- Jülich Centre for Neutron Science (JCNS), Forschungszentrum Jülich GmbH, Jülich, Germany
Polymer nanocomposites exhibit versatility in their mechanical and structural features predominantly due to the huge surface area provided by nanoparticles. Interaction of the nanoparticles with polymer matrix selectively dictates the applications suitable for a particular polymer nanocomposite system. Novel hybrid polymer-derived materials based on polymer grafted nanoparticles (NPs) can either be mixed with the polymer matrix or self-suspended without matrix polymer. In both cases superior properties are demonstrated compared to the traditional polymer nanocomposites, most notably by 1) incorporation of NPs into polymers without “mixing problems” and 2) a wide range of the transport phenomena (from solids to viscous fluids). Hence, hairy nanoparticle-based nanocomposites are equipped to handle specific and unique challenges in manufacturing and processing methods. It is known that the transport properties can be tuned by altering the molecular design of hairy nanoparticles (i.e., grafted polymer chemistry, NP concentrations, grafting density, and polymer molecular weight) and matrix polymer (e.g., molecular weight). In this article, we review the 1) most common methods of synthesizing hairy nanoparticle, 2) their microscopic dynamics and structural features and 3) some interesting applications of nanocomposite based on hairy nanoparticles. We discuss the effect of various parameters like nanoparticle size, molecular weight of the polymer etc. on the features of nanocomposites and its implications on the properties.
1 Introduction
Polymer nanocomposites derived from polymer grafted nanoparticles guarantee uniform dispersion of nanoparticles and immunity from phase separation [1–4]. In the absence of free polymer, they are known as one component nanocomposites (OCNC). Usage of OCNC have been explored in a variety of applications like gas separation, energy storage etc. due to their unique properties [5–10]. The synthesis chemistry of OCNC can control multiple parameters like the diameter, nanoparticle shape, polymer molecular weight (MW), grafting density etc., giving rise to remarkably tunable properties. Application oriented parameter optimization creates opportunity like probing the origin of properties, which is again a multiparametric problem.
The properties of OCNCs are dependent on the dynamics and viscoelasticity of grafted polymer. Dynamical and viscoelastic features of the polymer are governed by the assembly of nanoparticles and confinement effects. The assembly of nanoparticles is also dependent on the conformational entropy of the grafted chains. Therefore, there exists an interdependence of polymer properties, dynamics and particle assembly [4, 11]. Miscellaneous parts of this problem are discussed in literature by several researchers, many times in relevance of an application. Here, we review some of the crucial research in this area. The goal of this short review is to furnish the reader with sufficient knowledge to understand the critical advances in the field.
2 Synthesis
Synthesis of OCNC is carried out via two approaches: “grafting from” and “grafting to” methods [12–18]. In “grafting from” approach, the monomer is attached to nanoparticle surface and then polymerized, typically by free radical polymerization, such that the polymer grows on the nanoparticle surface [19, 20]. For example, Chevigny et. al. showed the three step polymer grafting process on silica nanoparticles, consisting of silanization of surface with aminopropyltriethoxysilane, followed by grafting of the initiator and polymerization [21]. Similar procedures are used with the other kind of nanoparticles like gold, iron oxide etc., [22–24]. Polymer synthesized from the surface of nanoparticles using reversible addition fragmentation chain transfer polymerization (RAFT) and Atom Transfer Radical Polymerization (ATRP) have been reported widely including the study of polymerization kinetics [25–29]. The advantage of grafting from method is achievement of high grafting densities due to the ease of attaching high number of monomers on surface. However, the grafted polymer suffers from high polydispersity, limiting their suitability for fundamental studies.
In the “grafting to” approach, polymerized chains are attached to the nanoparticles surface [13, 30]. This allows to graft monodisperse polymer on nanoparticle, making these excellent model systems. Polymer chains functionalized with coupling agents like carbonyldiimidazole, disuccinimidyl carbonate etc., are covalently bonded to the silanized particle surface [21, 31]. Due to the bulkiness of chains, the grafting densities are generally lower as compared to the “grafting from” method. However, some newly reported methods claim higher grafting densities even by “grafting to” method. Ligand exchange chemistry produces high grafting density with coordinate bond between the metal nanoparticles and ligand functionalized polymer chains [32, 33]. Shui et. al. reported that using a mixture of good and bad solvent for the polymer, grafting density could be controllably varied [33]. By changing the ratio between solvents, they achieved grafting densities up to 2.8 chains/nm2. In another method, micelles of block copolymers are generated in a solvent followed by the solidification of the micellar core [14, 34]. Different shapes of nanoparticles could be produced such as spheres, plates etc., depending on the ratio of the block lengths and polymer concentrations [35].
We note that there exists a variety of nanoparticle type and polymer combinations e.g., carbon nanotubes grafted with various polymers, polystyrene grafted silica, poly (3-hexylthiophene) grafted ZnO etc., [36–40] However, the discussion for all of these combinations is not the focus of this review. With a brief introduction to the gist of employed synthesis techniques, we now discuss the structure and dynamics of OCNCs.
3 Structure and dynamics
Structure and dynamics of OCNCs have been widely studied using techniques such as static and quasielastic light, small angle X-ray and neutron scattering (SAXS and SANS), microscopy, dielectric spectroscopy, rheology etc. Structural and dynamical features of OCNC exist over multiple length as well as time scales with further complexities at each level.
Some of the intriguing and counterintuitive features are present at length scales from few Angstroms to few nanometers of segmental dynamics i.e., from few picoseconds to few nanoseconds. One could assign the relaxation in this region to the monomer mobilities. Kim et al. used dielectric spectroscopy, rheology and SAXS on polyisoprene grafted silica nanoparticles to show that the segmental dynamics of grafted chains is slower and more coupled than that of the ungrafted chains [41]. They followed the effect of MW and grafting density to conclude that with increase in molecular weight, entanglements and grafting density, the slowing down of dynamics becomes lesser prominent. They attributed their observations to the space filling constraint felt by the grafted chains. On the other hand, Jhalaria et. al. showed using quasielastic neutron scattering (QENS), that for several MW of polymethyl-acrylate grafted silica nanoparticles, the segmental dynamics accelerates as compared to the free polymer [42]. This is attributed to the local increase in the molar volume in nanocomposites. Contrarily, report by Mark et al. showed unchanged segmental dynamics in low MW polyisoprene grafted nanoparticles suggesting MW dependence of the segmental motion in OCNCs [43]. This MW dependence was proven by us for two different MWs of polyisoprene grafted iron-oxide nanoparticles [44]. We analyzed the QENS (backscattering) and dielectric spectroscopy data using relaxation time spectrum analysis and showed a distribution of segmental relaxation times including both faster as well as slower segments for grafted polymer (Figure 1). A difference in the weighted contribution of these appears as the difference in average segmental relaxation times. Mapesa et al. used the distribution of relaxation times extracted from dielectric spectroscopy to study the difference between polymethyl-methacrylate filled with bare silica nanoparticles and corresponding OCNC, for various nanoparticles loadings [45]. The OCNC showed wider distribution of relaxation times especially extended to faster times. They also attributed the increase in segmental dynamics to the increase in molar volume due to increasing interphase between the nanoparticle and polymer. This generates higher packing frustration in OCNC interphase and hence local density fluctuation. The location dependent density of grafted and ungrafted polymers was studied by Power et. al using MD simulations [46]. They found maximum density of polymer segments near the nanoparticle surface, especially for grafted polymers, which gradually decreases to bulk values toward the chain ends. This is in line with the speculations based on QENS [44]. Therefore, the role of interface is crucial in deciding the OCNC properties.
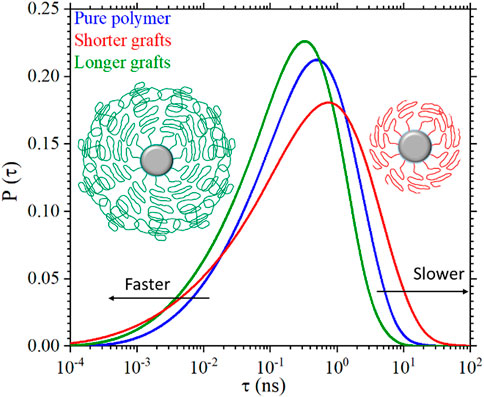
FIGURE 1. Representative distribution of relaxation times for shorter grafted chains and longer grafted chains as compared to the neat polymer.
Dukes et. al. discussed the conformational changes of the polymers grafted on spherical nanoparticles obtained by dynamic light scattering (DLS) [47]. At low grafting density, negligible interactions with the adjacent grafts are present. A mushroom like conformation is assumed with brush height (h) proportional to the radius of gyration (Rg) of the polymer i.e., h ∝ 2Rg. At moderate grafting densities, the neighboring grafts start to interpenetrate therefore, known as semidilute polymer brushes (SDPB). In this regime, h ∝ Nσ1/3lo5/3, where N is degree of polymerization, σ is the grafting density and lo is the monomer length. Polymer chains with much higher grafting densities are known as concentrated polymer brushes (CPB) where, h ∝ Nσ1/3lo2. Simulations have confirmed the transition from mushroom to brush like polymer conformation with increasing grafting density [10]. However, this transition was not observed for small nanoparticles. Wei et. al. performed SANS and neutron spin echo (NSE) experiments on polymethyl-acrylate grafted silica nanoparticles in solvent with different parts of polymer labelled with hydrogen and deuterium in different samples [48]. They showed that in OCNC, the interfacial region near the particle surface consists of stretched chain segments, hence representing the CPB region. However, the segments away from the particle are SDPB. The impact on the dynamics of different segments is also studied. NSE data showed that the segments near the core are slower than those away from the particle due to strong confinement.
The presence of an interfacial layer exhibiting substantial differences than the bulk polymer has been well reported [42–44, 49]. Holt et. al. compared the dynamics of interfacial layer for the poly (2-vinylpyridine) (P2VP) with different MW in OCNC with the physically adsorbed polymer nanoparticles using SAXS, differential scanning calorimetry and dielectric spectroscopy [50]. For low polymer MW, they found negligible difference in the decelerated dynamics of two nanocomposites. Interfacial model calculations show that the volume of interface includes the entire polymer. Similar dynamics is also observed for the high MW. However, for intermediate MW, the grafted polymer shows slower and more heterogenous dynamics as compared to the composites with ungrafted polymers. Using self-consistent field theory calculations, they showed that these trends could be related to the chain stretching in the interfacial region giving rise to slower dynamics, which is prominent in OCNC at intermediate MW. In a different publication, the same group reported that contrary to segmental motion, the β relaxation in P2VP, which corresponds to rotational relaxation of pyridine side groups, becomes faster in composites than the pure polymer [51]. The fastest dynamics exhibited by the OCNCs is counterintuitively accompanied by an increase in glass transition temperature (Tg) and decrease in segmental mobility. This is despite the fact that OCNC have largest free volume available leading to decrease in polymer density. They conclude that while the interfacial chain stretching leads to slower segmental dynamics, the secondary relaxation is controlled by density changes. This also reflects in weaker mechanical properties of OCNC as compared to the pure polymer and polymer adsorbed nanocomposites. Other than the relaxation time scales, the amplitudes of the relaxation are also affected by the presence of interfacial layer [52].
Miller et. al performed core-modified dissipative particle dynamics simulations on OCNC to analyze the effect of grafting on polymer conformation and relaxation [53]. They found an increase in the Rg of polymers after grafting. The confinement effects induced by grafting were characterized by confinement parameter ξ* = 1/Rg0
At length scales on the order of 100 nm to microns, glass like behavior of the OCNC is observed [1, 55, 56]. Wen et. al. using rheology and time concentration superposition showed that the grafted nanoparticles exhibit soft glass like characteristics where the particles perform in-cage rattling like motion [55]. At longer times, they observed the escape of grafted particles from the cage leading to fluid like response [3, 57–59]. These jamming-unjamming transitions are also observed at time scales on the order of 100 s using X-ray photon correlation spectroscopy (XPCS) [60]. The toughness of nanocomposites is related to this glassy response. Liu et. al. showed that the cage strength increases with equilibration of the nanocomposites systems whereas, decreases with increase in temperature [61]. Using SAXS they showed two types of interaction between the nanoparticles: 1. Repulsive interactions between the grafted particles and 2. Entropic attractions between the particle cores created by the grafted polymer.
Nanocomposites have tendency to assemble in different structural forms, from string to well-developed lattices [16, 62–70]. Other than the regular lattice packing of grafted nanoparticles, it is shown by Akcora et. al. that polymer grafted spherical nanoparticles also exhibit tendency to assemble into anisotropic structures when dispersed in free polymer [71]. This self-assembly is governed by the aggregation forces between the nanoparticle core, competing with the entropy of grafted chains [72]. The self-assembly of polymer grafted nanoparticles in solution has been studied by Bachhar et. al. using theoretical model based on the Daoud and Cotton theory for star polymers [73, 74]. They looked at the effect of polydispersity in the nanoparticle core size and showed that the distribution in the core size controls the self-assembly, overriding the effect of grafted chain dispersity. This led to a conclusion that in order to control the assembly of polymer grafted nanoparticles, tuning the nanoparticle size distribution must be the focus of synthetic efforts.
3 Properties
OCNCs exhibit significantly enhanced properties like viscoelasticity, crystallization, gas transport etc. than polymers or nanoparticle reinforced ungrafted polymer. We discuss some of these extensively reported properties here.
3.1 Viscoelasticity
OCNC in general exhibit higher viscosity and moduli as compared to the nanoparticle dispersed polymer or neat polymer in the low frequency range [1, 59, 75–78] Hattemer and Arya used course grain MD simulations to probe the origin of higher moduli in OCNC as compared to the particle dispersed nanocomposites [79]. They showed that the existence of grafts introduces additional distortion of the shear field in polymer leading to higher modulus. The low frequency higher modulus is also affected by the slower relaxation of the grafted chains. The higher moduli are captured using a phenomenological model based on the Rouse formulation. Sakib et. al. conducted rheology experiments along with DSC to show the shift of viscoelastic response in OCNC towards lower frequencies as compared to the neat polymer [57]. This is attributed to an increase in Tg due to the space filling constraint of grafted polymer similar to that reported by Kim et. al. [41, 80]. Whereas, the plateau modulus of grafted chains, which is related to the entanglements decreases for lower MW grafts and increases for high MW grafts. The explanation of this effect has been given as a decrease in the effective entanglement density in shorter chain due to lesser penetration of the nanoparticle core into the polymer of neighboring particles. For larger chains, the nanoparticle core also participates in entanglement, generating higher entanglement density. Chen et. al. investigated the viscoelastic properties of OCNC in terms of the variation in composite toughness with polymer MW [60]. The toughness of polymethylacrylate grafted SiO2 nanoparticles exhibits a peak in molecular weights in Laser-induced projectile impact testing. Combination of entanglement and particle jamming controls this behavior [58, 61]. XPCS and QENS are used to relate the toughness of OCNC to the segmental relaxations and the colloidal flow.
3.2 Polymer crystallization
Where presence of nanoparticle provides a nucleation site for the polymer, the existence of grafting impedes the formation of folded chain crystals [81, 82]. Kim et. al. showed that the polymer grafted on nanoparticles crystallizes in contrasting manners at different length scales. While at nanometer scale helical structures are abundantly present, at length scales greater than the nanoparticle size, the overall crystallinity is lower than the pure polymer, indicating restricted formation of crystalline domains [83]. They claim that the polymer chains crystallize into extended chain crystals due to crowding and therefore, the spherulites are not formed in the polymer brush on particle surface. However, Zhao et. al. reported the formation of spherulites even in case of densely grafted nanoparticles [84]. They advocated the formation of extended chain crystals for OCNC with high grafting densities. Employing Avrami analysis, they showed that with increase in the grafting density, the crystallization kinetics becomes faster. They attribute this to the ease of nucleation provided by the presence of nanoparticle surface. Wen et. al. found that for shorter grafted chains of methoxypolyethylene glycol, the confinement effects are stronger than longer chains [85]. For low grafting densities, the crystallinity and crystallization temperature decrease indicating resistance in crystallization. They obtained an Avrami index = 1 for low grafting density OCNC suggesting confined crystallization due to nucleation barrier. This effect is observed because of the enhanced interaction of grafted polymer with nanoparticle surface at low grafting densities.
3.3 Other transport properties
Polymer nanocomposites show suitable transport properties for various applications [86]. A series of papers have been published on significant enhancement in CO2 vs. CH4 separation for polymethacrylate grafted nanoparticles as compared to neat polymer membranes [5, 42, 87–90]. It is shown that by varying the MW and grafting density of the polymer, selectivity and gas permeability can be tuned. The gas diffusivity increases with increasing MW till a maximum and then decreases. Detailed study of the OCNC membranes is carried out using QENS, SAXS, MD simulations and theoretical models. It is shown that the increase in local free volume (decreased density) of the grafted polymer as compared to the neat polymer gives way for the enhanced gas transport. Midya et. al. presented a two phase model for the grafted polymer chains around the nanoparticles [88]. They showed that there exist two regions of polymer segments; near the particle surface segments are stretched (known as dry layer) and do not interpenetrate with the segments of adjacent particles whereas, away from the surface interpenetration among the polymer segments from different particles takes place (Figure 2). The stretching leads to a decrease in the local friction and hence faster segmental mobility [90]. This creates pathways for the gas transport.
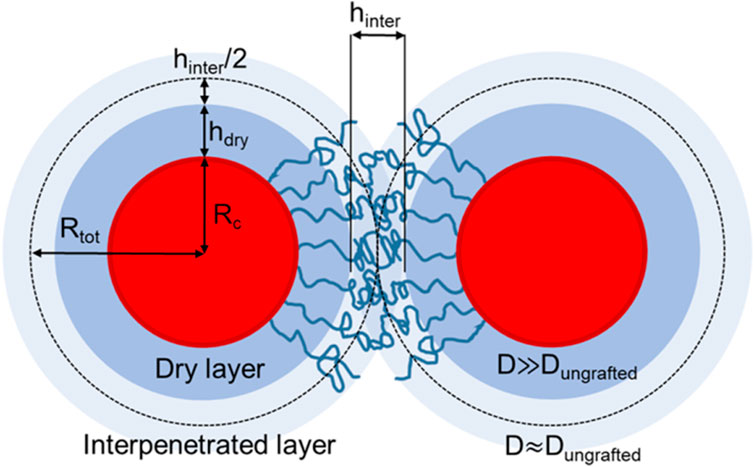
FIGURE 2. Schematic for the two-phase model of polymer grafted nanoparticle showing dry zone and interpenetrated zone. Reprinted with permission from Bilchak CR, Jhalaria M, Adhikari S, Midya J, Huang Y, Abbas Z, Nikoubashman A, Benicewicz BC, Rubinstein M, Kumar SK. Understanding Gas Transport in Polymer-Grafted Nanoparticle Assemblies. Macromolecules (2022) 55:3011–3019. [89] Copyright 2022 American Chemical Society.
OCNC also show outstanding ion transport in energy storage devices therefore, becoming potential candidates for the solid-state electrolytes [6]. These findings are supported by the tunable mechanical and ion conducting properties of OCNC leading to enhanced device stability. Grabowski et. al. showed that for supercapacitors, while the dispersion and dielectric breakdown remains similar in blended nanoparticle-polymer system and OCNC [91], the charge/discharge efficiency and the energy storage capacity of the hairy nanoparticle systems is substantial greater than the conventional nanocomposites.
Polymer grafted nanocomposite with or without free polymer or solvent present plenty of opportunities in various applications. However, the precise origin of the properties of these materials is still an emerging field. While the fundamental knowledge of OCNC physics is important for optimization and tunability of properties, it is also crucial for understanding the complex behavior of polymers and their derivatives.
Author contributions
This article is jointly written by AS and MK based on the literature in the field.
Conflict of interest
The authors declare that the research was conducted in the absence of any commercial or financial relationships that could be construed as a potential conflict of interest.
Publisher’s note
All claims expressed in this article are solely those of the authors and do not necessarily represent those of their affiliated organizations, or those of the publisher, the editors and the reviewers. Any product that may be evaluated in this article, or claim that may be made by its manufacturer, is not guaranteed or endorsed by the publisher.
References
1. Vlassopoulos D, Cloitre M. Tunable rheology of dense soft deformable colloids. Curr Opin Colloid Interf Sci (2014) 19:561–74. doi:10.1016/j.cocis.2014.09.007
2. Bailey EJ, Winey KI. Dynamics of polymer segments, polymer chains, and nanoparticles in polymer nanocomposite melts: A review. Prog Polym Sci (2020) 105:101242. doi:10.1016/j.progpolymsci.2020.101242
3. Kumar SK, Krishnamoorti R. Nanocomposites: Structure, phase behavior, and properties. Annu Rev Chem Biomol Eng (2010) 1:37–58. doi:10.1146/annurev-chembioeng-073009-100856
4. Kalb J, Dukes D, Kumar SK, Hoy S, Grest GS. End grafted polymernanoparticles in a polymeric matrix: Effect of coverage and curvature. Soft Matter (2011) 7:1418–25. doi:10.1039/c0sm00725k
5. Bilchak CR, Jhalaria M, Huang Y, Abbas Z, Midya J, Benedetti FM, et al. Tuning selectivities in gas separation membranes based on polymer-grafted nanoparticles. ACS Nano (2020) 14:17174–83. doi:10.1021/acsnano.0c07049
6. Choudhury S, Stalin S, Deng Y, Archer LA. Soft colloidal glasses as solid-state electrolytes. Chem Mater (2018) 30:5996–6004. doi:10.1021/acs.chemmater.8b02227
7. Bockstaller MR. Progress in polymer hybrid materials. Prog Polym Sci (2015) 40:1–2. doi:10.1016/j.progpolymsci.2014.11.001
8. Sarkar B, Alexandridis P. Block copolymer-nanoparticle composites: Structure, functional properties, and processing. Prog Polym Sci (2015) 40:33–62. doi:10.1016/j.progpolymsci.2014.10.009
9. Deng C, Wu J, Cheng R, Meng F, Klok H, Zhong Z. Functional polypeptide and hybrid materials: Precision synthesis via α-amino acid N-carboxyanhydride polymerization and emerging biomedical applications. Prog Polym Sci (2014) 39:330–64. doi:10.1016/j.progpolymsci.2013.10.008
10. Lin J, Zhang H, Morovati V, Dargazany R. PEGylation on mixed monolayer gold nanoparticles: Effect of grafting density, chain length, and surface curvature. J Colloid Interf Sci (2017) 504:325–33. doi:10.1016/j.jcis.2017.05.046
11. Martin TB, Dodd PM, Jayaraman A. Polydispersity for tuning the potential of mean force between polymer grafted nanoparticles in a polymer matrix. Phys Rev Lett (2013) 110:1–5. doi:10.1103/PhysRevLett.110.018301
12. Auroy P, Auvray L, Léger L. Silica particles stabilized by long grafted polymer chains. J Colloid Interf Sci (1992) 150:187–94. doi:10.1016/0021-9797(92)90279-U
13. Hübner E, Allgaier J, Meyer M, Stellbrink J, Pyckhout-Hintzen W, Richter D. Synthesis of polymer/silica hybrid nanoparticles using anionic polymerization techniques. Macromolecules (2010) 43:856–67. doi:10.1021/ma902213p
14. Hoheisel TN, Hur K, Wiesner UB. Block copolymer-nanoparticle hybrid self-assembly. Prog Polym Sci (2015) 40:3–32. doi:10.1016/j.progpolymsci.2014.10.002
15. Fischer S, Salcher A, Kornowski A, Weller H, Förster S. Completely miscible nanocomposites. Angew Chem Int Ed (2011) 50:7811–4. doi:10.1002/anie.201006746
16. Fernandes NJ, Koerner H, Giannelis EP, Vaia RA. Hairy nanoparticle assemblies as one-component functional polymer nanocomposites: Opportunities and challenges. MRS Commun (2013) 3:13–29. doi:10.1557/mrc.2013.9
17. Hou Z, Liu Y, Xu J, Zhu J. Surface engineering of magnetic iron oxide nanoparticles by polymer grafting: Synthesis progress and biomedical applications. Nanoscale (2020) 12:14957–75. doi:10.1039/d0nr03346d
18. Bonnevide M, Phan TNT, Malicki N, Kumar SK, Couty M, Gigmes D, et al. Synthesis of polyisoprene, polybutadiene and Styrene Butadiene Rubber grafted silica nanoparticles by nitroxide-mediated polymerization. Polymer (Guildf) (2020) 190:122190. doi:10.1016/j.polymer.2020.122190
19. Carrot G, Harrak AE, Oberdisse J, Jestin J, Bou? F. Polymer grafting from 10-nm individual particles: Proving control by neutron scattering. Soft Matter (2006) 2:1043–7. doi:10.1039/b600749j
20. Laruelle G, Parvole J, Francois J, Billon L. Block copolymer grafted-silica particles: A core/double shell hybrid inorganic/organic material. Polymer (2004) 45:5013–20. doi:10.1016/j.polymer.2004.05.030
21. Chevigny C, Gigmes D, Bertin D, Jestin J, Boué F. Polystyrene grafting from silica nanoparticles via nitroxide-mediated polymerization (NMP): Synthesis and SANS analysis with the contrast variation method. Soft Matter (2009) 5:3741–53. doi:10.1039/B906754J
22. Mandal TK, Fleming MS, Walt DR. Preparation of polymer coated gold nanoparticles by surface-confined living radical polymerization at ambient temperature. Nano Lett (2002) 2:3–7. doi:10.1021/nl015582c
23. Wang Y, Teng X, Wang JS, Yang H. Solvent-free atom transfer radical polymerization in the synthesis of Fe2O3@Polystyrene Core−Shell nanoparticles. Nano Lett (2003) 3:789–93. doi:10.1021/nl034211o
24. Vestal CR, Zhang ZJ. Atom transfer radical polymerization synthesis and magnetic characterization of MnFe2O4/polystyrene core/shell nanoparticles. J Am Chem Soc (2002) 124:14312–3. doi:10.1021/ja0274709
25. Li C, Han J, Ryu CY, Benicewicz BC. A versatile method to prepare RAFT agent anchored substrates and the preparation of PMMA grafted nanoparticles. Macromolecules (2006) 39:3175–83. doi:10.1021/ma051983t
26. Li C, Benicewicz BC. Synthesis of well-defined polymer brushes grafted onto silica nanoparticles via surface reversible Addition−Fragmentation chain transfer polymerization. Macromolecules (2005) 38:5929–36. doi:10.1021/ma050216r
27. Huang X, Wirth MJ. Surface-initiated radical polymerization on porous silica. Anal Chem (1997) 69:4577–80. doi:10.1021/ac9704523
28. Matyjaszewski K, Miller PJ, Shukla N, Immaraporn B, Gelman A, Luokala BB, et al. Polymers at interfaces: Using atom transfer radical polymerization in the controlled growth of homopolymers and block copolymers from silicon surfaces in the absence of untethered sacrificial initiator. Macromolecules (1999) 32:8716–24. doi:10.1021/ma991146p
29. Tsujii Y, Ejaz M, Sato K, Goto A, Fukuda T. Mechanism and kinetics of RAFT-mediated graft polymerization of styrene on a solid surface. 1. Experimental evidence of surface radical migration. Macromolecules (2001) 34:8872–8. doi:10.1021/ma010733j
30. Choudhury S, Agrawal A, Kim SA, Archer LA. Self-suspended suspensions of covalently grafted hairy nanoparticles. Langmuir (2015) 31:3222–31. doi:10.1021/la5048326
32. Ehlert S, Taheri SM, Pirner D, Drechsler M, Schmidt HW, Förster S. Polymer ligand exchange to control stabilization and compatibilization of nanocrystals. ACS Nano (2014) 8:6114–22. doi:10.1021/nn5014512
33. Shui Y, Su Y, Kuang X, Zhao W, Cai Y, Wang D. Facile and controllable synthesis of hybrid silica nanoparticles densely grafted with poly(ethylene glycol). Polym Int (2017) 66:1395–401. doi:10.1002/pi.5391
34. Cao X, Mao W, Mai Y, Han L, Che S. formation of diverse ordered structures in ABC triblock terpolymer templated macroporous silicas. Macromolecules (2018) 51:4381–96. doi:10.1021/acs.macromol.8b00242
35. Ruan Y, Gao L, Yao D, Zhang K, Zhang B, Chen Y, et al. Polymer-grafted nanoparticles with precisely controlled structures. ACS Macro Lett (2015) 4:1067–71. doi:10.1021/acsmacrolett.5b00408
36. Basheer BV, George JJ, Siengchin S, Parameswaranpillai J. Polymer grafted carbon nanotubes-synthesis, properties, and applications: A review. Nano-Structures & Nano-Objects (2020) 22:100429. doi:10.1016/j.nanoso.2020.100429
37. Zhang H, Lei X, Su Z, Liu P. A novel method of surface-initiate atom transfer radical polymerization of styrene from silica nanoparticles for preparation of monodispersed core-shell hybrid nanospheres. J Polym Res (2007) 14:253–60. doi:10.1007/s10965-007-9104-z
38. Awada RC, Medlej H, Blanc H, Delville S, Hiorns A, Bousquet C, et al. Versatile functional poly(3-hexylthiophene) for hybrid particles synthesis by the grafting onto technique: Core@shell ZnO nanorods. J Polym Sci (2014) 52:30–8. doi:10.1002/pola.26964
39. Keller CB, Walley SE, Jarand CW, He J, Ejaz M, Savin DA, et al. Synthesis of poly(caprolactone)-block-poly[oligo(ethylene glycol)methyl methacrylate] amphiphilic grafted nanoparticles (AGNs) as improved oil dispersants. Polym Chem (2021) 12:4758–69. doi:10.1039/d1py00418b
40. Bentz KC, Savin DA. Chain dispersity effects on brush properties of surface-grafted polycaprolactone-modified silica nanoparticles: Unique scaling behavior in the concentrated polymer brush regime. Macromolecules (2017) 50:5565–73. doi:10.1021/acs.macromol.7b00608
41. Kim SA, Mangal R, Archer LA. Relaxation dynamics of nanoparticle-tethered polymer chains. Macromolecules (2015) 48:6280–93. doi:10.1021/acs.macromol.5b00791
42. Jhalaria M, Buenning E, Huang Y, Tyagi M, Zorn R, Zamponi M, et al. Accelerated local dynamics in matrix-free polymer grafted nanoparticles. Phys Rev Lett (2019) 123. doi:10.1103/PhysRevLett.123.158003
43. Mark C, Holderer O, Allgaier J, Hübner E, Pyckhout-Hintzen W, Zamponi M, et al. Polymer chain conformation and dynamical confinement in a model one-component nanocomposite. Phys Rev Lett (2017) 119:1–5. doi:10.1103/PhysRevLett.119.047801
44. Sharma A, Kruteva M, Zamponi M, Ehlert S, Richter D, Förster S. Influence of molecular weight on the distribution of segmental relaxation in polymer grafted nanoparticles. Phys Rev Mater (2022) 6:1–6. doi:10.1103/physrevmaterials.6.l012601
45. Mapesa EU, Street DP, Heres MF, Kilbey SM, Sangoro J. Wetting and chain packing across interfacial zones affect distribution of relaxations in polymer and polymer-grafted nanocomposites. Macromolecules (2020) 53:5315–25. doi:10.1021/acs.macromol.0c00399
46. Power AJ, Remediakis IN, Harmandaris V. Interface and interphase in polymer nanocomposites with bare and core-shell gold nanoparticles. Polymers (2021) 13:541–28. doi:10.3390/polym13040541
47. Dukes D, Li Y, Lewis S, Benicewicz B, Schadler L, Kumar SK. Conformational transitions of spherical polymer brushes: Synthesis, characterization, and theory. Macromolecules (2010) 43:1564–70. doi:10.1021/ma901228t
48. Wei Y, Xu Y, Faraone A, Hore MJA. Local structure and relaxation dynamics in the brush of polymer-grafted silica nanoparticles. ACS Macro Lett (2018) 7:699–704. doi:10.1021/acsmacrolett.8b00223
49. Ndoro TVM, Voyiatzis E, Ghanbari A, Theodorou DN, Böhm MC, Müller-Plathe F. Interface of grafted and ungrafted silica nanoparticles with a polystyrene matrix: Atomistic molecular dynamics simulations. Macromolecules (2011) 44:2316–27. doi:10.1021/ma102833u
50. Holt AP, Bocharova V, Cheng S, Kisliuk AM, White BT, Saito T, et al. Controlling interfacial dynamics: Covalent bonding versus physical adsorption in polymer nanocomposites. ACS Nano (2016) 10:6843–52. doi:10.1021/acsnano.6b02501
51. Holt AP, Bocharova V, Cheng S, Kisliuk AM, Ehlers G, Mamontov E, et al. Interplay between local dynamics and mechanical reinforcement in glassy polymer nanocomposites. Phys Rev Mater (2017) 1:1–6. doi:10.1103/PhysRevMaterials.1.062601
52. Popov I, Carroll B, Bocharova V, Genix A, Cheng S, Khamzin A, et al. Strong reduction in amplitude of the interfacial segmental dynamics in polymer nanocomposites. Macromolecules (2020) 53:4126–35. doi:10.1021/acs.macromol.0c00496
53. Miller CA, Hore MJA. Simulation of the coronal dynamics of polymer-grafted nanoparticles. ACS Polym Au (2021) 2:157–68. doi:10.1021/acspolymersau.1c00031
54. Agarwal P, Kim SA, Archer LA. Crowded, confined, and frustrated: Dynamics of molecules tethered to nanoparticles. Phys Rev Lett (2012) 109:1–4. doi:10.1103/PhysRevLett.109.258301
55. Wen YH, Schaefer JL, Archer LA. Dynamics and rheology of soft colloidal glasses. ACS Macro Lett (2015) 4:119–23. doi:10.1021/mz5006662
56. Parisi D, Ruiz-Franco J, Ruan Y, Liu CY, Loppinet B, Zaccarelli E, et al. Static and dynamic properties of block copolymer based grafted nanoparticles across the non-ergodicity transition. Phys Fluids (2020) 32:127101. doi:10.1063/5.0031862
57. Sakib N, Koh YP, Huang Y, Mongcopa KIS, Le AN, Benicewicz BC, et al. Thermal and rheological analysis of polystyrene-grafted silica nanocomposites. Macromolecules (2020) 53:2123–35. doi:10.1021/acs.macromol.9b02127
58. Parisi D, Buenning E, Kalafatakis N, Gury L, Benicewicz BC, Gauthier M, et al. Universal polymeric-to-colloidal transition in melts of hairy nanoparticles. ACS Nano (2021) 15:16697–708. doi:10.1021/acsnano.1c06672
59. Zawodzinski TA, Alissa Park AH, Sangoro J, Mapesa EU, Cantillo NM, Hamilton ST, et al. Localized and collective dynamics in liquid-like polyethylenimine- based nanoparticle organic hybrid materials. Macromolecules (2021). doi:10.1021/acs.macromol.0c02370
60. Chen SH, Souna AJ, Stranick SJ, Jhalaria M, Kumar SK, Soles CL, et al. Controlling toughness of polymer-grafted nanoparticle composites for impact mitigation. Soft Matter (2022) 18:256–61. doi:10.1039/d1sm01432c
61. Liu X, Abel BA, Zhao Q, Li S, Choudhury S, Zheng J, et al. Microscopic origins of caging and equilibration of self-suspended hairy nanoparticles. Macromolecules (2019) 52:8187–96. doi:10.1021/acs.macromol.9b01473
62. Jiao Y, Akcora P. Understanding the role of grafted polystyrene chain conformation in assembly of magnetic nanoparticles. Phys Rev E (2014) 90:1–9. doi:10.1103/PhysRevE.90.042601
63. Mehdizadeh Taheri SM, Fischer S, Förster S. Routes to nanoparticle-polymer superlattices. Polymers (2011) 3:662–73. doi:10.3390/polym3020662
64. Taheri SM, Fischer S, Trebbin M, With S, Schröder JH, Perlich J, et al. Lyotropic phase behavior of polymer-coated iron oxide nanoparticles. Soft Matter (2012) 8:12124–31. doi:10.1039/c2sm26777b
65. Che J, Park K, Grabowski CA, Jawaid A, Kelley J, Koerner H, et al. Preparation of ordered monolayers of polymer grafted nanoparticles: Impact of architecture, concentration, and substrate surface energy. Macromolecules (2016) 49:1834–47. doi:10.1021/acs.macromol.5b02722
66. Patra TK, Singh JK. Coarse-grain molecular dynamics simulations of nanoparticle-polymer melt: Dispersion vs. agglomeration. J Chem Phys (2013) 138:144901. doi:10.1063/1.4799265
67. Jayaraman A. Polymer grafted nanoparticles: Effect of chemical and physical heterogeneity in polymer grafts on particle assembly and dispersion. J Polym Sci B Polym Phys (2013) 51:524–34. doi:10.1002/polb.23260
68. Meng D, Kumar SK, D. Lane D, Grest GS. Effective interactions between grafted nanoparticles in a polymer matrix. Soft Matter (2012) 8:5002–10. doi:10.1039/c2sm07395a
69. Hore MJA. Polymers on nanoparticles: Structure & dynamics. Soft Matter (2019) 15:1120–34. doi:10.1039/c8sm02110d
70. La T, Kumar K, Panagiotopoulos AZ. Self-assembly of polymer-grafted nanoparticles in thin film. Soft Matter (2014) 10:786–94. doi:10.1039/c3sm52328d
71. Akcora P, Liu H, Kumar SK, Moll J, Li Y, Benicewicz BC, et al. Anisotropic self-assembly of spherical polymer-grafted nanoparticles. Nat Mater (2009) 8:354–9. doi:10.1038/nmat2404
72. Kim JU, Matsen MW. Interaction between polymer-grafted particles. Macromolecules (2008) 41:4435–43. doi:10.1021/ma8002856
73. Bachhar N, Kumaraswamy G, Kumar SK. Core-size dispersity dominates the self-assembly of polymer-grafted nanoparticles in solution. Macromolecules (2019) 52:4888–94. doi:10.1021/acs.macromol.9b00704
74. Daoud M, Cotton JP. Star shaped polymers : A model for the conformation and its concentration dependence. J Phys France (1982) 43:531–8. doi:10.1051/jphys:01982004303053100
75. Voudouris P, Choi J, Gomopoulos N, Sainidou R, Dong H, Matyjaszewski K, et al. Anisotropic elasticity of quasi-one-component polymer nanocomposites. ACS Nano (2011) 5:5746–54. doi:10.1021/nn201431w
76. Goyal S, Escobedo FA. Structure and transport properties of polymer grafted nanoparticles. J Chem Phys (2011) 135:184902. doi:10.1063/1.3657831
77. Peng W, Ranganathan R, Keblinski P, Akcora P, Ozisik R. Viscoelastic and dynamic properties of polymer grafted nanocomposites with high glass transition temperature graft chains. J Appl Phys (2019) 126:195102. doi:10.1063/1.5119694
78. Giovino M, Buenning E, Jimenez A, Kumar SK, Schadler L. Polymer grafted nanoparticle viscosity modifiers. Macromol Chem Phys (2019) 220:1800543. doi:10.1002/macp.201800543
79. Hattemer GD, Arya G. Viscoelastic properties of polymer-grafted nanoparticle composites from molecular dynamics simulations. Macromolecules (2015) 48:1240–55. doi:10.1021/ma502086c
80. Kim D, Srivastava S, Narayanan S, Archer LA. Polymer nanocomposites: Polymer and particle dynamics. Soft Matter (2012) 8:10813–8. doi:10.1039/c2sm26325d
81. Khan J, Harton SE, Akcora P, Benicewicz BC, Kumar SK. Polymer crystallization in nanocomposites: Spatial reorganization of nanoparticles. Macromolecules (2009) 42:5741–4. doi:10.1021/ma900794t
82. Wen X, Su Y, Liu G, Li S, Müller AJ, Kumar SK, et al. Direct relationship between dispersion and crystallization behavior in poly(ethylene oxide)/Poly(ethylene glycol)-g-Silica nanocomposites. Macromolecules (2021) 54:1870–80. doi:10.1021/acs.macromol.0c02259
83. Kim SA, Archer LA. Hierarchical structure in semicrystalline polymers tethered to nanospheres. Macromolecules (2014) 47:687–94. doi:10.1021/ma4019922
84. Zhao W, Su Y, Wen X, Wang D. Manipulating crystallization behavior of poly(ethylene oxide) by functionalized nanoparticle inclusion. Polymer (2019) 165:28–38. doi:10.1016/j.polymer.2019.01.019
85. Wen X, Su Y, Shui Y, Zhao W, Müller AJ, Wang D. Correlation between grafting density and confined crystallization behavior of poly(ethylene glycol) grafted to silica. Macromolecules (2019) 52:1505–16. doi:10.1021/acs.macromol.8b02007
86. Jhalaria M, Cang Y, Huang Y, Benicewicz B, Kumar SK, Fytas G. Unusual high-frequency mechanical properties of polymer-grafted nanoparticle melts. Phys Rev Lett (2022) 128:187801. doi:10.1103/PhysRevLett.128.187801
87. Bilchak CR, Buenning E, Asai M, Zhang K, Durning CJ, Kumar SK, et al. Polymer-grafted nanoparticle membranes with controllable free volume. Macromolecules (2017) 50:7111–20. doi:10.1021/acs.macromol.7b01428
88. Midya J, Rubinstein M, Kumar SK, Nikoubashman A. Structure of polymer-grafted nanoparticle melts. ACS Nano (2020) 14:15505–16. doi:10.1021/acsnano.0c06134
89. Bilchak CR, Jhalaria M, Adhikari S, Midya J, Huang Y, Abbas Z, et al. Understanding gas transport in polymer-grafted nanoparticle assemblies. Macromolecules (2022) 55:3011–9. doi:10.1021/acs.macromol.1c02367
90. Jhalaria M, Huang Y, Ruzicka E, Tyagi M, Zorn R, Zamponi M, et al. Activated transport in polymer grafted nanoparticle melts. Macromolecules (2021) 54:6968–74. doi:10.1021/acs.macromol.1c00601
Keywords: nanocomposites, synthesis, dynamics, structure, properties
Citation: Sharma A and Kruteva M (2022) Towards the polymer nanocomposites based on hairy nanoparticles. Front. Phys. 10:1041903. doi: 10.3389/fphy.2022.1041903
Received: 11 September 2022; Accepted: 28 November 2022;
Published: 06 December 2022.
Edited by:
Marco Laurati, University of Florence, ItalyReviewed by:
Anastassia Rissanou, Foundation for Research and Technology Hellas (FORTH), GreeceAlan Ranjit Jacob, Indian Institute of Technology Hyderabad, India
Copyright © 2022 Sharma and Kruteva. This is an open-access article distributed under the terms of the Creative Commons Attribution License (CC BY). The use, distribution or reproduction in other forums is permitted, provided the original author(s) and the copyright owner(s) are credited and that the original publication in this journal is cited, in accordance with accepted academic practice. No use, distribution or reproduction is permitted which does not comply with these terms.
*Correspondence: Aakash Sharma, a.sharma@fz-juelich.de; Margarita Kruteva, m.kruteva@fz-juelich.de