisted Inverse Compton source and on the other one a THz FEL oscillator. The two radiation sources can be alimented either independently or in sequence, thus generating two synchronized radiation pulses in two different ranges of frequencies, namely X-rays and THz. The combination of limited space and low peak current together with a high repetition rate - up to 100 MHz - and high stability in beam energy has led to design the THz source as a FEL oscillator: i.e., the FEL undulator is placed inside a cavity whereby the radiation is re-circulated and high average THz power is realized.
Due to the wide versatility of BriXSinO’s beam lines, it exhibits strong potentiality as user facility for both X-rays and THz radiation.
The scientific case of the FEL source TerRa indicates strong interest in radiation of wavelengths between 10 and 50 μm (3–17 THz). The BriXSinO accelerator line delivers a 50–200 pC electron beam with energy between 22 and 40–45 MeV, as shown in Table 3. Taking the highest energy values and assuming an undulator with a period λw equal to 3.5 cm, from the resonance relation:
λ = λw(1 + aw2)/(2γ2) Eq. 1
(where aw = 0.657 B(T)·λw (cm), γ the Lorentz factor of the electron beam).
radiation having wavelength λ ranging from 10 to 60 µm (3–20 THz) can be generated with a peak efficiency (evaluated as the ratio between the Extra-Cavity radiation energy and the energy of the electron beam) of 4.4·10−3 around 20 µm.
The FEL Oscillator TerRa@BriXSinO source has been designed as the sequence of two undulator modules, separated by a drift where a quadrupole, phase shifters, diagnostics and correctors are allocated, and embedded into an optical cavity equipped with mirrors suitable to the considered frequency range. The segmented undulator TU&IO, whose pictorial view is represented in Figure 1, is made of two different sections that could be also characterized by different periods: TU (Terahertz Undulator) and IO (Infrared Oscillator). They can work either tuned at the same wavelength or separately, delivering different wavelengths.
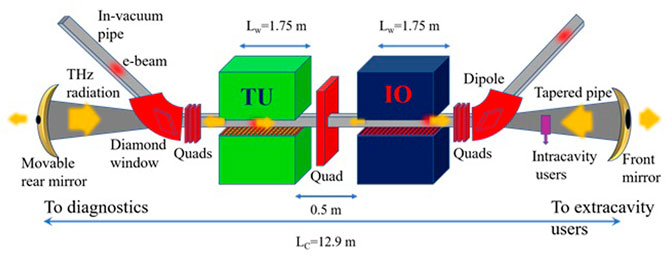
FIGURE 1. Schematic of the TerRA@BriXSinO source, with details of the segmented undulators TU&IO. The radiation produced by the electrons in the undulators is recirculated inside a cavity of length Lc = 12.9 m. The undulator modules are 1.75 m long and separated by 0.5 m where a quadrupole, phase shifters, diagnostics and correcters are located. The elements are not in scale.
The numerical modeling of the TerRa source has been carried on by using the three-dimensional, time-dependent FEL code Genesis 1.3 [46]. Starting from the electron beam parameters listed in Table 3, we have injected into the undulator a sequence of randomly prepared electron beams different one from each other both microscopically and macroscopically, to simulate the fluctuations of a bunch train. The study has been performed for radiation wavelengths between 10 and 50 μm, with an undulator having λw = 3.5 cm. Table 5 reports the main properties of the radiation at different wavelengths. As shown in Figure 2, single shot power in time (Figure 2A) and spectrum vs wavelength (Figure 2B) appear to be single spiked. Intra-cavity (IC) single shot radiation energy of 355 µJ (meaning 17.7 µJ of extra-cavity (EC) energy and 1.77 kW of average output power) at λ = 20 µm has been estimated. The option constituted by two undulator modules of different period allows therefore to obtain two different wavelengths with the same electron beam [47].
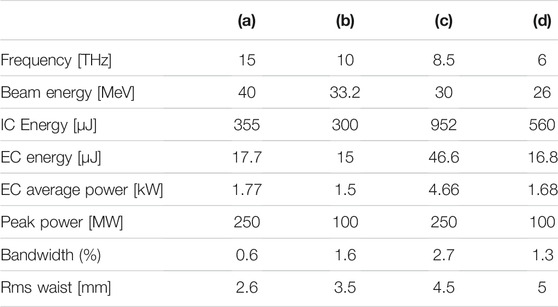
TABLE 5. Properties of the intra-cavity (IC) and extra-cavity (EC) radiation at different frequencies for TerRa@BriXSinO, assuming beam peak current of 10 A (a), 10 A (b), 20 A (c), 25 A (d).
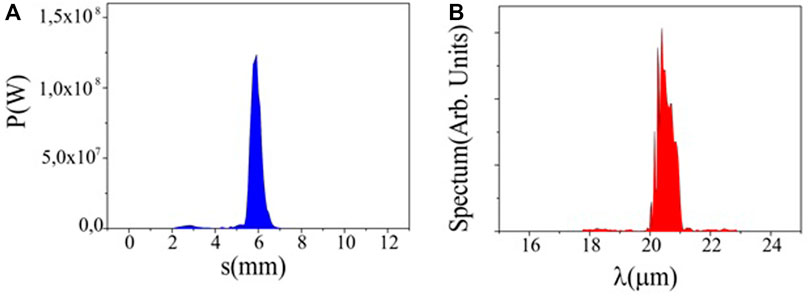
FIGURE 2. Radiation properties of the TerRA@BriXSinO source. (A) The Intra-cavity longitudinal profile of the power for a single shot of 355 mJ and (B) the spectral profile, when the two undulators (TU&IO) are in tune for an emission wavelength of λ = 20 μm at the saturation point of the oscillator.
Regarding the stability and coherence properties, the THz radiation is almost fully coherent and widely stable both in space and in time. The X rays produced by the Compton back-scattering in the other beam line of the device is temporally incoherent but can acquire a partial transverse coherence length of about few microns after meters of propagation.
The BriXSinO project is going to open new research opportunities for the run of high field two-color THz spectroscopy measurements or X-ray pump/THz probe experiments.
As a further option, the THz source could be coupled to a laser synchronized with the oscillator. A pump laser delivery station with photon energies in the three main bands, NIR, VIS and UV, could be installed in a dedicated area. The possibility to investigate the temporal recovery scale of different excited states of the sample in time resolved pump-probe experiments is directly related to the performance of the pump laser in terms of photon energy, time duration and jitter as well as pulse energy and repetition rate. The pump lasers will be distributed at the end-stations and carefully synchronized with the FEL beams by means of a dedicated synchronization unit. A 1 MHz repetition rate or higher will be obtained. A possible way to accomplish this option should be to operate with a laser system delivering 800 nm, 15 fs pulses aiming at a pulse energy of 0.2 mJ or larger, in burst mode (4÷5 MHz). An optical parametric amplifier and a second/third harmonic generation box would convert this wavelength into the desired pump wavelength throughout the UV-VIS-IR ranges (ca. 200—2000 nm).
Detection
In the framework of FEL applications, the detection and characterization of high field, large bandwidth electromagnetic signals having ultrashort time duration are of extreme importance and represent a challenge. The commonly used electronic devices and/or detectors do not satisfy the need for such advanced signal responses, due to the technical low capacity in terms of the rise times (ps level) and relatively low response times (ranging from ns to µs). The possibility to simultaneously measure pulse duration and intensity profile temporal response, and to monitor electric field evolution, is extremely crucial for phase and amplitude sensitive spectral measurements.
The electro-optic (EO) sampling is one of the most intensively used and proven candidates for high intensity THz/IR emission characterization. This technique also combines the advantage of the possible characterization of electron bunches. Optical pulses in the visible and NIR laser sources can reach ultra-high pulse durations (few tens of fs), that are much smaller than the oscillation period of the THz electric field (few hundreds of fs). Thus, THz sampling with such sources can be achieved with high resolution. EO techniques are based on the Pockels effect, based on the refractive index change of crystal media used in the presence of the THz electric field. The THz electric field induces a transient birefringence and therefore a phase shift between polarization components in these optically active crystals. THz and laser beams are superimposed in the EO crystal, and the transmitted electric field detected by using balanced photodiodes in conjunction with a Wollaston prism, as shown in the schematics of Figure 3.
In detecting high field THz beams using EO sampling there are a few technical considerations that one should consider. First, lattice symmetry [48] and thickness [49] of the EO crystal are important parameters affecting the sensing performance and the achievable bandwidth of the impinging electric field. The intrinsic optical phonon resonances of the crystal are also important and should be taken into account since THz frequencies in proximity are prone to strong absorptions and reflections. For example, resonances are found at 5.3 THz in ZnTe and 11 THz in GaP. Moreover, they correlate also with an anisotropic index of refraction profile within the media, producing a strong variation in between phase and group velocities of the probe pulse inside the crystal and limiting the temporal resolution [50]. Similarly, the dispersion of the probe pulse would significantly distort the THz time domain profile. These effects can be minimized by using a thin crystal media, at the expense of reduced intensity profile, or compensated by model combined EO detection methodology [51].
The TELBE THz facility consists of two super radiant THz sources where a sequential EO sampling is implemented for ultrafast benchmark experiments for pulse- and field-resolved THz diagnostics. ZnTe and GaP are the most commonly used crystals for EO sampling of broadband high THz fields. The interested readers may find a comparative work authored by Wu et al. [52] on the properties and the EO detection performances of commonly used nonlinear crystals for FEL assisted sources, and a thorough investigation into terahertz pulse EO sampling abilities of various crystals (including CdTe, DAST, GaAs, GaP, and ZnTe) in [53].
It is very important to note that the EO sampling technique can be also used for measuring the longitudinal electric field distribution of electron bunches with a sub-ps time resolution [54]. The given approach is realized at HUST (Huazhong University of Science and Technology) THz-FEL facility for sampling both the coherent THz electric field and the field distribution of the electron bunches [51]. The system uses a mode-locked 10 fs laser at 800 nm with 79.33 MHz repetition rate as a probe pulse, actively synchronized to the accelerator RF clock (2,856 MHz) and achieving a jitter around 100 fs [55]. Analogously to the EO sampling of transient electric fields, phase mismatch, dispersive propagation and absorption affects the detection of the temporal profile of fs electron bunches [56]. The emission and focusing properties of the original electron bunch profile differ with respect to the transition and diffraction radiation [51, 57].
Another well-established technique is field-streaking, that is based on the photoelectron momenta change created in the presence of an impinging electric field [58]. Mainly streak cameras are used for this purpose, yet their resolution (limited to a few tenths of picoseconds) is not sufficient for FELs pulse durations (few fs). On the other hand, for longer streaking field periods needed for FEL sources correspond with THz and FIR frequencies. The use of THz field-streaking has been demonstrated at FLASH [45] where researchers have used THz pulses from an undulator for measuring the single shot X-UV pulse durations.
THz Air Breakdown Coherent Detection (ABCD) is a recent technique removing the bandwidth limitations [59]. This approach uses the third-order nonlinear frequency mixing in air or selected gases as the sensing media and is based on the same physical phenomena of field-induced second harmonic generation under two-color excitation [60]. The greatly reduced absorption and dispersion allows detection up to 30 THz. This technique is favorable in terms of synchronization for gas plasma generated THz field detection. The interested readers can find in two thorough reviews authored by Dai et al. a detailed introduction to the mechanisms [19] and potential applications [61] of the THz air photonics technique.
Advanced Applications
As already mentioned above, with the growing technological advances in THz science the current definition of THz band (0.1–30 THz) is merging with the IR radiation. In this context, the THz science becomes a valid tool including the infrared and Raman spectral analysis but with superior properties due to the high intensity and coherence characteristics. The FEL based THz/IR methods have superiority among the other types of IR emission and detection techniques [70, 71]. The output characteristics are MW range pulse power, pulse durations in the order of picoseconds (10–1,000 optical cycles), up to few GHz of micro pulse repetition rates, and the continuous and fast tuning over a wide range of wavelengths combined with coherent radiation properties. Infrared and THz radiation is of great interest for various applications and for studying fundamental mechanisms [62–66]. Just to name, some examples are: strongly correlating quantum systems, magnetic states in solids, complex fluids, bio-fluids, nanoscopic structures, membrane proteins, bio-molecules, functioning dynamics in bio-systems, materials chemistry, electronic transitions in semiconductors, including interband dynamics, phonon scattering and inter-sub band dynamics in quantum wells, inter-sublevel spectroscopy and polaron dynamics in quantum dots, polaron dynamics, coherent dynamics, spin dynamics, impurities in semiconductors, vibrations in crystalline solids and amorphous materials covering the local modes in insulators, vibrational modes of hydrogen-like atoms and molecules.
FEL assisted radiation sources are gaining extreme importance for novel exploration and fundamental understanding of material structures through photon-matter interactions. Tunable wavelength and coherent radiation are generated by using relativistic electron bunches directed through undulators. The beam packages produced by FELs are compressible to few fs pulses allowing to study the minute interactions and ultra-fast dynamics of different materials. A number of significant works across a diverse range of subject areas have been conducted within the last few years using pump-probe experiments at X-ray FEL facilities. The combination of IR/THz and X-ray pulses allows to study the temporal evolution of different complex atomic and molecular systems. THz/FIR region of spectrum is of extreme importance for structural biology, condensed matter, and molecular dynamics, yet it cannot be separated away from X-ray, extreme UV and optical spectrum studies.
In this section devoted to application we will first give to the readers an overall picture in terms of the fundamental and material characterization studies done by FEL-assisted emission sources, covering the subject presented above. In the second part we will present a brief review on low band THz applications covering the biomedical and life science. Lastly, as a recent and novel approach, we will give a glance to the polariton chemistry, which combines the bio-medical and chemical structures of materials of biological interest with the fundamental physics and chemistry. We will focus on the mechanisms and possible future applications of this promising technique.
Fundamental Studies and Condensed Matter Applications
The inter-band processes occurring within the conduction band are more sensitive in the FIR frequency region. While the inter-sub-band transitions above Longitudinal Optical (LO) phonon frequencies have their sub-picosecond relaxation in the Mid-Infrared (MIR), relaxation mechanisms below this frequency can be observed in the THz range. The relaxation lifetime was first time measured based on the saturation detection utilizing a FEL source [67]. The transition selection rules are polarization sensitive. To this aspect the polarization sensitiveness of coherent THz radiation becomes an important factor for identification of the electronic transitions in semiconductors and quantum dots. Inter-sublevel transitions within confined states, transitions between singlet and triplet states, and composite states transitions (polarons) all occur in the MIR/FIR regime of the spectrum. The high intensity and tunability for FIR/VIS wavelength double resonance capability of FEL assisted IR/THz measurements of inter-sub-level transitions allow to characterize the electronic structure of the materials. Spin polarized electron dynamics in semiconductor spin and spin-split electronic structures are often studied at MIR/FIR regime of the spectrum. For this reason, the pump and probe measurements with circular polarization at MIR/FIR wavelengths are prone to many applications and fundamental studies. Many of the major FEL facilities such as FELIX, JFEL, FELBE, CLIO provide FIR output with simultaneous coverage of MIR (around 3–250 µm in wavelength) [68]. The wide wavelength tuning makes it possible to achieve resonant pumping of discrete transitions and multi-wavelength spectroscopy. There are many intrinsic properties and phenomena occurring in this frequency regime and enabling time-resolved spectroscopy of weak absorptions and non-linear optical processes. Combined pump-probe experimental stations at the European XFEL are another good example for the powerful, coherent IR/THz radiation related research tool where the time structure is synchronized with X-ray FEL. The undulator generated radiation can reach up to 30 MeV of energies. The SASE-FEL mechanism provides a sensitive polarization control with wide band tunability (0.3 THz - 30 THz) with few hundreds of µJ pulse energies and up to 100 MW peak power. The future generation of FELs is prone to combine THz with VIS-UV and X-rays, together with complementary techniques such as strong magnetic fields and near-field techniques. The rapid tunability together with the high photon flux character of FEL beams allows to study the weak absorption by using action spectroscopy methodologies based on the detection of different chemical and physical processes occurring, such as the change in particle mass through fragmentation and/or disassociation, change in ionization state through photoconduction, and photoemission. Some examples of this type of specialized experimentation techniques are the ion-trap system at FELIX, the sum-frequency generation set-up at CLIO and the near-field optical microscopy at FELBE. Moreover, the spin dynamics can be extracted by using a circularly polarized variant of pump-probe methods.
The very high intensity beam characteristics of FELs made it possible to experimentally investigate advanced nonlinear optical dynamics such as the characterization into relaxation rates of electronic transitions in semiconductors by bleaching, exploring the heavy ion formations on surfaces by the frequency-sum generation and multi-photon absorption and the Franz–Keldysh type of strong-field effects [69, 70]. Having ultra-short pulse duration, FEL generated radiation enables to observe very fast decays like the electronic lifetimes of low energy excitations. Infrared-active vibrational transitions are of extreme importance to investigate energy distribution of phonon modes within the material. The excited density starting from the bleaching condition can be probed when the ground and excited state populations reach the near saturation by ultrafast high field pump-probe experiments.
Low energy transitions in semiconductors in relation with the electron dynamics are of great interest not only for fundamental physics but also for the development of novel optoelectronic devices. Notable phenomena include band-to-band transitions and transport confinement in semiconductors for quantum cascading, impurities, and spin polarized excitations in solids for the manipulation of the polarization states of matter. Understanding the electronic structures under extreme magnetic fields by using the cyclotron resonances are important for a deeper investigation into new materials, such as semiconductor nitrides and magnetic semiconductors for spintronic applications.
There have been many reports investigating the relaxation rate properties of various advanced materials such as HgCdTe [71, 72], PbSe [73, 74], InSbN and hetero-structures of InAs/GaSb [75] in pump–probe experiments. The phonon dynamics and electron-phonon interactions are studied by monitoring the absorption and decay processes [76]. High intensity pump-probe measurements on the GaAs/AlGaAs quantum wells at low temperature and on Si/SiGe super-lattices have shown the fundamentals behind the inter-sub-band relaxation mechanisms and their dynamics under temperature changes. There have been successful attempts of lifetime measurements by using novel active device structures which combines quantum well infrared photodetector technique within a waveguide structure to determine the transition energy by voltage modulated waveguide transmission [77]. The FEL source at CLIO is one of the pioneers in the field. Some cornerstone studies have been conducted on GaAs/AlGaAs coupled quantum wells, with four bound state sub-bands observed under two color excitations and the gain measurement performed with time-resolved pump–probe experiments by the FEL [78].
Very complex dynamics such as Franz–Keldysh effect between electron and hole pairs under a strong driving field, electron tunneling through the electrostatic confining potential at low frequencies, ionization by multiphoton absorption, high harmonic generation at mid-range frequencies, resonance enhancement and second order susceptibility dispersion leading to second harmonic generation at THz frequencies [79] are observed in the presence of intense FEL assisted THz electric fields [69, 80, 81]. Moreover, side-bands of the quantum confined states [82–85] can be triggered under strong THz electric fields revealing new virtual states [86–88], frequency doubling and tripling [89, 90], characterization of depolarization shift [91]. Using synchronized pulses with a two-color scheme, time-resolved spectroscopy of internal dynamics of excitons is allowed by tuning the THz frequencies at the transition frequencies.
Double resonance spectroscopy correlates the inter-band and inter-sublevel transition energies [92]. One can use the mapping of the double resonance FIR modulated photoluminescence spectroscopy for distinguishing the mechanisms from dot to dot in correlation with the size and composition distributions [93, 94]. The non-linear optics of intra-band transitions including the third-harmonic generation [95, 96] is associated with resonant intra-valence band transitions, second harmonic generation [97, 98] and two-photon absorption [99, 100]. Polaron dynamics [101, 102] has been studied with the absorption saturation technique [103] and pump–probe spectroscopy [104–107]. Spin mixing effects and spin-lattice coupling have been studied [108] using a THz probe to improve the fundamental understanding of magneto-transport mechanisms in metals [109].
Since optical transitions are in the FIR region, the very short coherent pulses attained using FELs are required for experiments. The high power and coherent pulsed characteristics make it possible to investigate the coherent dynamics of matter such as Rabi oscillations and more complex time-resolved, coherent, degenerate four wave mixing effects. In such a way damped optical Rabi oscillations of polarons have been observed [110]. Spin polarized electron dynamics in semiconductors spin and spin-split electronic structures are often studied at MIR/FIR regime of the spectrum. The inter-band non-radiative and spin lifetimes and the inter-band transmission and circularly polarized intra-band transitions dynamics can be probed by FIR FEL pump-and-probe experiments [111].
Mechanisms driven by intense THz electromagnetic fields in spin systems recently gained great interest in modern spintronics as a novel way to obtain ultrafast control in macroscopic magnetization. Observation on the reconfiguration dynamics of magnetic domain structures under high field THz FEL pulses by Kurihara et al. [112] has proven the possible use of realizing thermal spin effects at this frequency range. To irradiate the ferromagnetic domains of single crystal ErFeO3, authors use intense (10 mJ/pulse) THz pulses generated by the FEL. Findings are that the domain mechanisms near the boundaries can be locally reconfigured under the THz−FEL pulse irradiation.
In another experiment, Gauthier et al. [113] used a chirped pulse amplification technique for the compression of free electron laser pulses in the extreme UV range. This work has proven the importance of FEL techniques to explore fast electron dynamics. Usenko et al. [114] demonstrated the use of attosecond interferometric autocorrelation technique by using the self-amplified spontaneous emission of FEL to gain insights of phase-controlled pulses. De Ninno et al. [115] implemented an interferometric method in order to measure and control the spectro-temporal characters of single shot ultrashort pulses seeded by a femtosecond laser.
A novel technique to achieve high intensity two-color FEL pulses and probe ultrafast dynamics by using twin electron bunches has been proposed in the optical range at SPARC [116, 117] and then implemented in the X-ray range at LCLS [118]. A similar electron beam with two beamlets at relatively low energy (50–80 MeV) can be used in an Inverse Compton experiment to generate two color X-rays [119]. At BriXSinO, two color X-rays radiation can be obtained by passing the electron beam in a two Fabry-Peròt cavity Inverse Compton source [120]. An all-optical synchronization of the soft X-ray FEL pulses with ultra-fast rates less than 30 fs was achieved by Schulz et al. at FLASH [121]. Ferrari et al. [122] has demonstrated a novel configuration technique to achieve elemental selectivity by use of a two-color seeded FEL source in an independently tunable resonant-pump resonant-probe to specific electronic excitations. The origin of incipient ferroelectricity in lead telluride, correlated with coupling of band-edge electrons and phonons revealed by ultrafast X-ray scattering, was reported by Jiang et al. [123]. Gerber et al. directly studied the photoinduced lattice dynamics in BaFe2As2 by measuring the rapid lattice oscillations which can alter the electronic and magnetic properties of the material [124]. Symmetry breakdown of electron emission in photoionization of argon atoms and ions was demonstrated by Ilchen et al. [125].
Molecular Dynamics, Structural Biology and Bio-Medical Applications
During the years, THz spectroscopy proved to be a very useful and successful tool for the investigation of many processes in pharmaceuticals, biology, and bio-medicine, since it probes low frequency vibrational modes [126–129]. These vibrations define large scale conformational changes occurring along the torsional degrees of freedom in complex biopolymers [130–132]. Moreover, the fingerprints of many materials of biological interest lie in the THz frequency band. THz related energies correspond to intermolecular hydrogen bonds and are therefore an ideal probe for the investigation of the binding of water and hydration levels in large biomolecules. THz spectroscopy techniques are also widely used for the real-time detection of protein–water dynamics upon protein folding and for the study of the water surrounding biomolecules, including carbohydrates [133–136], small peptides [137–140] and DNA oligomers [141–144].
There has been an intensive work done in the literature for pharmaceutical material characterization [145–149], for monitoring intermolecular interactions in pharmaceutical formulation [150–152], and for investigating drug delivery mechanisms. The interested reader may find a detailed list of terahertz spectral signatures of crystalline pharmaceuticals of major interest summarized by Shen [149].
THz spectroscopy in both frequency and time domain have been also applied for tracing gases. THz spectral signatures for example in hydrogen cyanide (HCN) [153, 154], carbon monoxide (CO) [155, 156], formaldehyde (H2CO) [157, 158], and water (H2O) [159, 160] allow to identify and quantify the individual gases from the pure rotational transitions.
Since THz waves are non-ionizing, they represent a suitable option for noninvasive spectroscopy, imaging [161, 162] and in-situ monitoring [163, 164]. Since THz radiation is highly sensitive to polar molecules, it has been successfully used to determine the hydration levels in skin tissue [159], both in vitro and in-vivo [161, 165], in order to discern between tumoral and healthy tissues for cancer diagnosis [166–168]. In many bio-applications, a key factor is that the THz radiation is strongly reflected by conductive materials and strongly absorbed by polar liquids, on the contrary the THz waves can penetrate a large number of organic or inorganic materials which are opaque in visible or IR ranges. For this reason, THz spectroscopy and imaging techniques are ideal candidate for the detection of concealed (multilayered) materials and tissues.
Even if the given examples on imaging and spectroscopy show the great potential of the investigation in the THz range, the major part refers to works done in the low frequency range (<6 THz) and at low energy level (µJ) [169]. Many of the complex molecular investigations were complemented with the integrated analysis of THz time domain response, yielding structural information together with classical IR spectroscopy [170, 171]. In this context, the FEL-assisted THz sources covering both bands of the spectrum will throw aside the technical difficulties faced with infrared and Raman spectroscopy. Moreover, IR/THz pump-probe experiments satisfying wide range tunability with high peak powers, high spectral and temporal resolution, polarization control and the possibility of synchronization with the X-ray pulses will allow to study complex materials on atomic and molecular time scales [172–174].
Spectral Analysis of Biomolecular Aggregates
Self-assembly of peptides and proteins have important role in bio-function. Similarly, the dissolution method of peptide fibril is potentially useful in medical fields, as a modification technique of cell structure and as a fabrication tool of biomaterials. Using a novel approach Kawasaki et al. [175] recently proposed a physical engineering technology based on a THz FEL. Using microscopy analysis, authors observed a remarkable reduction in the β-sheet with increasing α-helix of calcitonin peptide fibril irradiated by the FIR-FEL, with respect to the MIR-FEL. Their findings show that intense terahertz waves can dissociate fibrous conformation of peptide with little influence of thermal effects, proving that high field FIR-FEL irradiation can be used as an innovative processing tool in medical research.
Spectral Analysis at Strong Coupling Regime
In the past few years, strong coupling between electronic molecular transitions and photonic structures or in other words between organic molecules and confined light modes has been shown to modify the electronic landscape of the molecules, changing their physical and chemical properties, and affecting the chemical reaction rates, electronic and excitonic transport properties. The so called polaritonic chemistry, with its promising potential to tailor chemical structure and reactions through the hybrid light−matter states, has recently emerged as an exciting new research field. This phenomenon was observed in various types of materials, such as cold atoms [176–178], excitons in semiconductors [179–181], phonons in inorganic crystals [182, 183], organic molecules [184–186], nanocrystals [187].
Squibb et al. reported the acetylacetone photodynamics at femtosecond regime by using a seeded FEL source in a photoexcitation and photoemission scheme [188]. Their work has given a better understanding of the earlier stages of the photochemical processes involved on extremely short timescales. Perakis et al. revealed the highly complex water dynamics and the influence of cage effects on ultrafast phenomena by sub-100 fs measurements conducted by FEL sources [189]. The structures of peptide fragments formed during electron transfer dissociation was reported by Martens et al. by using FEL-based IR spectroscopy techniques [140]. The demonstrated technique was used for sequencing peptides and proteins by fragmentation. Levantino et al. showed that it is possible to track the motion of myoglobin, observing the breakage of a bond between the protein and a ligand occurring within picoseconds of time interval during the photoinduced ligand release [190]. Nogly et al. demonstrated a novel approach for the study of the complete photocycle dynamics of retinal proteins, reporting time-resolved experiments for the structural identification of the light-driven proton pump bacteriorhodopsin [191]. In a work by Grünbein et al., the possibility of MHz rate high quality data acquisition was shown, allowing the noninvasive characterization of lysozyme crystals from proteins [192].
It is important to note that the strong coupling with inter-molecular vibrations occurring in organic materials is in the low terahertz region [193], whereas many of the fundamental excitations and collective modes in solids such as the free carrier Drude response, crystal lattice vibrations, charge density waves, transition gaps in superconductors, magnetic excitations, surface plasmon and phonon polaritons have characteristic energies in the FIR range. Analogously, FIR provides fingerprints of molecule specific vibrational modes such as macromolecules and polymer chains in many soft and biological molecular materials [203–206].
More recent works refer to different kinds of polymers, molecules in liquid phases, organic crystals and organic liquid crystals in various geometries, nanowires, nanographene, proteins, light-harvesting complexes, and photosynthetic bacteria hybridization and many more. Interested readers may find the cited works on strong coupling observed in exotic materials listed above in the short review given by Hertzog et al. [194]. Moreover, a thorough review on polaritonic devices, with a comparison between organic and inorganic materials, is given by Sanvitto et al. [195], that includes polariton lasing [196], bosonic condensation [197], Bose−Einstein condensation [198], nonlinear interactions [199] and optical response [200].
Imaging Applications
Accelerator-based radiation plays a major role in THz imaging applications, being capable to couple high spatial and temporal resolution with highly sensitive chemical state analyses, which are of major importance in bio-medical research on molecular dynamics in the living environment. Tunable, narrow band FEL sources allow the investigation of the sub-molecular aspects of biochemistry with superior analytical performance to investigate hard tissues even down to a single cell. In fact, most bio-processes are in the range of FEL pulse lengths, and time-resolved data on vibrational modes can be obtained [105, 201].
Ion beam microprobes such as particle-induced X-ray emission, Rutherford Backscattering Spectrometry (RBS), Particle-Induced Gamma-ray Emission (PIGE), or Nuclear Reaction Analysis (NRA), Scanning transmission ion microscopy, X-Ray Fluorescence (XRF) and X-ray Absorption Spectroscopy (XAS) at microscopic levels, X-ray diffraction microscopy, mid-infrared microscopy (FTIR) and THz radiation are used in a complementary manner to achieve the needs for imaging and spectroscopical investigations of bio-matter.
Single cell imaging of live cyanobacteria by using X-ray FEL source was demonstrated by van der Schot et al. The authors have recorded signals from free-flying cell samples with 4 nm resolution [202, 203]. Schneider et al. has successfully shown the possibility of in situ single-shot diffractive fluence mapping by using integrated gratings to reduce the uncertainty in the laser beam profile characterization [204]. Leshem et al. has reported the full image reconstruction by a direct single-shot phase retrieval acquired from the diffraction patterns [205]. This work proves that it is possible to obtain coherent diffractive imaging of non-crystalline objects such as single molecules.
Polarization Sensitive Imaging
Pakluea et al. [206] proved a new modality of THz imaging for polarization sensitive materials by using coherent THz transition radiation generated with short electron bunches of around 8 MeV from a 45° tilted aluminum target. Later, the radiation was used to create images with a reflection setup. The authors carried out a full characterization of backward transition radiation properties, including spatial distribution, polarization, and spectral radiation. Moreover, they made THz imaging of the flow channels plate of proton exchange membrane fuel by mapping separately the radiation of p- and s-polarization components. The result of their study is that polarized transition radiation can be used to improve the THz imaging contrast and realize a better THz image, with p-polarization providing the highest reflectance contrast.
Near-Field Nanoscopy and Scanning Near-Field Optical Microscopy
Nanoscopy and IR scattering-type Scanning Near-field Optical Microscopy (s-SNOM) provides imaging and spectroscopy at nanometer scales. These techniques can be adapted to cover a broad electromagnetic spectrum of NIR, MIR, FIR and THz frequencies. With recent advances in technology, they can be possibly implemented to low temperature and ultrafast regime studies.
As explained in detail previously, many molecular specific activities, electron, and lattice dynamics, and plasmonic and polaritonic effects in quantum matter lie within the THz and FIR regime of the spectrum. Up to date, s-SNOM detection spectral band was mainly limited in between the NIR and MIR regimes and/or low THz window, and it was not accessible through THz/FIR frequencies. Recently, a successful adaptation of an ultrabroadband FIR s-SNOM nanoimaging and spectroscopy has been demonstrated by Khatib et al. [207]. The authors have combined an IR synchrotron radiation with a novel low-noise fast modulating Cu-doped Ge photoconductor. Using this approach, they exceed conventional limits by achieving ultrahigh spatial resolution (30 nm) at wavelengths up to the THz/FIR spectral window.
Programmatic Use of the BriXSinO Terahertz/Infrared Beamline
In the previous sections we have given a comprehensive survey of the main FEL assisted terahertz sources and their applications. Even if up to date FEL sources have speed up the scientific exploration, the goal of more intense sources has not been achieved yet. The BriXSinO project is expected to fulfill this demand. In the following we present a perspective on the potential use of the Milan facility, including a vision on some possible applications that may come true soon in a programmatic manner.
Non-Linear Physics Using the BriXSinO Source
In the recent literature, the possibility of combining near-infrared optical pulses and intense, sub-ps, broadband terahertz pulses to generate a THz-optical four-wave mixing in the investigated material has been successfully demonstrated by Rubano et al. [208]. In this novel technique the frequency spectrum of the generated signal was observed to display two intense sidebands close to the optical second harmonic central frequency 2ωL, where ωL is the optical central frequency of the near-infrared fundamental beam. The two sidebands are peaked around the central frequencies ωs,a = 2ωL ± ωT, where ωT is the THz central frequency. These two frequencies are analogous to the Stokes and anti-Stokes components appearing in standard hyper-Raman scattering, leading to a coherent THz Hyper-Raman (THYR) effect.
Despite the resolution in the frequency domain being poor due to the large bandwidth of the initial pulses, yet it is possible to observe the strong oscillations following the time domain evolution of the THz signal. The latter are due to the resonant coupling of the THz pulses with the proper frequencies of the material. It was demonstrated in crystals that the THYR signal carries information on a large variety of low-energy excitations including polaritons and phonons far from the Γ-point.
This phenomenon was first time observed by means of a table-top laser-based THz sources, thus making the observation of this nonlinear optical effect challenging due to the limited intensity per pulse. The high intensity and wide band tunability offered by the THz BriXSinO source will allow to extend this novel technique for more advanced material investigation and reveal new concepts in the nonlinear physics. Moreover, recent studies predict new nonlinear hyper-Rayleigh and hyper-Raman scattering mechanisms, which are specific of the interaction with chiral molecules by use of twisted photons [209]. This can be done adding a helical phase to the beams participating to the Hyper-Raman process, making this new technique very appealing for bio-sensing and microscopy of chiral biological samples.
Imaging Using the BriXSinO Terahertz/Infrared Source
Imaging using intense THz sources can be applied in many processes involving the photoelastic effect. The effect can be essentially described in terms of a permanent or transient birefringence induced in the material by the action of some internal/external force. All dielectric media in principle can show photoelasticity, but besides the study of the fundamental physics of this effect, it was predominantly used as a tool for studying and experimentally mapping the forces/stresses applied into a given material. This is particularly important and useful around discontinuities in the material, where those forces are strong and the material can have weak points (critical stress points), and in general for irregular geometries, where calculations can be cumbersome and less reliable. Naturally, the material under investigation must be, at least partially, transparent to visible light, and indeed this technique has been applied successfully for many years on studying relaxations/deformations dynamics in glasses and plastics.
In the case of a material which is not transparent to visible wavelengths, one immediate solution can be found by changing the wavelength itself. This is not always easy or straightforward as it may seem, because one should match the very high technological level which has been reached on visible light (both in terms of sources, manipulation, and detectors). Anyway, modern technologies, developed enough to make this technique possible, are available today. THz waves seem particularly interesting in this context, especially when powerful sources such as BriXSinO will be developed. The photoelastic effect requires a crossed polarizers configuration. Only a small portion of light, whose polarization has been affected by the material birefringence, will be transmitted, and therefore strong sources (and sensitive detectors) are key to make the experiment practically possible. One intrinsic feature of the THz wave is its high transitivity through optically opaque materials, making it a suitable radiation range to investigate the internal properties of these materials. Today, the research field of THz photoelasticity is rapidly growing, but still relatively unexplored. The proven ability of THz radiation to distinguish between different kinds of human bone tissue could be extremely beneficial to study the osseointegration process in real conditions.
The research proposal is to implement in the BriXSinO facility a THz photoelastic setup, with the aim of studying dental implants in animal (and, in a future, human) bones. The implants can be studied at first on dead animals (no osseointegration), then in dead animals which have been implanted a given time before death (study as a function of the time of osseointegration process) and finally they can be measured in-vivo, which is a crucial step to achieve the possibility to study real human implants. This last step presents some difficulties, due to the large amount of soft tissue surrounding the bone, which could absorb the THz radiation to a level in which the experiment is not feasible anymore. In order to circumvent this problem, the only solution is to increase the source power (that is why the BriXSinO source is an inevitable choice), to increase the sensor sensitivity and to search for wavelengths which are less affected by absorption. Once the experimental procedure will be validated, it will be possible to study different clinical protocols: delayed or immediate load, with all possible variations among those two cases (different healing times/drugs in the former, different load conditions in the latter, the number of supports for each implant, and so on). This approach will be extremely beneficial for the whole community working in this area.
Hybrid Approaches and Metamaterial Research Using the BriXSinO Terahertz/Infrared Source
Small Angle X-ray Scattering (SAXS) using a THz transparent 3D printed microfluidic cell was first presented by Schewa et al. [210]. X-ray studies reveal THz-induced, nonthermal changes in the structure of crystallized proteins, where the excitations of large molecules are associated with THz and FIR radiations. Using a microfluidic cell for the experiments, authors combined SAXS with an external THz field to determine the collective vibrational modes. Even if the authors have successfully demonstrated the main concept, the idea halted because of the lack of high field and more broadband THz sources. TerRa@BriXSinO can be the solution.
Photonic metamaterials are another concept of main interest, where tailored micro-structured subwavelength building blocks allow the light manipulation and the development of unconventional electro optical properties such as unnatural, metal level refractive index, zero reflection, perfect absorption and enhanced nonlinear phenomena. Main future advances are expected to focus on the possible applications into ultra-high-resolution imaging beyond the diffraction limit and in polarization based devices [211].
One of the major future perspectives for THz science and technology is focused on developing rapid, label-free, and cost-effective sensing applications for bacteriology and virology Research and analysis. THz spectroscopy gained extreme importance for the fast racing and qualification is needed including the morphological alteration monitoring of cells. All these innovative works show the importance of THz bio-matter interaction in the near future. The interested readers may find a full comparison of THz spectroscopy with common bacterial detection methods from the review by X. Yang et al. [212]. Main challenge however is the limited sensitivity, due to the THz wavelength being far larger than cells/viruses size (a typical bacterial cell is on the order of ∼λ/100 with respect to THz range), however it can be resolved by engineering suitable metamaterials with an improved coupling in between the living matter and THz radiation [213, 214]. In any case of these scenarios the design structure and/or the organisms under investigation stems from the highly absorptive nature of water and or the construction materials of the meta-devices where the strong attenuation of THz radiation starts playing the main role in the detection precision. Given these limitations for standard TDS, the BriXSinO THz/IR Source with high intensity and tunability characteristics will be a complementary technique not only for research on bio-systems (where water absorbance becomes a major problem) but also for sensing applications where metamaterial-based THz devices are involved.
Conclusion
In this article, we gave a comprehensive survey of the main FEL-assisted terahertz sources worldwide and presented the main characteristics of a future THz/FIR source based on a superconducting linear accelerator. The source is constituted by a FEL oscillator, alimented by a 100 MHz electron beam of 22–40 MeV of energy and 50–200 pC of charge. The wavelength of the radiation is between 10 and 60 µm with a peak of efficiency around 20 µm. Intra-cavity single shot radiation energy of 355 mJ (meaning 17.7 µJ of extra-cavity energy at 100 MHz and 1.77 kW of average output power) at λ = 20 µm has been estimated. The source is strongly monochromatic, with a bandwidth of 1% or smaller, highly coherent both transversally and longitudinally, with extreme versatility and tunability.
After the schematic description of the TerRa@BriXSinO THz/FIR source, the paper is devoted to the analysis of the potential use of the Milan facility, including a vision on some possible applications that may come true soon in a programmatic manner. We present different scenarios, ranging from non-linear physics under extreme conditions to polarization sensitive imaging and metamaterial-based sensing, where the TerRa source can be successfully applied.
In a near future, FELs will speed up scientific explorations and open new perspectives in many scientific different fields, from condensed matter to biology and life science, with a need for more and more intense and highly monochromatic beams, and the envisaged facility can have a strong role in fulfilling this demand.
Data Availability Statement
The raw data supporting the conclusions of this article will be made available by the authors, without undue reservation.
Author Contributions
Writing—original draft CK; Writing—review & editing AA and VP. All authors have made a substantial, direct, and intellectual contribution to the work and approved it for publication.
Funding
This work was conducted under the auspices of INFN Italy and supported in part by the project “TERA”. The authors would like to acknowledge funding from the Italian Government, PRIN-TWEET (grant code 2017YCTB59).
Conflict of Interest
The authors declare that the research was conducted in the absence of any commercial or financial relationships that could be construed as a potential conflict of interest.
Publisher’s Note
All claims expressed in this article are solely those of the authors and do not necessarily represent those of their affiliated organizations, or those of the publisher, the editors and the reviewers. Any product that may be evaluated in this article, or claim that may be made by its manufacturer, is not guaranteed or endorsed by the publisher.
References
1. Dhillon SS, Vitiello MS, Linfield EH, Davies AG, Hoffmann MC, Booske J, et al. The 2017 Terahertz Science and Technology Roadmap. J Phys D Appl Phys (2017). doi:10.1088/1361-6463/50/4/043001
2. Neyman PJ, Colson WB, Gottshalk SC, Todd AMM, Blau J, Cohn K. Free Electron Lasers in (2017). in Proceedings of the 38th International Free-Electron Laser Conference, FEL 2017 doi:10.18429/JACoW-FEL2017–MOP066
3. Ramian G. The World Wide Web Virtual Library: Free Electron Laser Research and Applications (2017). Available at: http://sbfel3.ucsb.edu/www/vl_fel.html (Accessed June, 2021).
5. Auston DH, Smith PR. Generation and Detection of Millimeter Waves by Picosecond Photoconductivity. Appl Phys Lett (1983). doi:10.1063/1.94468
6. Castro-Camus E, Alfaro M. Photoconductive Devices for Terahertz Pulsed Spectroscopy: a Review [Invited]. Photon Res (2016).
7. Burford NM, El-Shenawee MO. Review of Terahertz Photoconductive Antenna Technology. Opt Eng (2017). doi:10.1117/1.oe.56.1.010901
8. Bass M, Franken PA, Ward JF, Weinreich G. Optical Rectification. Phys Rev Lett (1962). doi:10.1103/physrevlett.9.446
9. Aoki K, Savolainen J, Havenith M. Broadband Terahertz Pulse Generation by Optical Rectification in GaP Crystals. Appl Phys Lett (2017). doi:10.1063/1.4983371
10. Mehdi I, Siles JV, Lee C, Schlecht E. THz Diode Technology: Status, Prospects, and Applications. Proc IEEE (2017). doi:10.1109/jproc.2017.2650235
11. Maestrini A, Thomas B, Wang H, Jung C, Treuttel J, Jin Y, et al. Schottky Diode-Based Terahertz Frequency Multipliers and Mixers. Comptes Rendus Phys (2010). doi:10.1016/j.crhy.2010.05.002
12. Rana F. Graphene Terahertz Plasmon Oscillators. IEEE Trans Nanotechnol (2008). doi:10.1109/tnano.2007.910334
13. Dobroiu A, Yamashita M, Ohshima YN, Morita Y, Otani C, Kawase K. Terahertz Imaging System Based on a Backward-Wave Oscillator. Appl Opt (2004). doi:10.1364/ao.43.005637
14. Momeni O, Afshari E. High Power Terahertz and Millimeter-Wave Oscillator Design: A Systematic Approach. IEEE J Solid-state Circuits (2011). doi:10.1109/jssc.2011.2104553
15. Sirtori C, Barbieri S, Colombelli R. Wave Engineering with THz Quantum cascade Lasers. Nat Photon (2013). doi:10.1038/nphoton.2013.208
16. Xie Y, Yang N, Duan S, Chu W, Wang Y, Zhao Z, et al. Mid-infrared-pumped Quantum cascade Structure for High-Sensitive Terahertz Detection. Opt Express (2017) 24:15180–8. doi:10.1364/OE.24.015180
17. Chevalier P, Amirzhan A, Wang F, Piccardo M, Johnson SG, Capasso F, et al. Widely Tunable Compact Terahertz Gas Lasers. Science (80- ) (2019). doi:10.1126/science.aay8683
18. Thomson MD, Kreß M, Löffler T, Roskos HG. Broadband THz Emission from Gas Plasmas Induced by Femtosecond Optical Pulses: From Fundamentals to Applications. Laser Photon Rev (2007).
19. Dai J, Liu J, Zhang X-C. Terahertz Wave Air Photonics: Terahertz Wave Generation and Detection with Laser-Induced Gas Plasma. IEEE J Select Top Quan Electron. (2011) 17:183–90. doi:10.1109/jstqe.2010.2047007
20. Buccheri F, Huang P, Zhang XC. Generation and Detection of Pulsed Terahertz Waves in Gas: from Elongated Plasmas to Microplasmas. Front Optoelectron (2018). doi:10.1007/s12200-018-0819-8
21. Withayachumnankul W, Naftaly M. Fundamentals of Measurement in Terahertz Time-Domain Spectroscopy. J Infrared, Millimeter, Terahertz Waves (2014). doi:10.1007/s10762-013-0042-z
22. Park GS, Kim YH, Han H, Han JK, Ahn J, Son JH, et al. Convergence of Terahertz Sciences in Biomedical Systems. Converg Terahertz Sci Biomed Syst (2012) 1–435. doi:10.1007/978-94-007-3965-9
23. Yang X, Zhao X, Yang K, Liu Y, Liu Y, Fu W, et al. Biomedical Applications of Terahertz Spectroscopy and Imaging. Trends Biotechnol (2016) 34:810–24. doi:10.1016/j.tibtech.2016.04.008
24. Wan M, Healy JJ, Sheridan JT. Terahertz Phase Imaging and Biomedical Applications. Opt Laser Tech (2020) 122:105859. doi:10.1016/j.optlastec.2019.105859
25. Jepsen PU, Cooke DG, Koch M. Terahertz Spectroscopy and Imaging - Modern Techniques and Applications. Laser Photon Rev (2011). doi:10.1002/lpor.201000011
26. Wen H, Kim KJ, Zholents A, Byrd J, Cavalleri A. Preface to Special Topic: Intense Terahertz Sources for Time-Resolved Studies of Matter. Rev Sci Instrum (2013). doi:10.1063/1.4790426
27. Tian Y, Liu J, Bai Y, Zhou S, Sun H, Liu W, et al. Undulator for Intense Terahertz Radiation. Nat Photon (2017).
28. Son S, Moon SJ, Park J. Langmuir Wave Undulator for Terahertz Radiation. Opt Lett (2012). doi:10.1364/ol.37.005172
29. Santoro G, Yousef I, Jamme F, Dumas P, Ellis G. Infrared Synchrotron Radiation from Bending Magnet and Edge Radiation Sources for the Study of Orientation and Conformation in Anisotropic Materials. Rev Sci Instrum (2011). doi:10.1063/1.3562900
30. Liao GQ, Li YT, Zhang YH, Liu H, Ge XL, Yang S, et al. Demonstration of Coherent Terahertz Transition Radiation from Relativistic Laser-Solid Interactions. Phys Rev Lett (2016). doi:10.1103/physrevlett.116.205003
31. Tian Q, Du Y, Xu H, Liang Y, Wang Y, Tan Y, et al. Single-shot Spatial-Temporal Electric Field Measurement of Intense Terahertz Pulses from Coherent Transition Radiation. Phys Rev Accel Beams (2020). doi:10.1103/physrevaccelbeams.23.102802
32. Cavalleri A, Wall S, Simpson C, Statz E, Ward DW, Nelson KA, et al. Tracking the Motion of Charges in a Terahertz Light Field by Femtosecond X-ray Diffraction. Nature (2006). doi:10.1038/nature05041
33. Vijayraghavan K, Adams RW, Vizbaras A, Jang M, Grasse C, Boehm G, et al. Terahertz Sources Based on Čerenkov Difference-Frequency Generation in Quantum cascade Lasers. Appl Phys Lett (2012). doi:10.1063/1.4729042
34. Tripathi D, Uma R, Tripathi VK. Excitation of Terahertz Radiation by an Electron Beam in a Dielectric Lined Waveguide with Rippled Dielectric Surface. Phys Plasmas (2012). doi:10.1063/1.4751457
35. Zhang Y, Dong L, Zhou Y. Enhanced Coherent Terahertz Smith-Purcell Superradiation Excited by Two Electron-Beams. Opt Express (2012). doi:10.1364/oe.20.022627
36. Korbly SE, Kesar AS, Sirigiri JR, Temkin RJ. Observation of Frequency-Locked Coherent Terahertz Smith-purcell Radiation. Phys Rev Lett (2005). doi:10.1103/physrevlett.94.054803
37. Tavella F, Stojanovic N, Geloni G, Gensch M. Few-femtosecond Timing at Fourth-Generation X-ray Light Sources. Nat Photon (2011). doi:10.1038/nphoton.2010.311
38. Wu Z, Fisher AS, Goodfellow J, Fuchs M, Daranciang D, Hogan M, et al. Intense Terahertz Pulses from SLAC Electron Beams Using Coherent Transition Radiation. Rev Scientific Instr (2011). doi:10.1063/1.4790427
39. Casalbuoni S, Schmidt B, Schmüser P, Arsov V, Wesch S. Ultrabroadband Terahertz Source and Beamline Based on Coherent Transition Radiation. Phys Rev Spec Top - Accel Beams (2009). doi:10.1103/physrevstab.12.030705
40. Hoffmann MC, Schulz S, Wesch S, Wunderlich S, Cavalleri A, Schmidt B. Coherent Single-Cycle Pulses with MV/cm Field Strengths from a Relativistic Transition Radiation Light Source. Opt Lett (2011). doi:10.1364/ol.36.004473
41. Ding WJ, Li FY, Weng SM, Bai P, Sheng ZM. Coherent Transition Radiation from Relativistic Beam-Foil Interaction in the Terahertz and Optical Range. arXiv (2019).
42. Stojanovic N, Drescher M. Accelerator- and Laser-Based Sources of High-Field Terahertz Pulses. J Phys B: Mol Opt Phys (2013) 46:192001. doi:10.1088/0953-4075/46/19/192001
43. Geloni G, Kocharyan V, Saldin E, Schneidmiller E, Yurkov M. Theory of Edge Radiation. Part I: Foundations and Basic Applications. Nucl Instr Methods Phys Res Sect A Accel Spectrometers, Detect Assoc Equip (2009). doi:10.1016/j.nima.2009.03.240
44. Geloni G, Kocharyan V, Saldin E, Schneidmiller E, Yurkov M. Theory of Edge Radiation. Part II: Advanced Applications. Nucl Instr Methods Phys Res Sect A Accel Spectrometers, Detect Assoc Equip (2009). doi:10.1016/j.nima.2009.04.039
45. Gensch M, Bittner L, Chesnov A, Delsim-Hashemi H, Drescher M, Faatz B, et al. New Infrared Undulator Beamline at FLASH. Infrared Phys Technol (2008). doi:10.1016/j.infrared.2007.12.032
46. Reiche S. 1.3: A Fully 3D Time-dependent FEL Simulation Code. Nucl Instr Methods Phys Res Sect A Accel Spectrometers, Detect Assoc Equip (1999). doi:10.1016/S0168–9002(99)00114-X
48. Mashkovich EA, Shugurov AI, Ozawa S, Estacio E, Tani M, Bakunov MI. Noncollinear Electro-Optic Sampling of Terahertz Waves in a Thick GaAs Crystal. IEEE Trans Terahertz Sci Technol (2015). doi:10.1109/tthz.2015.2461439
49. Cunningham PD, Hayden LM. Optical Properties of DAST in the THz Range. Opt Express (2010). doi:10.1364/oe.18.023620
50. Luo C, Zhao Z, Shi W, Chen Z. An Investigation on Terahertz Response in Electro-Optic Crystals Excited at 1.03 Μm Wavelength. Chin Sci Bull (2014).
51. Wu B, Zhang Z, Cao L, Fu Q, Xiong Y. Electro-optic Sampling of Optical Pulses and Electron Bunches for a Compact THz-FEL Source. Infrared Phys Tech (2018) 92:287–94. doi:10.1016/j.infrared.2018.06.014
52. Wu B, Cao L, Fu Q, Tan P, Xiong Y. Comparison of the Detection Performance of Three Nonlinear Crystals for the Electro-Optic Sampling of a FEL-THZ Source. in IPAC 2014: Proceedings of the 5th International Particle Accelerator Conference (2018).
53. Pradarutti B, Matthäus G, Riehemann S, Notni G, Nolte S, Tünnermann A. Highly Efficient Terahertz Electro-Optic Sampling by Material Optimization at 1060 Nm. Opt Commun (2008). doi:10.1016/j.optcom.2008.06.055
54. Casalbuoni S, Schlarb H, Schmidt B, Schmüser P, Steffen B, Winter A. Numerical Studies on the Electro-Optic Detection of Femtosecond Electron Bunches. Phys Rev Spec Top - Accel Beams (2008). doi:10.1103/physrevstab.11.072802
55. Wu B, Cao L, Xiong YQ, Fu Q, Tan P. Study on Coherent Detection of FEL-THz Source Based on Electro-Optic Sampling. Yuanzineng Kexue Jishu/atomic Energ Sci Technol (2015).
56. Steffen B, Arsov V, Berden G, Gillespie WA, Jamison SP, MacLeod AM, et al. Electro-optic Time Profile Monitors for Femtosecond Electron Bunches at the Soft X-ray Free-Electron Laser FLASH. Phys Rev Spec Top - Accel Beams (2009). doi:10.1103/physrevstab.12.032802
57. Yan X, MacLeod AM, Gillespie WA, Knippels GMH, Oepts D, Van der Meer AFG. Application of Electro-Optic Sampling in FEL Diagnostics. Nucl Instr Methods Phys Res Section A: Acc Spectrometers, Detectors Associated Equipment (1016) 9002(01):01603–5.
58. Frühling U, Wieland M, Gensch M, Gebert T, Schütte B, Krikunova M, et al. Single-shot Terahertz-Field-Driven X-ray Streak Camera. Nat Photon (2009). doi:10.1038/nphoton.2009.160
59. Lu X, Zhang XC. Investigation of Ultra-broadband Terahertz Time-Domain Spectroscopy with Terahertz Wave Gas Photonics. Front Optoelectron (2014). doi:10.1007/s12200-013-0371-5
60. Balakin AV, Borodin AV, Dzhidzhoev MS, Gorgienko VM, Esaulkov MN, Zhvaniya IA, et al. Terahertz Emission during Interaction of Ultrashort Laser Pulses with Gas Cluster Beam. in Journal of Physics: Conference Series (2014). doi:10.1088/1742-6596/735/1/012021
61. Dai J, Liu J, Ho IC, Karpowicz N, Zhang XC. Mechanism and Potential Applications of THz Air Photonics. In Optics InfoBase Conference Papers (2014). doi:10.1364/cleo.2010.cmp1
62. Arik E, Koral C, Altan H, Esentürk O. 2013. A New Method for Alcohol Content Determination of Fuel Oils by Terahertz Spectroscopy. in International Conference on Infrared, Millimeter, and Terahertz Waves, IRMMW-THz. doi:10.1109/IRMMW-THz.2013.6665885
63. Passarelli A, Bartosik H, Rumolo G, Vaccaro VG, Masullo MR, Koral C, et al. Novel Measurement Technique for the Electromagnetic Characterization of Coating Materials in the Sub-THz Frequency Range. Phys Rev Accel Beams (2018) 21:103101. doi:10.1103/PhysRevAccelBeams.21.103101
64. Koral C, Papari G, Silvestri B, İmparato C, Andreone A. THz Time Domain Spectroscopy Investigation into Phase Transition of Hybrid Sol Gel Synthesised TiO2 Nano Powders (2014).
65. Papari G, Koral C, Hallam T, Duesberg G, Andreone A. Terahertz Spectroscopy of Amorphous WSe2 and MoSe2 Thin Films. Materials (2018) 11:1613. doi:10.3390/ma11091613
66. Koral C, Ortaç B, Altan H. Terahertz Time-Domain Study of Silver Nanoparticles Synthesized by Laser Ablation in Organic Liquid. IEEE Trans Terahertz Sci Technol (2016). doi:10.1109/tthz.2016.2572360
67. Heyman JN, Unterrainer K, Craig K, Galdrikian B, Sherwin MS, Campman K, et al. Temperature and Intensity Dependence of Intersubband Relaxation Rates from Photovoltage and Absorption. Phys Rev Lett (1995). doi:10.1103/physrevlett.74.2682
68. Murdin BN. Far-infrared Free-Electron Lasers and Their Applications. Contemp Phys (2009) 50:391–406. doi:10.1080/00107510902733856
69. Zhang TY, Zhao W. Excitonic Optical Absorption in Semiconductors under Intense Terahertz Radiation. Chin Phys B (2008). doi:10.1088/1674–1056/17/11/054
70. Srivastava A, Srivastava R, Wang J, Kono J. Laser-induced Above-Band-gap Transparency in GaAs. Phys Rev Lett (2004) 93:1–4. doi:10.1103/PhysRevLett.93.157401
71. Alymov G, Rumyantsev V, Morozov S, Gavrilenko V, Aleshkin V, Svintsov D. Fundamental Limits to Far-Infrared Lasing in Auger-Suppressed HgCdTe Quantum Wells. ACS Photon (2020). doi:10.1021/acsphotonics.9b01099
72. Ciesla CM, Murdin BN, Phillips TJ, White AM, Beattie AR, Langerak CJGM, et al. Auger Recombination Dynamics of Hg0.795Cd0.205Te in the High Excitation Regime. Appl Phys Lett (1997). doi:10.1063/1.119588
73. Zhang X, Shen JX, Van De Walle CG. Anomalous Auger Recombination in PbSe. Phys Rev Lett (2020). doi:10.1103/physrevlett.125.037401
74. Klimov VI, McGuire JA, Schaller RD, Rupasov VI. Scaling of Multiexciton Lifetimes in Semiconductor Nanocrystals. Phys Rev B - Condens Matter Mater Phys (2008). doi:10.1103/physrevb.77.195324
75. Zabel T, Reuterskiöld Hedlund C, Gustafsson O, Karim A, Berggren J, Wang Q, et al. Auger Recombination in In(Ga)Sb/InAs Quantum Dots. Appl Phys Lett (2015). doi:10.1063/1.4905455
76. Menzel S, Zibik EA, Aivaliotis P, Cockburn JW, Wilson LR, Steer MJ. Evidence for Nonadiabatic Electron-Phonon Intraband Scattering in Self-Assembled Quantum Dots. Phys Rev B - Condens Matter Mater Phys (2008). doi:10.1103/physrevb.77.153302
77. Rauter P, Fromherz T, Bauer G, Vinh NQ, Murdin BN, Phillips JP, et al. Direct Monitoring of the Excited State Population in Biased SiGe Valence Band Quantum wells by Femtosecond Resolved Photocurrent Experiments. Appl Phys Lett (2006). doi:10.1063/1.2397004
78. Gauthier-Lafaye O, Sauvage S, Boucaud P, Julien FH, Glotin F, Prazeres R, et al. Investigation of Mid-infrared Intersubband Stimulated Gain under Optical Pumping in GaAs/AlGaAs Quantum wells. J Appl Phys (1998). doi:10.1063/1.367079
79. Dekorsy T, Yakovlev VA, Seidel W, Helm M, Keilmann F. Infrared-Phonon–Polariton Resonance of the Nonlinear Susceptibility in GaAs. Phys Rev Lett (2003).
80. Yan JY, Liu RB, Zhu BF. Exciton Absorption in Semiconductor Superlattices in a strong Longitudinal THz Field. New J Phys (2009). doi:10.1088/1367-2630/11/8/083004
81. Teich M, Wagner M, Schneider H, Helm M. Semiconductor Quantum Well Excitons in strong, Narrowband Terahertz fields. New J Phys (2013). doi:10.1088/1367-2630/15/6/065007
82. Huttner U, Huber R, Kira M, Koch SW. Strong-field Terahertz Excitations in Semiconductors. Encyclopedia Mod Opt (2018). doi:10.1016/B978-0-12-803581-8.09576-X
83. Houver S, Lebreton A, Mottaghizadeh A, Amanti MI, Sirtori C, Beaudoin G, et al. Multi-Terahertz Sideband Generation on an Optical Telecom Carrier with a Quantum Cascade Laser. ACS Photon (2018). doi:10.1021/acsphotonics.7b01124
84. Maslov AV, Citrin DS. Optical Absorption and Sideband Generation in Quantum wells Driven by a Terahertz Electric Field. Phys Rev B - Condens Matter Mater Phys (2000). doi:10.1103/physrevb.62.16686
85. Kono J, Su MY, Inoshita T, Noda T, Sherwin MS, Allen SJ, et al. Resonant Terahertz Optical Sideband Generation from Confined Magnetoexcitons. Phys Rev Lett (1997). doi:10.1103/physrevlett.79.1758
86. Otteneder M, Kvon ZD, Tkachenko OA, Tkachenko VA, Jaroshevich AS, Rodyakina EE, et al. Giant Terahertz Photoconductance of Quantum Point Contacts in the Tunneling Regime. Phys Rev Appl (2018). doi:10.1103/physrevapplied.10.014015
87. Apostolakis A, Pereira MF. Superlattice Nonlinearities for Gigahertz-Terahertz Generation in Harmonic Multipliers. Nanophotonics (2020). doi:10.1515/nanoph-2020-0155
88. Pereira MF, Anfertev VA, Zubelli JP, Vaks VL. Terahertz Generation by Gigahertz Multiplication in Superlattices. J Nanophotonics (2017). doi:10.1117/1.jnp.11.046022
89. Parkinson P, Joyce HJ, Gao Q, Tan HH, Zhang X, Zou J, et al. Carrier Lifetime and Mobility Enhancement in Nearly Defect-free Core-Shell Nanowires Measured Using Time-Resolved Terahertz Spectroscopy. Nano Lett (2009).
90. Winnerl S, Schomburg E, Brandl S, Kus O, Renk KF, Wanke MC, et al. Frequency Doubling and Tripling of Terahertz Radiation in a GaAs/AlAs Superlattice Due to Frequency Modulation of Bloch Oscillations. Appl Phys Lett (2000). doi:10.1063/1.1290141
91. De Seta M, Capellini G, Ortolani M, Virgilio M, Grosso G, Nicotra G, et al. Narrow Intersubband Transitions in N-type Ge/SiGe Multi-Quantum wells: Control of the Terahertz Absorption Energy Trough the Temperature Dependent Depolarization Shift. Nanotechnology (2012) 23:465708–4484. doi:10.1088/0957-4484/23/46/465708
92. Müller T, Parz W, Strasser G, Unterrainer K. Influence of Carrier-Carrier Interaction on Time-dependent Intersubband Absorption in a Semiconductor Quantum Well. Phys Rev B - Condens Matter Mater Phys (2004). doi:10.1103/physrevb.70.155324
93. Murdin BN, Hollingworth AR, Barker JA, Clarke DG, Findlay PC, Pidgeon CR, et al. Double-resonance Spectroscopy of InAs/GaAs Self-Assembled Quantum Dots. Phys Rev B - Condens Matter Mater Phys (2000). doi:10.1103/physrevb.62.r7755
94. Tarasov GG, Zhuchenko ZY, Lisitsa MP, Mazur YI, Wang ZM, Salamo GJ, et al. Optical Detection of Asymmetric Quantum-Dot Molecules in Double-Layer InAs/GaAs Structures. Semiconductors (2006). doi:10.1134/s1063782606010143
95. Lemaître A, Gérard JM, Thierry-Mieg V. Third-harmonic Generation in Inas/gaas Self-Assembled Quantum Dots. Phys Rev B - Condens Matter Mater Phys (1999).
96. Hu X, Zhang Y, Guzun D, Ware ME, Mazur YI, Lienau C, et al. Photoluminescence of InAs/GaAs Quantum Dots under Direct Two-Photon Excitation. Sci Rep (2020). doi:10.1038/s41598-020-67961-z
97. Tokman M, Bodrov SB, Sergeev YA, Korytin AI, Oladyshkin I, Wang Y, et al. Second Harmonic Generation in Graphene Dressed by a strong Terahertz Field. Phys Rev B (2019). doi:10.1103/physrevb.99.155411
98. Lin S, Yu S, Talbayev D. Measurement of Quadratic Terahertz Optical Nonlinearities Using Second-Harmonic Lock-In Detection. Phys Rev Appl (2018). doi:10.1103/physrevapplied.10.044007
99. Lakhmanskaya O, Simpson M, Murauer S, Nötzold M, Endres E, Kokoouline V, et al. Rotational Spectroscopy of a Triatomic Molecular Anion. Phys Rev Lett (2018). doi:10.1103/physrevlett.120.253003
100. Samyh A, Salhi W, Rajira A, Akabli H, Abounadi A, Almaggoussi A. Double Two-Photon Absorption in an Asymmetric Stepped Quantum Well in the Terahertz Range. Superlattices Microstruct (2019). doi:10.1016/j.spmi.2019.05.032
101. Liu Z, Vaswani C, Luo L, Cheng D, Yang X, Zhao X, et al. Coherent Band-Edge Oscillations and Dynamic LO Phonon Mode Splitting as Evidence for Polaronic Coupling in Perovskites. arXiv (2020).
102. Abreu AVP, Teixeira JF, Fonseca ALDA, Gargano R. E Silva GM, Ribeiro LA. Impact of the Electron-Phonon Interactions on the Polaron Dynamics in Graphene Nanoribbons. J Phys Chem A (2016).
103. Liu XN, Yao DZ, Xu QQ, Zhang RJ, Xiong GG. Saturation of Intraband Absorption in a Self-Assembled Double-Quantum-Dot Molecule. Chem Phys Lett (2012). doi:10.1016/j.cplett.2012.09.046
104. Hafez HA, Chai X, Levesque P, Al-Naib I, Dignam MM, Lassonde P, et al. Carrier Dynamics in Gated Graphene Revealed by Tunable-Infrared-Pump/terahertz-Probe Spectroscopy. In: 2016 Conference on Lasers and Electro-Optics, CLEO (2016). doi:10.1364/cleo_at.2016.jw2a.43
105. Shaker H, Krasilnikov M, Boonpornprasert P, Li X, Georgiev G, Brachmann A, et al. Accelerator-based Tunable THz Source for Pump-And-Probe Experiments at the European X-ray Free-Electron Laser Facility. Int Conf Infrared, Millimeter, Terahertz Waves, Irmmw-thz (2019). doi:10.1109/IRMMW-THz10.1109/irmmw-thz.2019.8874425
106. Strait JH, Wang H, Shivaraman S, Shields V, Spencer M, Rana F. Very Slow Cooling Dynamics of Photoexcited Carriers in Graphene Observed by Optical-Pump Terahertz-Probe Spectroscopy. Nano Lett (2011). doi:10.1021/nl202800h
107. George PA, Strait J, Dawlaty J, Shivaraman S, Chandrashekhar M, Rana F, et al. Ultrafast Optical-Pump Terahertz-Probe Spectroscopy of the Carrier Relaxation and Recombination Dynamics in Epitaxial Graphene. Nano Lett (2008). doi:10.1021/nl8019399
108. Amelin K, Alexanian Y, Nagel U, Roõm T, Robert J, Debray J, et al. Terahertz Magneto-Optical Investigation of Quadrupolar Spin-Lattice Effects in Magnetically Frustrated Tb2 Ti2 O7. Phys Rev B (2020).
109. Jin Z, Tkach A, Casper F, Spetter V, Grimm H, Thomas A, et al. Accessing the Fundamentals of Magnetotransport in Metals with Terahertz Probes. Nat Phys (2015). doi:10.1038/nphys3384
110. Bras F, Sauvage S, Fishman G, Boucaud P, Ortega JM, Gérard JM. Fast Decoherence of Slowly Relaxing Polarons in Semiconductor InAs Quantum Dots. Europhys Lett (2005). doi:10.1209/epl/i2005-10010-x
111. Murdin BN, Litvinenko K, Clarke DG, Pidgeon CR, Murzyn P, Phillips PJ, et al. Spin Relaxation by Transient Monopolar and Bipolar Optical Orientation. Phys Rev Lett (2006). doi:10.1103/physrevlett.96.096603
112. Kurihara T, Hirota K, Qiu H, Phan KTN, Kato K, Isoyama G, et al. Reconfiguration of Magnetic Domain Structures of ErFeO3 by Intense Terahertz Free Electron Laser Pulses. Sci Rep (2020) 10:2–6. doi:10.1038/s41598-020-64147-5
113. Gauthier D, Allaria E, Coreno M, Cudin I, Dacasa H, Danailov MB, et al. Chirped Pulse Amplification in an Extreme-Ultraviolet Free-Electron Laser. Nat Commun (2016). doi:10.1038/ncomms13688
114. Usenko S, Przystawik A, Jakob MA, Lazzarino LL, Brenner G, Toleikis S, et al. Attosecond Interferometry with Self-Amplified Spontaneous Emission of a Free-Electron Laser. Nat Commun (2017). doi:10.1038/ncomms15626
115. De Ninno G, Gauthier D, Mahieu B, Ribič PR, Allaria E, Cinquegrana P, et al. Single-shot Spectro-Temporal Characterization of XUV Pulses from a Seeded Free-Electron Laser. Nat Commun (2015). doi:10.1038/ncomms9075
116. Petrillo V, Anania MP, Artioli M, Bacci A, Bellaveglia M, Chiadroni E, et al. Observation of Time-Domain Modulation of Free-Electron-Laser Pulses by Multipeaked Electron-Energy Spectrum. Phys Rev Lett (2013). doi:10.1103/physrevlett.111.114802
117. Ronsivalle C, Anania MP, Bacci A, Bellaveglia M, Chiadroni E, Cianchi A, et al. Large-bandwidth Two-Color Free-Electron Laser Driven by a Comb-like Electron Beam. New J Phys (2014). doi:10.1088/1367-2630/16/3/033018
118. Marinelli A, Ratner D, Lutman AA, Turner J, Welch J, Decker F-J, et al. High-intensity Double-Pulse X-ray Free-Electron Laser. Nat Commun (2015) 6:1–6. doi:10.1038/ncomms7369
119. Petrillo V, Bacci A, Curatolo C, Ferrario M, Gatti G, Maroli C, et al. Dual Color X Rays from Thomson or Compton Sources. Phys Rev Spec Top - Accel Beams (2014). doi:10.1103/physrevstab.17.020706
120. Drebot I, Petrillo V, Serafini L. Two-colour X-Gamma ray Inverse Compton Back-Scattering Source. EPL (2017). doi:10.1209/0295-5075/120/14002
121. Schulz S, Grguraš I, Behrens C, Bromberger H, Costello JT, Czwalinna MK, et al. Femtosecond All-Optical Synchronization of an X-ray Free-Electron Laser. Nat Commun (2015). doi:10.1038/ncomms6938
122. Ferrari E, Spezzani C, Fortuna F, Delaunay R, Vidal F, Nikolov I, et al. Widely Tunable Two-Colour Seeded Free-Electron Laser Source for Resonant-Pump Resonant-Probe Magnetic Scattering. Nat Commun (2016) 7:1–8. doi:10.1038/ncomms10343
123. Jiang MP, Trigo M, Savic I, Fahy S, Murray D, Bray C, et al. The Origin of Incipient Ferroelectricity in lead telluride. Nat Commun (2016). doi:10.1038/ncomms12291
124. Gerber S, Kim KW, Zhang Y, Zhu D, Plonka N, Yi M, et al. Direct Characterization of Photoinduced Lattice Dynamics in BaFe2As2. Nat Commun (2015). doi:10.1038/ncomms8377
125. Ilchen M, Hartmann G, Gryzlova EV, Achner A, Allaria E, Beckmann A, et al. Symmetry Breakdown of Electron Emission in Extreme Ultraviolet Photoionization of Argon. Nat Commun (2018). doi:10.1038/s41467-018-07152-7
126. Shi C, Zhu J, Xu M, Wu X, Peng Y. An Approach of Spectra Standardization and Qualitative Identification for Biomedical Materials Based on Terahertz Spectroscopy. Sci Program (2020). doi:10.1155/2020/8841565
127. Zhang Y, Wang C, Huai B, Wang S, Zhang Y, Wang D, et al. Continuous-wave Thz Imaging for Biomedical Samples. Appl Sci (2021).
128. Sun Q, He Y, Liu K, Fan S, Parrott EPJ, Pickwell-MacPherson E. Recent Advances in Terahertz Technology for Biomedical Applications. Quant Imaging Med Surg (2017). doi:10.21037/qims.2017.06.02
129. Gong A, Qiu Y, Chen X, Zhao Z, Xia L, Shao Y. Biomedical Applications of Terahertz Technology. Appl Spectrosc Rev (2020). doi:10.1080/05704928.2019.1670202
130. Zhang T, Zakharova M, Vozianova A, Podshivalov A, Fokina M, Nazarov R, et al. Terahertz Optical and Mechanical Properties of the Gelatin-Starch-Glycerol-Bentonite Biopolymers. J Biomed Photon Eng (2020). doi:10.18287/jbpe20.06.020304
131. Ogasa Y, Hayashi S. Label-Free Detection of Biopolymer Using Terahertz Waves. J Spectrosc Soc Jpn (2008). doi:10.5111/bunkou.57.228
132. Xu J, Plaxco KW, Allen SJ, 2006. Terahertz Spectroscopy of Biopolymers in Water: Absorption and Circular Dichroism. in doi:10.1109/icimw.2006.368783
133. Buttersack C. Hydrophobicity of Carbohydrates and Related Hydroxy Compounds. Carbohydr Res (2017). doi:10.1016/j.carres.2017.04.019
134. Heyden M, Bründermann E, Heugen U, Niehues G, Leitner DM, Havenith M. Long-range Influence of Carbohydrates on the Solvation Dynamics of Water - Answers from Terahertz Absorption Measurements and Molecular Modeling Simulations. J Am Chem Soc (2008). doi:10.1021/ja0781083
135. Cui X, Cai W, Shao X. Glucose Induced Variation of Water Structure from Temperature Dependent Near Infrared Spectra. RSC Adv (2016). doi:10.1039/c6ra18912a
136. Yang X, Zhao X, Yang K, Liu Y, Liu Y, Fu W, et al. Biomedical Applications of Terahertz Spectroscopy and Imaging. Trends Biotechnol (2016). doi:10.1016/j.tibtech.2016.04.008
137. Wang P, He MX, Li M, Qu QH, Liu R, Chen Y De. Application of Terahertz Spectroscopy in the Detection of Bioactive Peptides. Guang Pu Xue Yu Guang Pu Fen Xi/Spectroscopy Spectr Anal (2020) doi:10.3964/j.issn.1000-0593(2020)
138. Neu J, Stone EA, Spies JA, Storch G, Hatano AS, Mercado BQ, et al. Terahertz Spectroscopy of Tetrameric Peptides. J Phys Chem Lett (2019). doi:10.1021/acs.jpclett.9b01091
139. Falconer RJ, Markelz AG. Terahertz Spectroscopic Analysis of Peptides and Proteins. J Infrared, Millimeter, Terahertz Waves (2012). doi:10.1007/s10762-012-9915-9
140. Martens J, Grzetic J, Berden G, Oomens J. Structural Identification of Electron Transfer Dissociation Products in Mass Spectrometry Using Infrared Ion Spectroscopy. Nat Commun (2016) 7:1–7. doi:10.1038/ncomms11754
141. Paciaroni A, Comez L, Longo M, Sebastiani F, Bianchi F, Orecchini A, et al. Terahertz Collective Dynamics of DNA as Affected by Hydration and Counterions. J Mol Liq (2020). doi:10.1016/j.molliq.2020.113956
142. Cheon H, Yang HJ, Lee SH, Kim YA, Son JH. Terahertz Molecular Resonance of Cancer DNA. Sci Rep (2016). doi:10.1038/srep37103
143. Cheon H, Paik JH, Choi M, Yang HJ, Son JH. Detection and Manipulation of Methylation in Blood Cancer DNA Using Terahertz Radiation. Sci Rep (2019). doi:10.1038/s41598-019-42855-x
144. Xinda W, Zhengxun S, Yan L, Siyi F, Baihe L, Zuobin W. ReviewofDNA DetectionandApplicationBasedonTHzSpectroscopy. Laser Optoelectron Prog (2020). doi:10.3788/lop57.170003
145. Patil MR, Ganorkar SB, Patil AS, Shirkhedkar AA. Terahertz Spectroscopy: Encoding the Discovery, Instrumentation, and Applications toward Pharmaceutical Prospectives. Crit Rev Anal Chem (2020). doi:10.1080/10408347.2020.1802219
146. Markl D, Ruggiero MT, Zeitler JA. Pharmaceutical Applications of Terahertz Spectroscopy and Imaging. Eur Pharm Rev (2016).
147. Alves-Lima D, Song J, Li X, Portieri A, Shen Y, Zeitler JA, et al. Review of Terahertz Pulsed Imaging for Pharmaceutical Film Coating Analysis. Sensors (Switzerland) (2020). doi:10.3390/s20051441
148. Bawuah P, Markl D, Farrell D, Evans M, Portieri A, Anderson A, et al. Terahertz-Based Porosity Measurement of Pharmaceutical Tablets: a Tutorial. J Infrared, Millimeter, Terahertz Waves (2020). doi:10.1007/s10762-019-00659-0
149. Shen Y-C. Terahertz Pulsed Spectroscopy and Imaging for Pharmaceutical Applications: A Review. Int J Pharmaceutics (2011) 417:48–60. doi:10.1016/j.ijpharm.2011.01.012
150. Lien Nguyen K, Friščić T, Day GM, Gladden LF, Jones W. Terahertz Time-Domain Spectroscopy and the Quantitative Monitoring of Mechanochemical Cocrystal Formation. Nat Mater (2007). doi:10.1038/nmat1848
151. Liang J, Zhang X, Wang N, Chang T, Cui HL. Vibrational Spectra of Pyrazinamide and Isoniazid Studied by Terahertz Spectroscopy and Density Functional Theory. Spectrochim Acta - A Mol Biomol Spectrosc (2020). doi:10.1016/j.saa.2019.117591
152. Dierks TM, Korter TM. Comparison of Intermolecular Forces in Anhydrous Sorbitol and Solvent Cocrystals. J Phys Chem A (2017). doi:10.1021/acs.jpca.7b04313
153. Qin J, Zhu B, Du Y, Han Z. Terahertz Detection of Toxic Gas Using a Photonic crystal Fiber. Opt Fiber Technol (2019). doi:10.1016/j.yofte.2019.101990
154. Sterczewski LA, Przewłoka A, Kaszub W, Sotor J. Computational Doppler-Limited Dual-Comb Spectroscopy with a Free-Running All-Fiber Laser. APL Photon (2019). doi:10.1063/1.5117847
155. Araki M, Tabata Y, Shimizu N, Matsuyama K. Terahertz Spectroscopy of CO and NO: The First Step toward Temperature and Concentration Detection for Combustion Gases in Fire Environments. J Mol Spectrosc (2019). doi:10.1016/j.jms.2019.05.007
156. Kilcullen P, Hartley ID, Jensen ET, Reid M. Terahertz Time Domain Gas-phase Spectroscopy of Carbon Monoxide. J Infrared, Millimeter, Terahertz Waves (2015). doi:10.1007/s10762-014-0139-z
157. Zakharenko O, Motiyenko RA, Margulès L, Huet TR. Terahertz Spectroscopy of Deuterated Formaldehyde Using a Frequency Multiplication Chain. J Mol Spectrosc (2015). doi:10.1016/j.jms.2015.09.005
158. Xia Y, Du Y, Zhang H, Hong Z. The Qualitative and Quantitative Detection of Sodium Formaldehyde Sulfoxylate Content in Brightener by Using THz-TDS Technology. J Chin Cereal Oils Assoc (2015).
159. Heshmat B, Andrews GM, Naranjo-Montoya OA, Castro-Camus E, Ciceri D, Sanchez AR, et al. Terahertz Scattering and Water Absorption for Porosimetry. Opt Express (2017). doi:10.1364/oe.25.027370
160. Zalden P, Song L, Wu X, Huang H, Ahr F, Mücke OD, et al. Molecular Polarizability Anisotropy of Liquid Water Revealed by Terahertz-Induced Transient Orientation. Nat Commun (2018). doi:10.1038/s41467-018-04481-5
161. Yu L, Hao L, Meiqiong T, Jiaoqi H, Wei L, Jinying D, et al. The Medical Application of Terahertz Technology in Non-invasive Detection of Cells and Tissues: Opportunities and Challenges. RSC Adv (2019). doi:10.1039/c8ra10605c
162. Borovkova M, Khodzitsky M, Demchenko P, Cherkasova O, Popov A, Meglinski I. Terahertz Time-Domain Spectroscopy for Non-invasive Assessment of Water Content in Biological Samples. Biomed Opt Express (2018). doi:10.1364/boe.9.002266
163. Zou Y, Liu Q, Yang X, Huang H-C, Li J, Du L-H, et al. Label-free Monitoring of Cell Death Induced by Oxidative Stress in Living Human Cells Using Terahertz ATR Spectroscopy. Biomed Opt Express (2018). doi:10.1364/boe.9.000014
164. Zotov AK, Gavdush AA, Katyba GM, Safonova LP, Chernomyrdin NV, Dolganova IN. In Situ terahertz Monitoring of an Ice ball Formation during Tissue Cryosurgery: a Feasibility Test. J Biomed Opt (2021). doi:10.1117/1.jbo.26.4.043003
165. Mizuno M, Kitahara H, Sasaki K, Tani M, Kojima M, Suzuki Y, et al. Dielectric Property Measurements of Corneal Tissues for Computational Dosimetry of the Eye in Terahertz Band In Vivo and In Vitro. Biomed Opt Express (2021). doi:10.1364/boe.412769
166. Yamaguchi S, Fukushi Y, Kubota O, Itsuji T, Ouchi T, Yamamoto S. Origin and Quantification of Differences between normal and Tumor Tissues Observed by Terahertz Spectroscopy. Phys Med Biol (2016) 61:6808–20. doi:10.1088/0031-9155/61/18/6808
167. El-Shenawee M, Vohra N, Bowman T, Bailey K. Cancer Detection in Excised Breast Tumors Using Terahertz Imaging and Spectroscopy. Biomed Spectrosc Imaging (2019). doi:10.3233/bsi-190187
168. Danciu M, Alexa-Stratulat T, Stefanescu C, Dodi G, Tamba BI, Mihai CT, et al. Terahertz Spectroscopy and Imaging: A Cutting-Edge Method for Diagnosing Digestive Cancers. Materials (Basel) (2019). doi:10.3390/ma12091519
169. Lewis RA. A Review of Terahertz Sources. J Phys D Appl Phys (2014). doi:10.1088/0022-3727/47/37/374001
170. Imparato C, Iervolino G, Fantauzzi M, Koral C, Macyk W, Kobielusz M, et al. Photocatalytic Hydrogen Evolution by Co-catalyst-free TiO2/C Bulk Heterostructures Synthesized under Mild Conditions. RSC Adv (2020) 10:12519–34. doi:10.1039/D0RA01322F
171. Koral C, Fantauzzi M, Imparato C, Papari GP, Silvestri B, Aronne A, et al. Defects in the Amorphous−crystalline Evolution of Gel-Derived TiO2. J Phys Chem C (2020).
172. Rossbach J, Schneider JR, Wurth W. 10 Years of Pioneering X-ray Science at the Free-Electron Laser FLASH at DESY. Phys Rep (2019). doi:10.1016/j.physrep.2019.02.002
173. Meister S, Lindenblatt H, Trost F, Schnorr K, Augustin S, Braune M, et al. Atomic, Molecular and Cluster Science with the Reaction Microscope Endstation at FLASH2. Appl Sci (2020). doi:10.3390/app10082953
174. Schoenlein RW, Boutet S, Minitti MP, Dunne AM. The Linac Coherent Light Source: Recent Developments and Future Plans. Appl Sci (2017). doi:10.3390/app7080850
175. Kawasaki T, Tsukiyama K, Irizawa A. Dissolution of a Fibrous Peptide by Terahertz Free Electron Laser. Sci Rep (2019) 9:1–8. doi:10.1038/s41598-019-47011-z
176. Thompson RJ, Rempe G, Kimble HJ. Observation of normal-mode Splitting for an Atom in an Optical Cavity. Phys Rev Lett (1992). doi:10.1103/physrevlett.68.1132
177. He Q, Badshah F, Alharbi T, Li L, Yang L. Normal-mode Splitting in a Linear and Quadratic Optomechanical System with an Ensemble of Two-Level Atoms. J Opt Soc Am B (2020). doi:10.1364/josab.37.000148
178. Tan Z, Wang L, Liu M, Zhu Y, Wang J, Zhan M. Normal-mode Splitting of Four-Level Atom-Cavity System under Collective strong Coupling. arXiv (2019).
179. Khitrova G, Gibbs HM, Kira M, Koch SW, Scherer A. Vacuum Rabi Splitting in Semiconductors. Nat Phys (2006). doi:10.1038/nphys227
180. Zheng H, Chen Z, Zhu H, Tang Z, Wang Y, Wei H, et al. Dispersion of Exciton-Polariton Based on ZnO/MgZnO Quantum wells at Room Temperature. Chin Phys B (2020). doi:10.1088/1674-1056/ab99b3
181. Wang J, Su R, Xing J, Bao D, Diederichs C, Liu S, et al. Room Temperature Coherently Coupled Exciton-Polaritons in Two-Dimensional Organic-Inorganic Perovskite. ACS Nano (2018). doi:10.1021/acsnano.8b03737
182. Shelton DJ, Brener I, Ginn JC, Sinclair MB, Peters DW, Coffey KR, et al. Strong Coupling between Nanoscale Metamaterials and Phonons. Nano Lett (2011). doi:10.1021/nl200689z
183. Jin X, Cerea A, Messina GC, Rovere A, Piccoli R, De Donato F, et al. Reshaping the Phonon Energy Landscape of Nanocrystals inside a Terahertz Plasmonic Nanocavity. Nat Commun (2018). doi:10.1038/s41467-018-03120-3
184. Lidzey DG, Bradley DDC, Skolnick MS, Virgili T, Walker S, Whittaker DM. Strong Exciton-Photon Coupling in an Organic Semiconductor Microcavity. Nature (1998). doi:10.1038/25692
185. Ballarini D, De Giorgi M, Gambino S, Lerario G, Mazzeo M, Genco A, et al. Polariton-Induced Enhanced Emission from an Organic Dye under the Strong Coupling Regime. Adv Opt Mater (2014). doi:10.1002/adom.201400226
186. Nikolis VC, Mischok A, Siegmund B, Kublitski J, Jia X, Benduhn J, et al. Strong Light-Matter Coupling for Reduced Photon Energy Losses in Organic Photovoltaics. Nat Commun (2019). doi:10.1038/s41467-019-11717-5
187. Fu M, Tamarat P, Trebbia JB, Bodnarchuk MI, Kovalenko MV, Even J, et al. Unraveling Exciton–Phonon Coupling in Individual FAPbI3 Nanocrystals Emitting Near-Infrared Single Photons. Nat Commun (2018).
188. Squibb RJ, Sapunar M, Ponzi A, Richter R, Kivimäki A, Plekan O, et al. Acetylacetone Photodynamics at a Seeded Free-Electron Laser. Nat Commun (2018). doi:10.1038/s41467-017-02478-0
189. Perakis F, Camisasca G, Lane TJ, Späh A, Wikfeldt KT, Sellberg JA, et al. Coherent X-Rays Reveal the Influence of Cage Effects on Ultrafast Water Dynamics. Nat Commun (2018). doi:10.1038/s41467-018-04330-5
190. Levantino M, Schirò G, Lemke HT, Cottone G, Glownia JM, Zhu D, et al. Ultrafast Myoglobin Structural Dynamics Observed with an X-ray Free-Electron Laser. Nat Commun (2015). doi:10.1038/ncomms7772
191. Nogly P, Panneels V, Nelson G, Gati C, Kimura T, Milne C, et al. Lipidic Cubic Phase Injector Is a Viable crystal Delivery System for Time-Resolved Serial Crystallography. Nat Commun (2016). doi:10.1038/ncomms12314
192. Grünbein ML, Bielecki J, Gorel A, Stricker M, Bean R, Cammarata M, et al. Megahertz Data Collection from Protein Microcrystals at an X-ray Free-Electron Laser. Nat Commun (2018). doi:10.1038/s41467-018-05953-4
193. Damari R, Weinberg O, Krotkov D, Demina N, Akulov K, Golombek A, et al. Strong Coupling of Collective Intermolecular Vibrations in Organic Materials at Terahertz Frequencies. Nat Commun (2019). doi:10.1038/s41467-019-11130-y
194. Hertzog M, Wang M, Mony J, Börjesson K. Strong Light-Matter Interactions: A New Direction within Chemistry. Chem Soc Rev (2019) 48:937–61. doi:10.1039/c8cs00193f
195. Sanvitto D, Kéna-Cohen S. The Road towards Polaritonic Devices. Nat Mater (2016). doi:10.1038/nmat4668
196. Kéna-Cohen S, Forrest SR. Room-temperature Polariton Lasing in an Organic Single-crystal Microcavity. Nat Photon (2010). doi:10.1038/nphoton.2010.86
197. Ren J, Liao Q, Huang H, Li Y, Gao T, Ma X, et al. Efficient Bosonic Condensation of Exciton Polaritons in an H-Aggregate Organic Single-Crystal Microcavity. Nano Lett (2020). doi:10.1021/acs.nanolett.0c03009
198. Plumhof JD, Stöferle T, Mai L, Scherf U, Mahrt RF. Room-temperature Bose-Einstein Condensation of Cavity Exciton-Polaritons in a Polymer. Nat Mater (2014). doi:10.1038/nmat3825
199. Daskalakis KS, Maier SA, Murray R, Kéna-Cohen S. Nonlinear Interactions in an Organic Polariton Condensate. Nat Mater (2014). doi:10.1364/cleo_si.2014.stu3o.5
200. Barachati F, Simon J, Getmanenko YA, Barlow S, Marder SR, Kéna-Cohen S. Tunable Third-Harmonic Generation from Polaritons in the Ultrastrong Coupling Regime. ACS Photon (2018). doi:10.1021/acsphotonics.7b00305
201. Grosse E. THz Radiation from Free Electron Lasers and its Potential for Cell and Tissue Studies. Phys Med Biol (2002) 47. doi:10.1088/0031-9155/47/21/312
202. Van Der Schot G, Svenda M, Maia FRNC, Hantke M, Deponte DP, Seibert MM, et al. Imaging Single Cells in a Beam of Live Cyanobacteria with an X-ray Laser. Nat Commun (2015) 6:1–9. doi:10.1038/ncomms6704
204. Schneider M, Günther CM, Pfau B, Capotondi F, Manfredda M, Zangrando M, et al. In Situ single-shot Diffractive Fluence Mapping for X-ray Free-Electron Laser Pulses. Nat Commun (2018). doi:10.1038/s41467-017-02567-0
205. Leshem B, Xu R, Dallal Y, Miao J, Nadler B, Oron D, et al. Direct Single-Shot Phase Retrieval from the Diffraction Pattern of Separated Objects. Nat Commun (2016). doi:10.1038/ncomms10820
206. Pakluea S, Rimjaem S, Saisut J, Thongbai C. Coherent THz Transition Radiation for Polarization Imaging Experiments. Nucl Instr Methods Phys Res Section B: Beam Interactions Mater Atoms (2020) 464:28–31. doi:10.1016/j.nimb.2019.11.027
207. Khatib O, Bechtel HA, Martin MC, Raschke MB, Carr GL. Far Infrared Synchrotron Near-Field Nanoimaging and Nanospectroscopy. ACS Photon (2018) 5:2773–9. doi:10.1021/acsphotonics.8b00565
208. Rubano A, Mou S, Marrucci L, Paparo D. Terahertz Hyper-Raman Time-Domain Spectroscopy. ACS Photon (2019). doi:10.1021/acsphotonics.9b00265
209. Kain V, Velotti FM, Fraser MA, Goddard B, Prieto J, Stoel LS, et al. Resonant Slow Extraction with Constant Optics for Improved Separatrix Control at the Extraction Septum. Phys Rev Accel Beams (2019). doi:10.1103/physrevaccelbeams.22.101001
210. Schewa S, Schroer MA, Zickmantel T, Song Y-H, Blanchet CE, Gruzinov AY, et al. A THz Transparent 3D Printed Microfluidic Cell for Small Angle X-ray Scattering. Rev Scientific Instr (2020) 91:084101. doi:10.1063/5.0004706
211. Soukoulis CM, Wegener M. Past Achievements and Future Challenges in the Development of Three-Dimensional Photonic Metamaterials. Nat Photon (2011). doi:10.1038/nphoton.2011.154
212. Yang X, Yang K, Luo Y, Fu W. Terahertz Spectroscopy for Bacterial Detection: Opportunities and Challenges. Appl Microbiol Biotechnol (2016). doi:10.1007/s00253-016-7569-6
213. Papari GP, Koral C, Andreone A. Sensing Biological Fluids Using Resonating Surface Plasmon Polaritons in the THz Range. In: 2019 13th International Congress on Artificial Materials for Novel Wave Phenomena, Metamaterials (2019). doi:10.1109/MetaMaterials.2019.8900883
Keywords: Terahertz (THz) radiation, infrared - IR, spectroscopy, accelerators, imaging, free electron laser (FEL)
Citation: Koral C, Mazaheri Z, Papari GP, Andreone A, Drebot I, Giove D, Masullo MR, Mettivier G, Opromolla M, Paparo D, Passarelli A, Petrillo V, Piccirillo B, Rubano A, Ruijter M, Russo P and Serafini L (2022) Multi-Pass Free Electron Laser Assisted Spectral and Imaging Applications in the Terahertz/Far-IR Range Using the Future Superconducting Electron Source BriXSinO. Front. Phys. 10:725901. doi: 10.3389/fphy.2022.725901
Received: 16 June 2021; Accepted: 04 February 2022;
Published: 03 March 2022.
Edited by:
Ralf Hendrik Menk, Elettra Sincrotrone Trieste, ItalyReviewed by:
Naoto Yagi, Japan Synchrotron Radiation Research Institute, JapanArya Fallahi, ETH Zurich, Switzerland
Copyright © 2022 Koral, Mazaheri, Papari, Andreone, Drebot, Giove, Masullo, Mettivier, Opromolla, Paparo, Passarelli, Petrillo, Piccirillo, Rubano, Ruijter, Russo and Serafini. This is an open-access article distributed under the terms of the Creative Commons Attribution License (CC BY). The use, distribution or reproduction in other forums is permitted, provided the original author(s) and the copyright owner(s) are credited and that the original publication in this journal is cited, in accordance with accepted academic practice. No use, distribution or reproduction is permitted which does not comply with these terms.
*Correspondence: Antonello Andreone, YW5kcmVvbmVAdW5pbmEuaXQ=