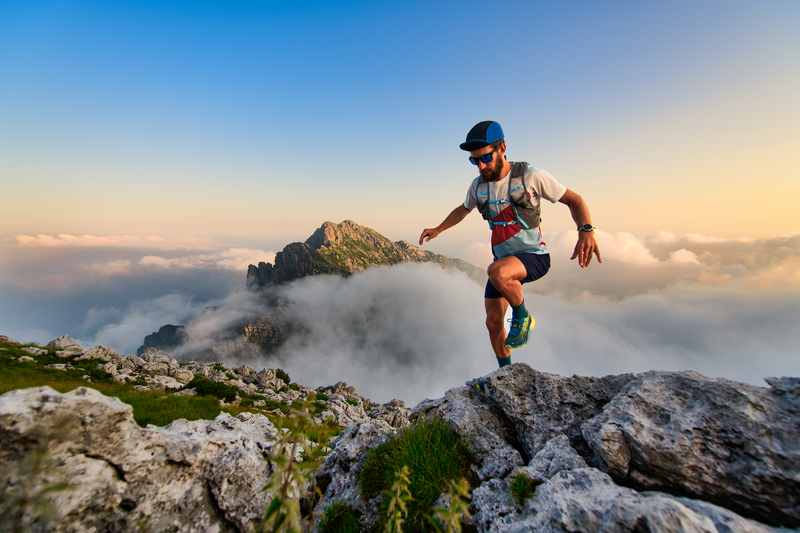
95% of researchers rate our articles as excellent or good
Learn more about the work of our research integrity team to safeguard the quality of each article we publish.
Find out more
BRIEF RESEARCH REPORT article
Front. Phys. , 15 November 2022
Sec. Optics and Photonics
Volume 10 - 2022 | https://doi.org/10.3389/fphy.2022.1043435
This article is part of the Research Topic Miniaturized High-Power Solid-state Laser and Applications View all 23 articles
We demonstrate a 143.4 W high power combined white supercontinuum (SC) source using a (7 × 1) SC fiber combiner. Based on the criteria of adiabatic tapering and brightness conservation, a (7 × 1) SC fiber combiner is designed and theoretically investigated, and the simulated results of the transmission efficiencies of laser at different wavelengths of the combiner verify that the combiner has the ability of combining white SC sources efficiently. Then, the (7 × 1) SC fiber combiner is fabricated, and three white SC fiber laser sources with average power of about 50 W are set up for beam combining experiment. Finally, a 143.4 W high power combined white SC source is achieved with high combining efficiency of 97.4%. and the corresponding combined SC spectrum ranges from 450 nm to 1700 nm, which remains consistent with the overall spectral range of the three SC sources. The experiment result indicates that the (7 × 1) SC fiber combiner is greatly capable of combining high power white SC sources efficiently, thereby increasing the average power of the white SC source significantly. To the best of our knowledge, this is the first demonstration of a fiber combiner for high power white SC sources with such a high combining efficiency.
The development of high power white supercontinuum (SC) fiber laser source which contains a large portion of visible-waveband power, is of particular interest in many areas, such as hyperspectral spectral imaging, astronomical optical frequency combs, and remote illumination, due to its broadband spectrum, high brightness, high coherence, and compact structure [1–4]. In recent years, a lot of high power white SC fiber laser sources have been demonstrated. For example, in 2016, using a 63.3 W ps pulses with a large chirp as pump source, 30.4 W white SC source was obtained with spectrum ranging from 385 nm to 2,400 nm [5]. Based on a high nonlinear photonic crystal fiber (PCF), 53.3 W white SC source with spectrum ranging from 430 nm to 2,400 nm was generated using a 120 W picosecond pulse laser in the same year [6]. In 2018, 80 W white SC source pumped by a 114 W pulse fiber laser was obtained, and the corresponding spectral range was from 370 nm to 2,400 nm [7]. In the same year, a 215 W white SC source spanning from 480 nm to over 2,000 nm was reported with a pulses pump source of 556 W [8]. In 2020, a 104 W white SC source covering from 370 nm to 2,400 nm was generated by pumping cascaded photonic crystal fibers with 375 W ps pulse [9]. It can be seen from these reports that the commonly used method of generating high power white SC source is to couple high power pulses provided by a master oscillator power amplifier (MOPA) pulsed fiber laser into a length of PCF with high nonlinear coefficient through a mode field adapter (MFA) to stimulate high power white SC laser. This method is simple in principle and easy to implement, but there are still two technical difficulties which limit the further increase of white SC average power. The first one is the nonlinear effects during the pulses amplification process in the high power MOPA pulsed fiber laser, such as Stimulated Raman Scattering (SRS). The effects will cause the pump energy to be transferred to other wavelengths, and thus the signal pulses cannot be further linearly amplified to obtain high power output. The gain fiber with large mode field area is usually adopted to solve this problem [5], However, the excessively large mode field area will cause serious mode field mismatch between the pigtail of pulses fiber laser and the PCF, thereby reducing the coupling efficiency of the pulses. In addition, inserting a repetition frequency multiplier (RFM) before the amplifiers of the pulses fiber laser is another way to suppress nonlinear effects [6–9], yet the RFM only works in a limited frequency doubling range. Therefore, the nonlinear effects problem is difficult to completely overcome. The second one is the thermal damage of fibers under high power operation condition of fiber laser. For example, the heat accumulation on gain fibers and PCFs caused by quantum defect and on MFA caused by coupling loss will increase temperature of the fibers, which may cause the fibers to burn and even damage the entire laser. The commonly used method to solve this problem is to place the fibers prone to accumulating heat on the water-cooled heat sink [8, 10, 11], but such a complicated structure is not conducive to the practical application of white SC fiber laser.
For above two difficulties, SC beam combining technology is an ideal solution. It can not only effectively avoid nonlinear effects and fiber thermal damage because the single-channel laser source does not need to pursue high power limit, but also increase the white SC average power significantly. However, commercial fiber combiners can only work at a single wavelength, and so far, there are only a handful reports on the SC fiber combiner. In 2015, using a (3 × 1) broadband fiber combiner, a 202.2 W high power combined near-infrared SC source with spectrum ranging from 1,060 nm to 1900 nm was obtained [12]. Up to now, the fiber combiner for high power white SC sources has not been reported.
In this paper, a 143.4 W high power combined white SC source using a (7 × 1) SC fiber combiner is demonstrated. We design and theoretically investigate a (7 × 1) SC fiber combiner based on the criteria of adiabatic tapering and brightness conservation, and theoretically prove that the designed combiner has the ability to combine white SC sources efficiently by simulating the transmission efficiencies of laser at different wavelengths. Then, a (7 × 1) SC fiber combiner is fabricated, and three white SC fiber laser sources with average power of about 50 W are set up for beam combining experiment. Finally, a 143.4 W high power combined white SC source is achieved with high combining efficiency of 97.4%, and the combined spectrum ranging from 450 nm to 1700 nm remains consistent with the overall spectral range of three SC sources. The experiment result indicates that the (7 × 1) SC fiber combiner is capable of combining high power white SC sources efficiently, and has the capability of increasing the average power of the white SC source significantly. To the best of our knowledge, this is the first demonstration of a fiber combiner for high power white SC sources with such a high combining efficiency. And this research result will contribute to further increasing the white SC power, thereby broadening the application fields of white SC source.
Compared with ordinary fiber combiners working at a single wavelength, the difficulty in designing a SC fiber combiner is to make it work at multiple wavelengths to achieve wide-spectrum beam combination. Therefore, two design criteria need to be followed. They are the brightness conservation [13] and the adiabatic tapering [14].
The brightness conservation formula is
The general method of fabricating a fiber combiner is to insert the input fiber bundle into a silica tube at first, then tapering them, and finally splicing the tapered fiber bundle to the output fiber. The basic structure of the designed (7 × 1) SC fiber combiner is schematically shown in Figure 1A. In the process of tapering the input fiber bundle, as the fiber core diameter decreases gradually, the laser mode will no longer maintain the initial mode field distribution, and will leak into the fiber cladding to cause transmission loss. Therefore, the transmission loss of fiber combiner mainly comes from the tapered region of input fiber bundle. The tapered region structure of a single fiber is shown in Figure 1B, where
FIGURE 1. (A) The structure of the (7 × 1) SC fiber combiner. (B) The schematic of tapered region of a single fiber.
Using the relevant formulas of the criteria of adiabatic tapering in the [14], the relationships between critical taper length and
FIGURE 2. (A) The relationships between the critical taper length and TR at different wavelengths. (B) The transmission efficiencies of the SC combiner at different wavelengths.
Utilizing the specific structural parameters designed, a mathematic model of the (7 × 1) SC fiber combiner is established. Based on the Finite-Difference Beam Propagation Method, the laser transmission efficiencies at different wavelengths of a wide-spectrum source ranging from 400 nm to 1800 nm are simulated, as shown in Figure 2B. It can be seen that the shorter the wavelength is, the higher the transmission efficiency is, which is consistent with the theoretical analysis. And the average transmission efficiency of the wide-spectrum source can reach more than 90%. The simulation result proves that the designed (7 × 1) SC fiber combiner has the ability to combine white SC sources efficiently.
Based on the structure parameters designed above, a (7 × 1) SC fiber combiner is fabricated experimentally, and three white SC fiber laser sources are set up for beam combining experiment. Their average powers are 52 W, 47.1 W and 48.1 W respectively, with the corresponding SC spectra ranging from 480 nm to 1,700 nm, from 470 nm to 1,700 nm, and from 450 nm to 1,700 nm, as shown in Figure 3A, which are measured by optical spectrum analyzers of YOKOGAWA AQ6370C (measuring range from 600 nm to 1,700 nm) and Ocean Optics HR4000 (measuring range from 200 nm to 1,100 nm). The details of the white SC fiber laser source can be found in [6]. The experimental setup is shown in Figure 3B. Three white SC fiber laser sources are connected to the three input fibers of the combiner, and a 1-cm long output end cap with an 8-deg cleaved facet is spliced to the output fiber of the combiner to eliminate back reflection and prevent end facet damage.
FIGURE 3. (A) The spectra of the three white SC sources. (B) The experimental setup of white SC beam combining.
Firstly, the transmission efficiencies of seven channels of the (7 × 1) SC fiber combiner are measured separately with the 52 W white SC fiber laser source. Figure 4A shows the measurement result that the transmission efficiency of each channel is above 97% and the average transmission efficiency of seven channels is up to 98.8%. Meanwhile, from the inset of Figure 4A, it can be seen that output spectrum from each channel is consistent with the input spectrum of 52 W white SC source, which indicates that the SC fiber combiner has consistent transmission efficiency for each wavelength over the overall SC range. The experiment results show that the fabricated SC fiber combiner can transmit the white SC source efficiently and uniformly.
FIGURE 4. (A) The transmission efficiency of each channel of the (7 × 1) SC fiber combiner. Inset: Input spectrum and output spectra of the (7 × 1) SC fiber combiner. (B) The combined SC spectrum, and the photograph of white SC beam combining experimental at 143.4 W combined output power.
Then, three white SC fiber laser sources and three channels selected randomly from the (7 × 1) SC fiber combiner are used to perform the white SC beam combining experiment, with No.3 channel corresponding to 48.1 W white SC source, No.4 channel corresponding to 47.1 W white SC source, and No.7 channel corresponding to 52 W white SC source. Eventually, 143.4 W high power combined white SC source is successfully achieved with high combining efficiency of 97.4%, and the corresponding combined SC spectrum ranging from 450 nm to 1700 nm is consist with the overall spectral range of three white SC sources, as shown in Figure 4B. The experiment result indicates that the fabricated (7 × 1) SC fiber combiner is greatly capable of combining high power white SC sources efficiently, and based on the high combining efficiency, the combiner has the potential to increase the average power of white SC source significantly. Meanwhile, it is worth noting that the current combined power is limited by the number of white SC fiber laser sources, thus more sources will be built in the future for beam combining experiment to obtain higher power white SC source. In addition, the combining efficiency of the SC fiber combiner is slightly lower than the average transmission efficiency of seven channels of the combiner, which may be caused by the increase of the splice point loss between the input fiber bundle and the output fiber under high power operation conditions, thus the splicing quality will be further improved in the next work.
In conclusion, the beam combining technology is an ideal method for obtaining high power white SC source, because it not only can effectively avoid some technical difficulties in the commonly used methods of generating high power white SC source, such as nonlinear effects and fiber thermal damage, but also has the ability of further increasing the white SC power. In this paper, a 143.4 W high power combined white SC source using a SC fiber combiner has been presented. Based on the criteria of adiabatic tapering and brightness conservation, a (7 × 1) SC fiber combiner has been designed and theoretically investigated, and is verified to be capable of combining white SC sources efficiently by simulating the transmission efficiencies of laser at different wavelengths. Then, the (7 × 1) SC fiber combiner is fabricated, and three white SC fiber laser sources with average power of about 50 W are set up for beam combining experiment. Finally, a 143.4 W high power combined white SC source is achieved with high combining efficiency of 97.4%, and the corresponding combined spectrum ranging from 450 nm to 1700 nm is consist with the overall spectral range of three white SC sources The experiment result indicates that the (7 × 1) SC fiber combiner is capable of combining high power white SC sources efficiently, and has the ability of increasing the average power of the white SC source significantly. At present, the combined power is limited by the number of white SC sources, thus more sources will be built in the future for beam combining to obtain higher power white SC source. To the best of our knowledge, this is the first demonstration of a fiber combiner for high power white SC sources with such a high combining efficiency, and the research result will contribute to further increasing the average power of the white SC source, thereby broadening the application fields of white SC source.
The original contributions presented in the study are included in the article/Supplementary Material, further inquiries can be directed to the corresponding author.
CS, TG, and ZW contributed to conception and design of the study. CS performed theoretical simulations. CS and KC conducted the experiment. CS wrote the manuscript. All authors contributed to manuscript revision, read, and approved the submitted version.
The authors declare that the research was conducted in the absence of any commercial or financial relationships that could be construed as a potential conflict of interest.
All claims expressed in this article are solely those of the authors and do not necessarily represent those of their affiliated organizations, or those of the publisher, the editors and the reviewers. Any product that may be evaluated in this article, or claim that may be made by its manufacturer, is not guaranteed or endorsed by the publisher.
1. Dudley JM, Genty G, Coen S. Supercontinuum generation in photonic crystal fiber. Rev Mod Phys (2006) 78(4):1135–84. doi:10.1103/revmodphys.78.1135
2. Martinez-Ramirez D, Buller G, MoCathy A, Ren X, Morak AWS, Nichol C, Woodhouse I. Developing hyperspectral LiDAR for structure and biochemical analysis of forest data. In: Proc. EARSEL conf. Adv. Geosci (2012). p. 1–11.
3. Hartl I, Li XD, Chudoba C, Ghanta RK, Ko TH, Fujimoto JG, et al. Ultrahigh- resolution optical coherence tomography using continuum generation in an air-silica microstructure optical fiber. Opt Lett (2001) 26(9):608–10. doi:10.1364/ol.26.000608
4. Dudley JM, Taylor JR. Ten years of nonlinear optics in photonic crystal fibre. Nat Photon (2009) 3(2):85–90. doi:10.1038/nphoton.2008.285
5. Zou X, Wu J, Wang XD, Qiu JF, Ye Z, Sun C, Ge TW. An all-fiber supercontinuum laser source with high power of 30.4 W and ultra-wide spectrum of 385-2400 nm. In: Proc. CLEO, OSA (2016). Paper SW1Q.4.
6. Sun C, Ge TW, Li SY, An N, Cao K, Wang ZY. 53.3 W visible-waveband extra high power supercontinuum all-fiber laser. IEEE Photon J (2016) 8(6):1–7. doi:10.1109/jphot.2016.2616509
7. Qi X, Chen S, Li Z, Liu T, Ou Y, Wang N, et al. High-power visible-enhanced all-fiber supercontinuum generation in a seven-core photonic crystal fiber pumped at 1016nm. Opt Lett (2018) 43(5):1019–22. doi:10.1364/ol.43.001019
8. Zhao L, Li Y, Guo C, Zhang H, Liu Y, Yang X, et al. Generation of 215 W supercontinuum containing visible spectra from 480 nm. Opt Commun (2018) 425:118–20. doi:10.1016/j.optcom.2018.04.066
9. Zhang H, Li Y, Yan D, Dong K, Lin H, Wang J, et al. All-fiber high power supercontinuum generation by cascaded photonic crystal fibers ranging from 370 nm to 2400 nm. IEEE Photon J (2020) 12:1–8. doi:10.1109/jphot.2020.2983120
10. Sun C, Ge TW, Li SY, An N, Wang ZY. 67.9 W high power white supercontinuum all-fiber laser source. Appl Opt (2016) 55(14):3746–50. doi:10.1364/ao.55.003746
11. Chen H, Wei H, Liu T, Zhou X, Yan P, Chen Z, et al. All-fiber-integrated high-power supercontinuum sources based on multi-core photonic crystal fibers. IEEE J Sel Top Quan Electron (2014) 20(5):64–71. doi:10.1109/jstqe.2014.2304136
12. Zhou H, Jin A, Chen Z, Zhang B, Zhou X, Chen S, et al. Combined supercontinuum source with >200 W power using a 3×1 broadband fiber power combiner. Opt Lett (2015) 40(16):3810–3. doi:10.1364/ol.40.003810
13. Benoit S, Pierre P, Mathieu F. Pump combiner loss as a function of input numerical aperture power distribution. In: Proc. SPIE 7195, The International Society for Optical Engineering (2009). Paper 719523.
Keywords: supercontinuum generation, laser beam combining, fiber lasers, high power, white supercontinuum
Citation: Sun C, Ge T, Cao K and Wang Z (2022) 143.4 W high power combined white supercontinuum source using a (7 × 1) supercontinuum fiber combiner. Front. Phys. 10:1043435. doi: 10.3389/fphy.2022.1043435
Received: 13 September 2022; Accepted: 27 October 2022;
Published: 15 November 2022.
Edited by:
Liyuan Chen, Hilase Center, CzechiaCopyright © 2022 Sun, Ge, Cao and Wang. This is an open-access article distributed under the terms of the Creative Commons Attribution License (CC BY). The use, distribution or reproduction in other forums is permitted, provided the original author(s) and the copyright owner(s) are credited and that the original publication in this journal is cited, in accordance with accepted academic practice. No use, distribution or reproduction is permitted which does not comply with these terms.
*Correspondence: Chang Sun, Y2hhbmdzdW5AYnVhYS5lZHUuY24=
Disclaimer: All claims expressed in this article are solely those of the authors and do not necessarily represent those of their affiliated organizations, or those of the publisher, the editors and the reviewers. Any product that may be evaluated in this article or claim that may be made by its manufacturer is not guaranteed or endorsed by the publisher.
Research integrity at Frontiers
Learn more about the work of our research integrity team to safeguard the quality of each article we publish.