- 1Radiopharmaceutical Research Centre, Horia Hulubei National Institute for R&D in Physics and Nuclear Engineering, Magurele, Romania
- 2Extreme Light Infrastructure—Nuclear Physics, Horia Hulubei National Institute for R&D in Physics and Nuclear Engineering, Magurele, Romania
- 3University of Bucharest, Doctoral School of Physics, Faculty of Physics, Bucharest, Romania
The use of radioisotopes in nuclear medicine applications became essential for the diagnosis and follow-up imaging of many oncological, cardiovascular, and neurodegenerative diseases, in a safe and non-invasive way. Their use in the personalized treatment of tumors is on the verge to change the oncological patient management. Optimization of the radioisotopes production aims to maximize the production efficiency while minimizing side reactions and costs. A practical approach to balance these non-converging ways is to employ the simulation tools in the process design phase and experimental setup. In this way, the production yield can be estimated and the radionuclide impurities content that appears during the bombardment of the target of interest can be optimally set below acceptable limits. Copper-64 is an emerging radionuclide in nuclear medicine theragnostic applications due to three decay modes, namely electron capture, electron (β−) and positron (β+) emissions, and a 12.7 h half-life, favorable for visualization of fast biological processes. Optimization of 64Cu production by irradiation of enriched 64Ni targets with protons in a particular geometry on a TR-19 cyclotron is discussed in this work. The simulated activity produced on different levels of enrichment of the 64Ni targets was calculated using the Monte Carlo simulation in the Geant4 platform, where a customized solid target irradiation system set-up was replicated; the obtained parameters were implemented in the experiments and the results were compared, aiming to validate the simulation parameters through experimental data.As the simulated and experimental results regarding the production of 64Cu via 64Ni(p,n)64Cu reaction are in good compliance, the tool can be further applied for the optimization of the production of other radionuclides on the same set-up.
Introduction
In recent years, a growing interest in the use of radiopharmaceuticals labeled with metal radionuclides has been shown, copper being one of them, as five of its 27 radioisotopes gained notably importance for molecular imaging applications (60Cu, 61Cu, 62Cu, and 64Cu) and targeted therapy (64Cu and 67Cu), respectively [1].
Paramount versatility of 64Cu devolves from branches of β+ decay (0.653 MeV; 17.85%), ß− decay (0.579 MeV; 38.48%), and electron capture (1.675 MeV, 43.53%) [2], being also an Auger electrons emitter (Auger KLL, 6.26–6.57 keV, 100%) [3, 4]. Its relatively long half-life of 12.7 h, compared with fluorine-18 (T1/2 = 110 min) and carbon-11 (T1/2 = 20.4 min) makes it particularly suitable for simultaneous treatment and monitoring by PET imaging. The production of copper radionuclides, using different cyclotrons, target supports, and enriched starting materials has been studied and the results are reported in the relevant literature [5–7]. The demand for 64Cu increased as it was proved that its production with high yield and high specific activities using typical “medical” cyclotrons (proton energy ranging from 11 to 18 MeV) was feasible in routine conditions [8].
Among the investigated nuclear reactions, 64Ni(p,n)64Cu is the most widely employed, due to the high production yield for the 64Cu at low-energy protons [9, 10]. This production route bears the advantage of fewer side reactions inducing radionuclide impurities into the final product [11–13]. Considering the disadvantage of this process consisting of expensive enriched 64Ni targets, it is essential to optimize production by maximizing the yield. Geant4 is a software platform for the simulation of the passage of particles through matter, based on Monte Carlo methods [14]. It is used on the large scale in various areas of physics, and also in specific medical applications. The simulation tool allows for testing of the radioisotope production parameters, aiming at finding the optimal irradiation parameters.
Geant4 simulation of 64Cu radioisotope production in a solid target
We used Geant4 for simulations of the nuclear reactions for 64Cu production via a solid target irradiation system, installed on the extension of the proton beamline of a TR-19 cyclotron (Advanced Cyclotron Systems Inc., Canada). The outcome was implemented at the Radiopharmaceutical Research Centre, for the production of 64Cu, followed by radiochemical processing.
The code was used for simulations of irradiation of the solid target with accelerated protons, as it can model complex geometries, taking the advantage of greater flexibility in the irradiation parameters selection, and also the output data. Any type of target material can be used in the simulation, as it relies on an internal Geant4 database for half-lives, cross-section evaluation, and nuclear data.
While predicting the yield for the production of a specific isotope of interest and the expected activity, simulations also allow predicting the nuclidic impurities generated during irradiation. The building and validation of the Monte Carlo simulation platform using Geant4 were initially programmed to model a solid target system of the GE Healthcare PETtrace cyclotron from the South Australian Health and Medical Research Institute (SAHMRI) [15]. The nuclear model implemented in this simulation code is based on direct nuclear reaction mechanism. Subsequently, it was included as part of Geant4 advanced examples. The yield and activity values were calculated according to the formulas used in the validated simulation code [16]. The final value of the specific activity is given in mCi·μA−1·h−1, calculated by dividing the activity by the current of the proton beam and the time of irradiation. In the simulations, the values of σi (E) were taken from the ENDF database. The graphical user interface of Geant4 allows the selection and modifications of simulation parameters, such as the energy, shape, and current of the proton beam, the target geometry and material, the foil geometry and material, and the irradiation time.
In this work, the simulation was modified to match the particular geometry of the solid target irradiation station, mounted on a 26ᵒ slope down extension proton beamline of a TR-19 cyclotron, at 160 cm from the exit point. The closing of the vacuum is made withf pure Al foil, with a thickness of 320 µm (acting also a degrader). The cooling of the target is done with He and water. The TR-19 cyclotron is installed at Radiopharmaceutical Research Centre (CCR), from Horia Hulubei National Institute for R&D in Physics and Nuclear Engineering (IFIN-HH), Magurele. The energy of the extracted protons can be set in the 14–19 MeV range, with a 0.1 MeV energy step. The transfer of the target to/from the cyclotron irradiation station is done pneumatically through a flexible tube, which connects the electrodeposition and dissolution module, to the irradiation station.
The solid target irradiation station is made of a tube surrounded by aluminum, a degrader foil made of aluminum, the volume of helium between the degrader foil and the target, used for cooling the front of the target, and the 64Ni target electrodeposited on a baseplate of platinum. The geometry of the solid target irradiation station is presented in Figure 1. The proton beam enters from the left, and is transported through the tube and the degrader before impacting the target.
The collimator is located right before the Aluminum foil (Figure 2) resulting in a negligible angular spread. We also calculated the energetic spread taking into account: the emerging cyclotron bandwidth (broadening = 0.3 MeV) and the straggling induced by the Aluminum foil. The final value of the energetic spread is 0.633 MeV.
Simulation results
According to the 64Ni(p,n)64Cu excitation function, the highest yield of this nuclear reaction is expected at 11.66 MeV incident proton beam energy, as it is shown in Figure 3, with good efficacy in the 6–13 MeV range, thus avoiding the opening of unwanted channels, resulting in isotopes from competitive nuclear reactions [17]. The cross-section values from R. Adam (2009) were used in our simulations.
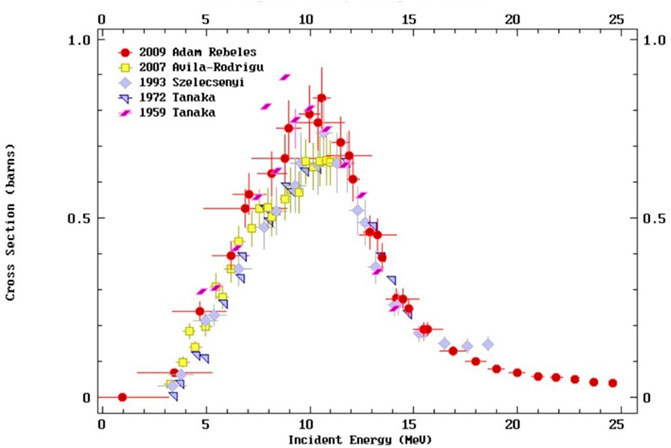
FIGURE 3. Dependence of the cross-sections of 64Ni(p,n)64Cu nuclear reaction by the energy of the incident protons.
TR-19 cyclotron accelerates H− ions, and can extract proton beam at variable energy, in the range of 14–19 MeV, with a maximum current intensity of 300 μA; the intensity of the proton current is limited to a maximum of 40 µA by the design of the solid target irradiation system and by the cooling system. The role of aluminum foil is to degrade the energy of the incident beam to achieve the proper energy window when reaching the target, as required by the above-mentioned nuclear reaction.
Taking into account these aspects, several simulations were performed varying the thickness of the degrader (aluminum foil): no foil, 200, 320, and 400 μm were considered, respectively. In Figure 4, the 64Cu activity at the end of bombardment (EOB) is represented as a function of energy, depending on the thickness of the aluminum foil degrader, at constant irradiation parameters 4 h and 20 µA (80 µAh). The simulation results showed that the optimal thickness of the aluminum foil is 320 μm, for which the estimated activity increased from 177 mCi (2.2 mCi/µAh) without Al foil to about 277 mCi (3.5 mCi/µAh).
Figure 5 presents the energy loss of the primary particles when beam is reaching the 320 μm Al foil (a), at the exit from the Al foil (b), at the target surface (c) and at the exit from the target (target dimensions: 600 μm thickness and 6 mm diameter) (d).
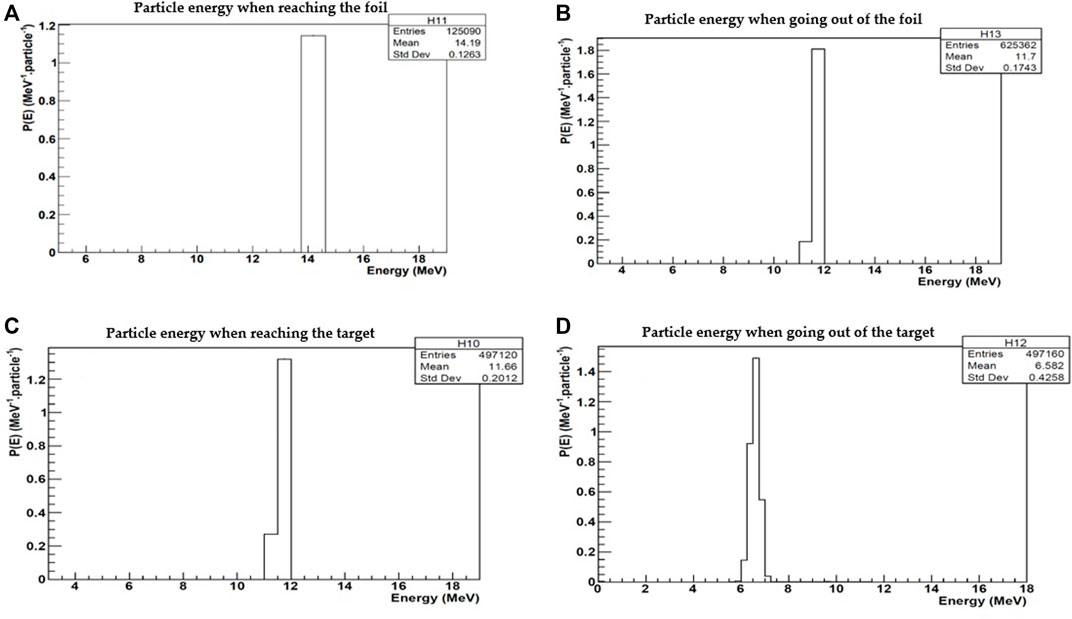
FIGURE 5. The energy loss corresponding to the primary particles when beam is reaching the Al foil (320 μm) (A), at the exit of the Al foil (B), on the target surface (C) and at the exit from the target material (600 μm thickness and 6 mm diameter) on the target baseplate (D).
As result of these simulations, the proton beam energy on target is degraded from 14.2 ± 0.3 MeV to 11.66 ± 0.633 MeV. The simulation input parameters referred also to different levels of enrichment of the 64Ni targets, as presented in Table 1, as the price difference between 99.53% and 95% is significant.
On 99.53% 64Ni enriched target, the maximum activity at EOB for 64Cu radioisotope resulted from simulation was around 277 mCi (3.5 mCi/µAh), for the following input parameters: current intensity 20 μA, irradiation time 4 h and the thickness of Al foil 320 μm. In the case of 95% 64Ni enriched target, the maximum activity at EOB for 64Cu was around 255 mCi (3.2 mCi/µAh), with the same input parameters.
Figure 6 presents the comparison between the 64Cu activity at EOB by using different enrichment levels of target material (64Ni).
The ratio between the two enrichment levels of the 64Ni targets is 1.048, while the ratio of the activities obtained in the simulations is 1.080, with a relative positive difference of 3%.
In Figure 7 can be observed that after a sufficiently long time of irradiation, around 6–7 times half-lives of the 64Cu, the saturation is reached, meaning that the number of decaying 64Cu nuclei is the same as the number of produced 64Cu nuclei.
The results plotted in Figures 4, 6, 7 contain statistical errors (multiple simulations were carried out, under the same conditions, and then the uncertainty was determined). When irradiating 64Ni enriched target with protons, various radioisotopes can be produced by side nuclear reaction on the metallic impurities contained in the target material. Therefore, some radionuclidic impurities are expected to be found in the final product. Table 2 shows the main radioisotope and possible contaminants that are produced during the bombardment. This simulation was conducted for bombardment with 11.66 MeV protons on 99.53% 64Ni enriched target, whose composition is presented in Table 1.
Experimental production of 64Cu by using 64Ni enriched target at TR-19 cyclotron
Materials and experimental methods
The experimental work was performed at Radiopharmaceutical Research Centre [18]. 64Cu was produced via nuclear reaction 64Ni(p,n)64Cu, using the TR-19 cyclotron and Alceo 2.0 automated solid target system (Comecer, Italy) [19–21].
The automated solid target system employed in the process includes modules for electro-deposition (EDS), pneumatic transfer system (PTS), and purification (Taddeo-PRF). The process started with the electroplating of an enriched 64Ni target (99.53%) and continued with its irradiation using a proton beam extracted at 14 MeV. The producing yield is dependent on irradiation parameters such as energy, current intensity, irradiation time, and geometry.
The process involves the electroplating of enriched 64Ni (99.53%) on the platinum baseplate attached to a dedicated shuttle, pneumatically transferred to the irradiation station. Electrodeposition and dissolution of the target were carried out on the EDS module. The extraction and purification of 64Cu from the resulting solution are based on ion exchange chromatography and were carried out on the Taddeo-PRF automated purification module. At the end of the process, 64Cu was delivered in the form of [64Cu]CuCl2 solution.
The target material, isotopically enriched 64Ni, whose composition is presented in Table 1, was purchased from Isoflex Company (San Francisco, CA, United States).
Trace grade reagents were purchased as follows: nitric acid and ammonium chloride from Honeywell (Muskegon, MI, United States); hydrochloric acid and aqueous ammonia from Sigma-Aldrich (Steinheim, Germany). Trace grade water, used for the preparation of solutions and cleaning of the target was purchased from Honeywell (Muskegon, MI, United States). AG1-X8 ion exchange resin was purchased from Bio-Rad Laboratories (Hercules, CA, United States). Acetonitrile for high-performance liquid chromatography (HPLC) was purchased from Merck KGaA, (Darmstadt, Germany).
The radionuclidic purity of [64Cu]CuCl2 solution was assessed using an HPGe detector (Baltic Scientific Instruments (Riga, Latvia) with 25% relative efficiency; the amplified output of the detector was processed by a 4,096 channels multi-channel analyzer (MCA) system. The fitting program InterWinner 7.0 was used for spectral data processing.
Target preparation and irradiation
The 64Ni solution used for electroplating was prepared by dissolution of about 50 mg of highly enriched 64Ni (99.53%) in HNO3 (60%), followed by the pH adjustment with buffer solution (ammonium acetate/ammonium hydroxide). The target is obtained after 15–20 h of electroplating, when the color of the solution turned from cobalt blue to colorless, indicating that the process is finalized. At the end of electroplating, 47.4 mg of the metallic target was deposited on the center of the platinum baseplate, in a shape of 6 mm diameter with approx. 600 µm thickness. These values correspond to an apparent density of 2.79 g/cm3 (which is 3.19-fold lower than the density of the solid 64Ni). Hence, for the most accurate results, this value of apparent density was declared as the input parameter for simulation.
The irradiation of the 64Ni target was performed with a 14 MeV proton energy degraded to 11.66 MeV by the use of an aluminum foil of 320 μm in the beam line, placed in front of the target. The target was irradiated for 4 h at a 20 μA beam current.
The simulation results were verified in terms of obtained activity by experimental work. The parameters of the experimental work and simulation are presented in Table 3.
For the experimental value of the final activity of the target, the value of uncertainty can be attributed due to process loss of activity, mainly in the purification step, the uncertainty on the cross-section values used, geometry and unevenly distribution of the mass in the real target.
A good correlation of the simulation results with the experimental data was observed, the estimated activity in the given conditions, of 277 ± 13.9 mCi, was well replicated by the 236 ± 20.1 mCi experimentally obtained. The difference of only 14% is expected and acceptable; it can be attributed to the process losses of activity, mainly in the purification step, and also to the uncertainty of the cross-section values used, geometry, and uneven distribution of the mass in the real target.
Characterization of the [64Cu]CuCl2 solution
To eliminate the metallic contaminants, the solution containing [64Cu]CuCl2 was purified by the cation exchange technique in the purification module. The acidic solution obtained after the dissolution of the target with HCl was transferred to the purifying column loaded with 9 g of AG1-X8 resin. The nickel of the target was recovered with 40 ml of HCl 6M in chloride form (NiCl2) followed by washing of metallic impurities with HCl 4M. 64Cu was eluted with HCl 0.5 M in the form of 64CuCl2. The gamma spectrum acquired before purification is presented in Figure 8, where the expected cobalt isotopes 56Co, 57Co, and 58Co, were identified, confirming the simulation results. As expected, the radionuclides with shorter half-lives, such as 60Cu, 61Cu, and 62Cu, were not detected in the analyzed sample.
After purification, a sample consisting on 2 μl of [64Cu]CuCl2 was analyzed, identifying the characteristic peaks of 64Cu and checking the presence of other possible radionuclide impurities. The gamma spectrum presented in Figure 9 shows that only the characteristic peak of 64Cu (1,345 keV) and the annihilation peaks (511 keV and 1,022 keV) were identified, confirming the high yield of separation and the excellent radionuclide purity >99.99% of purified [64Cu]CuCl2 solution.
Conclusion
Monte Carlo simulation of the 64Cu production route was in good agreement with the experimental results obtained through 64Ni(p,n)64Cu reaction, at the TR-19 cyclotron. Comparing the activity of 64Cu experimentally obtained versus the simulated activity, a 14% difference can be considered a very good result, being justified by the process losses during target irradiation and transfer, and accepted sum of uncertainties, such as activity measurements and cross-section values, target assemble geometry and mass distribution of the target. There is also an unavoidable activity loss in the post-irradiation processing (dissolution and purification).
One of the most important parameters is the radionuclide purity (>99.99%) of the final solution, which indicates that the simulation provided correct indications, and the entire process implemented on our fully automated solid target system can be successfully used for the production of 64Cu with radiopharmaceutical grade purity.
The confirmation of the simulated results with such a narrow error gives the confidence to use this simulation code for the optimization of production processes, aimed at pharmaceutical grade radioisotopes of medical interest, with different energies ranges, on the same configuration of the automated solid target system, e.g. 89Y (p,n)89Zr, 68Zn (p,n)68Ga, 44Ca (p,n)44Sc.
Data availability statement
The original contributions presented in the study are included in the article/supplementary material, further inquiries can be directed to the corresponding authors.
Author contributions
SB writing and edited the article, reviewed the data regarding copper radioisotope production; physical-chemical analyses, performed simulations regarding copper radioisotope production using the 64Ni(p,n)64Cu route, RL reviewed the data regarding copper radioisotope production and radiochemistry, DC reviewed the data regarding the process of copper radioisotope production, LC reviewed the data regarding TR-19 cyclotron, DN conceived and designed the experiments, and managed resources, CAU verified and reviewed the simulation results. All authors discussed the results and contributed to the final manuscript.
Funding
This work was supported by a grant of the Ministry of Research, Innovation and Digitization, CNCS/CCCDI - UEFISCDI, project number ERANET-EURONANOMED-3-I2PAD, within PNCDI III.
Conflict of interest
The authors declare that the research was conducted in the absence of any commercial or financial relationships that could be construed as a potential conflict of interest.
Publisher’s note
All claims expressed in this article are solely those of the authors and do not necessarily represent those of their affiliated organizations, or those of the publisher, the editors and the reviewers. Any product that may be evaluated in this article, or claim that may be made by its manufacturer, is not guaranteed or endorsed by the publisher.
References
1. Szymański P, Frączek T, Markowicz M, Mikiciuk-Olasik E. Development of copper-based drugs, radiopharmaceuticals and medical materials. Biometals (2012) 25:1089–112. doi:10.1007/s10534-012-9578-y
2.Cu-64_tables. Cu-64_tables.pdf (2022). [Online]. Available at: http://www.nucleide.org/DDEP_WG/Nuclides/Cu-64_tables.pdf (Accessed March 8, 2022).
3. Anderson CJ, Ferdani R. Copper-64 radiopharmaceuticals for PET imaging of cancer: Advances in preclinical and clinical research. Cancer Biother Radiopharm (2009) 24:379–93. doi:10.1089/cbr.2009.0674
4. Niculae D, Dusman R, Leonte RA, Chilug LE, Dragoi CM, Nicolae A, et al. Biological pathways as substantiation of the use of copper radioisotopes in cancer theranostics. Front Phys (2021) 8:568296. doi:10.3389/fphy.2020.568296
5. Mazière B, Stulzaft O, Verret JM, Comar D, Syrota A. [55Co]- and [64Cu]DTPA: New radiopharmaceuticals for quantitative tomocisternography. Int J Appl Radiat Isot (1983) 34:595–601. doi:10.1016/0020-708x(83)90061-3
6. Leonte RA, Niculae D, Crăciun LS, Căta-Danil G. Medical radioisotopes production at TR-19 cyclotron from IFIN-HH. UPB Scientific Bull Ser A: Appl Math Phys (2017) 79:223–36.
7. Leonte RA, Cocioabă D, Chilug LE, Băruță (Ilie SI, Eșanu TR, Burghelea B, et al. Process validation for production of copper radioisotopes in a TR-19 variable energy cyclotron. AIP Conf Proc (2020) 2295:020022. doi:10.1063/5.0031538
8. Synowiecki MA, Perk LR, Nijsen JFW. Production of novel diagnostic radionuclides in small medical cyclotrons. EJNMMI Radiopharm Chem (2018) 3:3. doi:10.1186/s41181-018-0038-z
9. Maiko K, Carey P, Gaehle G, Madrid E, Voller T, Margenau W, et al. A semi-automated system for the routine production of copper-64. Appl Radiat Isot (2012) 70:1803–6. doi:10.1016/j.apradiso.2012.03.009
10. Ohya T, Nagatsu K, Suzuki H, Fukada M, Minegishi K, Hanyu M, et al. Efficient preparation of high-quality 64 Cu for routine use. Nucl Med Biol (2016) 43:685–91. doi:10.1016/j.nucmedbio.2016.07.007
11. McCarthy D, Shefer R, Klinkowstein R, Bass L, Margeneau W, Cutler C, et al. Efficient production of high-specific-activity 64Cu using a biomedical cyclotron. Nucl Med Biol (1997) 24:35–43. doi:10.1016/s0969-8051(96)00157-6
12. Szelecsényi F, Blessing G, Qaim S. Excitation functions of proton induced nuclear reactions on enriched 61Ni and 64Ni: Possibility of production of No-carrier-added 61Cu and 64Cu at a small cyclotron. Appl Radiat Isot (1993) 44:575–80. doi:10.1016/0969-8043(93)90172-7
13. Obata A, Kasamatsu S, McCarthy D, Welch M, Saji H, Yonekura Y, et al. Production of therapeutic quantities of 64Cu using a 12 MeV cyclotron. Nucl Med Biol (2003) 30:535–9. doi:10.1016/s0969-8051(03)00024-6
14. Agostinelli S, Allison J, Amako KA, Apostolakis J, Araujo H, Arce P, et al. Geant4 - a simulation toolkit. Nucl Instrum Methods Phys Res A (2003) 17:250–303. doi:10.1016/S0168-9002(03)01368-8
15. Poignant F, Penfold S, Asp J, Takhar P, Jackson P. GEANT4 simulation of cyclotron radioisotope production in a solid target. Physica Med (2016) 32:728–34. doi:10.1016/j.ejmp.2016.04.006
16.Geant4. Geant4 10.6 release notes. 2019. [Online]. Available at: https://geant4-data.web.cern.ch/ReleaseNotes/ReleaseNotes4.10.6.html (Accessed March 8, 2022).
17.EXFOR. Experimental nuclear reaction data (EXFOR). 2022. [Online]. Available at: https://www-nds.iaea.org/exfor/ (Accessed March 8, 2022).
18. Ursu I, Craciun L, Niculae D, Zamfir NV. The radiopharmaceuticals research Centre of IFIN-HH at start. Rom J Phys (2013) 58:1327–36.
19.ACSI. Advanced cyclotron systems Inc. (ACSI). 2003. [Online]. Available at: http://www.advancedcyclotron.com/cyclotron-solutions/tr19 (Accessed March 8, 2022).
20.ALCEO. Solid target processing system (ALCEO). 2019. [Online]. Available at: https://www.comecer.com/alceo-solid-target-processing-system (Accessed March 8, 2022).
Keywords: 64Cu production, cyclotron production, 64Ni target, nuclear reactions, Geant4 simulation
Citation: Baruta S, Leonte R, Cocioaba D, Craciun L, Ur CA and Niculae D (2022) Cyclotron production of 64Cu by proton irradiation of enriched 64Ni target: Validation of Geant4 simulation parameters through experimental data. Front. Phys. 10:1038014. doi: 10.3389/fphy.2022.1038014
Received: 06 September 2022; Accepted: 15 November 2022;
Published: 30 November 2022.
Edited by:
Michela Marafini, Centro Fermi - Museo storico della fisica e Centro studi e ricerche Enrico Fermi, ItalyReviewed by:
Riccardo Mirabelli, Sapienza University of Rome, ItalyFrancesco Collamati, Universities and Research, Italy
Copyright © 2022 Baruta, Leonte, Cocioaba, Craciun, Ur and Niculae. This is an open-access article distributed under the terms of the Creative Commons Attribution License (CC BY). The use, distribution or reproduction in other forums is permitted, provided the original author(s) and the copyright owner(s) are credited and that the original publication in this journal is cited, in accordance with accepted academic practice. No use, distribution or reproduction is permitted which does not comply with these terms.
*Correspondence: Simona Baruta, simona.baruta@nipne.ro; Dana Niculae, dana.niculae@nipne.ro