- 1State Key Laboratory of Advanced Optical Communication, System and Network, School of Electronics, Peking University, Beijing, China
- 2Key Laboratory for the Physics and Chemistry of Nanodevices, School of Electronics, Peking University, Beijing, China
This review aimed to recount the scientific career and contributions of Prof. Wang Yiqiu, as well as his contribution to the research on quantum precision measurement and cold atom physics, as a tribute to his upcoming 90th birthday. Having contributed greatly to fields of research such as nuclear magnetic resonance, microwave atomic clocks, laser cooling of atoms, Bose–Einstein condensate, optical tweezers, and optical atomic clocks, the venerable Prof. Wang is a prominent figure in these research fields in China and has played a pivotal role in China’s development of these subjects.
Introduction
Prof. Wang Yiqiu, born on September 1932, has been a prominent figure in China’s research on quantum precision measurement and cold atom physics. Early on in his career, he studied nuclear magnetic resonance as a graduate student at Leningrad University and independently made several important discoveries. After his return from the Soviet Union, he turned his expertise toward China’s development of microwave atomic clock, establishing China’s first cesium beam atomic clock, as well as systematically explaining the Majorana shift in cesium beam atomic clocks. During his research on atomic clocks, his experience with laser frequency stabilization allowed him to pioneer China’s earliest studies on the laser cooling of atoms, Bose–Einstein condensates and optical tweezers. In addition, Prof. Wang provided valuable insights and inspiration for the research of optical atomic clocks. This article aimed to recount Prof. Wang’s scientific career and contributions, in honor of his academic legacy, as well as in celebration to his upcoming 90th birthday.
Nuclear magnetic resonance
In 1957, Wang Yiqiu entered the Physics Department of Moscow State University to study radio and microwave spectroscopy. In 1958, he transferred to Leningrad University and became a graduate student in the Prof. Scripov’s group of radio-frequency spectroscopy. Prof. Scripov was a young and innovative scientist. He first invented the Fourier transform with nuclear magnetic resonance free induction signal to solve the problem of the high-resolution spectrum. He also invented a radio-frequency quantum oscillator (perhaps it could be called “Raser”), which can accurately measure the strength of the earth’s magnetic field. In the first year, he suggested that Wang Yiqiu prepared some experimental instruments while passing all radio science course examinations. The most important thing was to build a high uniformity electromagnet with a stable magnetic field. With the help of laboratory experience, Wang Yiqiu made an electromagnet with a magnetic field controlled at about 4000 G (0.4 T) and an electronic circuit with higher sensitivity at the end of 1959. This device can be used to measure NMR signals of liquids and solids. Prof. Scripov suggested that Wang Yiqiu measured the chemical shifts of fluorine NMR signals in some fluoride solutions, especially to determine the chemical shifts of HF molecules. This is of certain significance because there were differences in this value in the scientific literature of the Soviet Union at that time, which is related to the explanation of the properties of chemical bonds in HF molecules. By systematically measuring the concentration effect of aqueous solution of KHF2–H2O, Wang definitively determined the chemical shift value of the HF molecule and the discrepancy was eliminated. Prof. Scripov sent the research study to the most authoritative scientific journal in the Soviet Union, ДАН CCCP (report of Soviet Academy of Sciences) [1].
Thereafter Wang Yiqiu measured the chemical shifts of fluorine NMR signals in aqueous solution systems of some fluorides. The results were published in a journal of structural chemistry [2]. However, Prof. Scripov suggested that he did a more difficult task. That was to find the anisotropy in the chemical shift of NMR signals in solids. The subject he proposed was graphite. Apparently, its layer structure indicates that the chemical bond of the carbon atoms within the same layer should be different than that between layers, so the anisotropy would appear in the chemical shifts of the 13C isotope NMR signals if the experiments were conducted when the graphite layers were parallel to the magnet field or perpendicular to that. This is because the natural abundance of the 13C isotope is only about 1.1% (usual 12C isotope nuclei have no magnet moment and no NMR signals). Furthermore, the NMR frequency of the 13C isotope in the same magnetic field is about 1/4 compared with the 19F (its abundance is 100%), according to the general rule, the intensity of the NMR signals is proportional to the cubic of frequency, in this regards; the sensitivity of the 19F signals is more than 5000 times to that of 13C. So, Wang Yiqiu suspected that he could detect the 13C NMR signal. Nevertheless, he had improved the sensitivity of his homemade NMR spectrograph and finally detected the fluorine signals in LiF solid powder with a good signal-to-noise ratio. However, for detecting the 13C signal in the graphite, Wang Yiqiu had estimated that the sensitivity of his instruments would need to be raised at least more than one order of magnitude. It was impossible at that time in his laboratory.
Ironically, while Wang Yiqiu had been bothered by improving the sensitivity of his apparatus to measure the anisotropy of the 13C NMR signals, he found a study by Lauterbur in “Physical Review Letters,” who had discovered the anisotropy of the 13C NMR signals in CaCO3 crystal [3] (In 2003, he got the Nobel Prize in Physiology and Medicine for his invention of NMR imaging technology). So, searching for this phenomenon had lost any significant physical meaning. However, when Wang Yiqiu tested the signals in LiF crystalline powder, he also got the 19F signal for NH4F crystalline powder. Fortunately, he found that the data of the chemical shifts of the 19F signals for these two crystals were different on a big scale. Then Wang Yiqiu tried to get all the alkaline and alkaline-earth fluoride crystals, and carefully measured the values of the chemical shift for each of them. He conducted the measurement from midnight to 3 or 4 o’clock morning for the lowest noise. It was clear that the value of the chemical shift for every fluoride depends on the atomic number of the neighboring metal, with exception for some light metals. The overall results are shown in Figure 1 [4]. One can see that, in general, the chemical shift decreases with the atomic number of the alkali or alkali-earth metal increases with exception for LiF. This systematic rule of the chemical shifts for the 19F NMR spectra of the alkaline and alkali-earth fluoride crystals brought great attention to Prof. Scripov, and he asked Wang Yiqiu to write an article soon. It was published also in “ДАН CCCP” [5], but Prof. Scripov refused to be an author. He said, all ideas and explanations belonged to Wang, and he should just mention Prof. Scripov in the acknowledgment. The work was of interest to other groups [6–9], and there were still some research groups citing this work after 10 years.
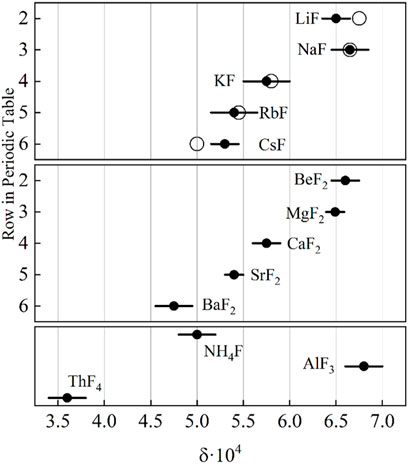
FIGURE 1. Chemical shift of the 19F NMR signal in different fluoride crystals, the black dots with the error bar are the experimental results, the circle is theoretical value calculated from [11].
For explaining the experimental facts, Wang Yiqiu had read a lot of literature as well as some famous books, such as “Nature of the Chemical Bond” (Pauling),“Dynamic Theory of Crystal Lattice” (Born M. and Huang Kun), and especially the “Structure of Matter” (Xu Guangxian, in Chinese). According to the Ramsey theory [10] of the chemical shifts, they originated from the different shielding effects of the electrons around the nucleus to the outside magnetic field. They consist of two parts, the first part is the effect of the electrons of the inner spherical closed shells, which gives rise to the diamagnetic effect; and the other part is contributed by outer valence electrons, which may give rise to a total magnetic moment, and it will not shield, but strengthen the magnetic field outside. This is a paramagnetic effect and produces a negative value for the shielding factor. Wang Yiqiu recognized that in these ionic crystals there exists also some partial covalent bond, and the chemical shifts might be related to the deformation of this outer shell valence electron wave function of the fluorine ion F- in different fluoride crystals during their combination with metal atoms to form a crystal. In the literature, Wang Yiqiu found several studies that dealt with the bond characteristic of the different crystals. However, most of them could not explain the experimental results. Finally, he found a study by [11], which proposed that the overlap of the wave functions of the electrons of the fluorine and the neighboring metal in the free ion state may approximately give rise to the chemical shift for these ionic crystals. They give a formula, which is related to the overlap integrals of the two-electron wave functions and the mean distance between two atoms. For the latter, Wang Yiqiu could use the data from the book of Born and Huang Kun, but the overlap integrals should be calculated from the known wave functions of the outer electrons for both fluorine and alkali and alkali-earth metal ions, at least, for the outer shell electron. Furthermore, Wang Yiqiu had no idea how to calculate these overlap integrals and did not know the wave functions of the electrons with different quantum numbers for all these atoms. Prof. Scripov introduced Wang Yiqiu to the director of the theoretical physics division of the Institute, Professor Petrashin. Fortunately, he was delighted to help Wang Yiqiu to perform this work. He nominated a clever student of the last undergraduate year to help Wang Yiqiu in the calculation. They had all the data of the Hartree or Hartree–Fock wave functions for the fluorine and alkali atoms, but no such data for alkali-earth atoms. At that time the electronic computer was not popular. But the work load was huge. So they performed this calculation in a machinery calculation factory for more than 1 month. Then they used the formula of [11] to get the results in Figure 1 (circle for each alkali fluoride crystal). Though the theory was not so accurate, the theoretical data agree with the experiment rather well. The discrepancy for the LiF crystal can be easily explained by the fact that in comparison with other ions the volume of the Li+ ion is too small, so the repelling force between neighboring F-ions will distort the wave functions and result in a smaller chemical shift. With these results, Wang Yiqiu earned the Degree of the Candidate of the Physics-Mathematical Sciences in 1961. Afterward, the theoretical division worked out some new data of the electron wave functions modified by the crystalline field, they calculated again for these overlap integrals, and produced even better results considering some electron wave functions for the next nearest neighboring ions. They were published after Wang Yiqiu returned to China [12]. These results were interesting also because from them people could estimate the chemical shift figure for the nude (without electron shielding) fluorine nucleus [6–9, 13–20] and thus got approximately the value of the nuclear magnetic moment of fluorine. It has some meaning in nuclear physics, so far, the only one which we know the accurate value of its nuclear magnetic moment is hydrogen . Vice-president of Peking University, a famous theoretical physicist, Professor Wang Zhuxi suggested Wang Yiqiu to write a study concerning his thesis and to publish in “Acta Physica Sinica,” and meanwhile translate it in Russian to publish in the “Science China” also. The former had come out in 1984 [11]. The latter had passed the evaluation by editors of this journal but failed because of the mistake of Peking University administration. Fortunately, this study has been translated into English in the United States and published in a journal of “Chinese Physics.” Wang Yiqiu’s work on the alkali fluoride crystals has been repeated in the United States more than 10 years later, and the results and conclusion were very similar [8].
When Wang Yiqiu came back to Peking University, he belonged to the newly established Department of Radio-Electronics in the Division of the Radio-Frequency Spectroscopy. At that time, they had a group of NMR, possessing a commercial 40 MHz high-resolution NMR spectrograph with a permanent magnet and a homemade spectrograph with less sensitivity. It was impossible to perform the work that Wang Yiqiu supposed. It was the temperature or pressure effects of the chemical shifts in alkali fluorides because of the lack of both an appropriate NMR spectrograph and the useful set-up for change of the temperature or pressure on a large scale. With the great help of Professor Xu Guangxi of the Chemistry Department and Professor Qian Renyuan of the Chemistry Institute of Academy of Science, they organized a systematic seminar on NMR application in chemistry, utilizing the 40 MHz spectrograph as a practical tool. After more than 1 year, the department moved to the new campus in Changping, the liaison with chemistry was cut off totally by long distance. Also, the name of the division was changed to “Radio-Frequency Spectroscopy and Quantum Electronics.” His research task turned to magnetic optical double resonance, and his research direction began to change. However, in Changping, Wang Yiqiu with his two advanced students spent 2 years to made a radio-frequency quantum generator by using the Overhauser effect in the aqueous solution of K2(SO3)2NO in a weak magnetic field, and observed the building up process of stimulated emission in details [21]. It could simulate the laser establishing process and develop a magnetometer. The research method of nuclear magnetic resonance—the interaction between the electromagnetic field and matter affects his subsequent research: atomic clock, laser cooling, and Bose–Einstein condensation.
Microwave atomic clocks
In the recent decades, microwave atomic clocks have seen tremendous progress in the development of various aspects of their performance [22, 23]. Prof. Wang Yiqiu is a pioneering figure in the research on quantum frequency standards in China [24]. In 1961, Wang Yiqiu returned from the Soviet Union and was appointed director of the section of Spectrum and Quantum Electronics. In 1963, Wang Yiqiu began to lead the research on the atomic clock based on optically pumped cesium vapor cells. At the end of 1965, the optically pumped cesium vapor cell atomic clock was successfully developed, and the stability of the three prototypes was from 1 × 10–10 to 5 × 10–11. In 1966, this research was interrupted because of the Cultural Revolution. In 1972, Wang Yiqiu and his colleague began to work on optically pumped vapor cell atomic clock again, but instead of cesium, this time they used rubidium isotopes, and worked in cooperation with the Beijing Dahua Electronic Instrument Factory. In the beginning of 1976, their design passed the appraisal of the Ministry of Electronics for mass production, and in 1978, they had produced more than 200 sets of Rb frequency standards; the physical parts were completed in the Hanzhong Peking University Branch. This work has been awarded with a National Science Conference Prize. After 1976 Wang Yiqiu has led the development of magnetic-state-selected cesium beam atomic clocks. Figure 2 shows a photo of Prof. Wang working on a cesium tube atomic clock. In 1981, Wang first reported observations on Majorana transitions in cesium beam atomic clocks [25]. Majorana transitions are indicated by a subsequent peak in the intensity distribution of the deflected beam and asymmetry in hyperfine σ transitions resonance which reverses with the C-field. Wang also made further detailed analyses of Majorana transitions in a follow-up study [26], drawing the conclusion that Majorana transitions are mostly excited by the inhomogeneity of the C-field, which is the magnetic field used in the drift phase of cesium beam microwave clocks for separating hyperfine levels. The discovery of Majorana transitions shed new light on the underlying physical principles of the cesium beam atomic clock and provides new insight into the evaluation and elimination of frequency shifts in atomic clocks [27, 28]. It was found that Majorana transitions exist not only in cesium beam atomic clocks with magnetic state selection, but also affect the transition frequency in optically pumped cesium beam clocks [27] and atomic fountain clocks [28]. Prof. Wang also proposed an experimental scheme for realizing a continuous atomic fountain clock [29, 30], and the concept is being developed in JILA.
In 1980, Prof. Wang Yiqiu also began to lead the development of optically pumped cesium beam atomic clocks, deducing a formula of the clock signal for different pumping and detecting schemes [31]. Then they built up the first prototype optically pumped cesium beam atomic clock in China [32, 33]. In the 1990s, after receiving his doctorate from the United States, Yang Donghai returned to Peking University to join Professor Wang’s optically pumped cesium atomic clock research group [34–36]. Together with Yang, Prof. Wang proposed and realized an optically pumped cesium beam atomic clock with a sharp angle incidence probe laser [37–40]. This novel design utilizes the Doppler shift and broadening from the atomic beam to drastically reduce the effect of laser frequency noise on the signal-to-noise-ratio and is widely used in compact atomic beam frequency standards [41–43]. Since the effect of laser frequency noise on the clock’s SNR is reduced, a similar level of clock performance can be achieved without sophisticated laser frequency stabilization schemes, thereby greatly reducing the size and complexity of the system. They obtained the long-term stability data for this kind of frequency standard first [40]. For their outstanding work, Prof. Wang and Prof. Yang received the Rao Yutai Award from the Chinese Physics Society in 1993. In his research on compact cesium beam atomic clocks, Wang also contributed greatly to the development of compact frequency-stabilized diode laser systems that are utilized as pumping and probing lasers in the atomic clocks, reducing the size of the physical package to below 10 × 10 × 5 cm3 while maintaining a high level of frequency stability [44, 45]. From 1995 to 2005, with the help of Prof. Wang Yiqiu, Prof. Yang Donghai and associate professor Wang Fenzhi were granted some national projects to develop a compact optically pumped cesium clock. Doctoral students, Chen Jingbiao and Zhang Junhai are the key members in the team to build a compact optically pumped cesium atomic clock [36].
Wang’s pioneering work on quantum precision measurement, atomic clocks, in particular, has greatly inspired China’s development of commercial atomic clocks, with various institutes such as Peking University [46, 47], Chengdu Spaceon Electronics Co., Ltd. [48], and Lanzhou Institute of Physics [49, 50] all working toward commercial clocks with high performance at a relatively low cost.
Recent works on experimental cesium atomic clocks have also benefitted greatly from helpful discussions with and suggestions from Prof. Wang. Among these works are optically pumped cesium-beam atomic frequency standards by Peking University [51] and collaboration between Peking University and Chengdu Spaceon Electronics Co., Ltd. [52], reaching 3e−12/√τ and 2e−12/√τ frequency stability, respectively. Wang’s work also inspired research on optically pumped cesium beam atomic clocks with multi-pole magnets for magnetic focusing of the atomic beam [53, 54].
In order to further improve the technique of the optically pumped cesium atomic clock and meet the needs of the country, under the instruction and help of Prof. Wang Yiqiu and Prof. Yang Donghai, Prof. Chen Xuzong together with his PhD students, Qi Xianghui (now is an associate professor) and Wang Qing (now is a lecturer) inherited research on the optically pumped cesium atomic clock from 2008, Chen’s team was granted four terms of national projects to study the high-performance optically pumped cesium clocks since 2008. The goal is to achieve the stability of the 5071A high-quality cesium atomic clock. During the research, first, the theoretical analysis of the interaction between atomic beam and light and microwave is carried out [55], and the main factors affecting the high stability of atomic clock are found. It has improved the stability of laser frequency stabilization, reduced the noise of laser frequency stabilization [56, 57], and improved the frequency stability and power stability of microwave. After more than 10 years of efforts, the stability of miniaturized optically pumped cesium atomic clock has been improved to 3 × 10–12/√τ, which is three times that of the 5071A high-quality clock [58], and the 5-day stability is better than 7 × 10–15 [59, 60]. Figures 3A,B show the frequency stability and photo of the portable optically pumped cesium clock developed by Peking University.
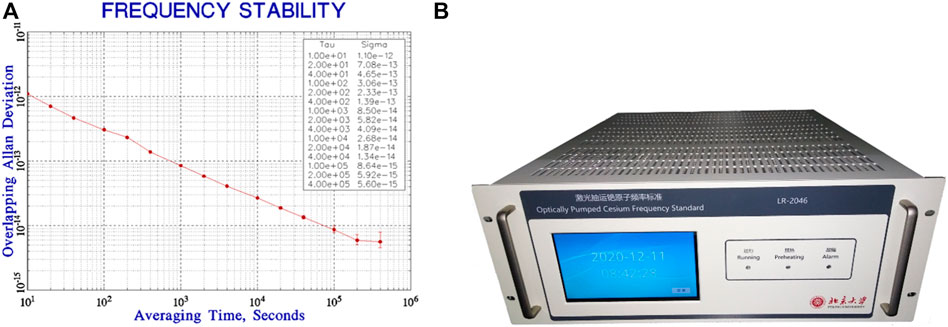
FIGURE 3. (A) Frequency stability curve for the optically pumped cesium clock. (B) Portable optical pumped cesium clock.
Meanwhile, another new structure of the compact cesium beam clock is also under development by an associate professor, Dr. Wang Yanhui, collaborating with his formal supervisor, Prof. Dong Taiqian and associate professor Liu Shuqing at Peking University. The optically detected the magnetic-state-selected cesium beam clock (OMCC) combines both the advantages of the magnetic-state-selected schemes and the optically detection schemes [61]. It avoids the technical issues concerning the lifetime of the electron multiplier and has narrower velocity distribution, resulting in a narrower Ramsey linewidth. The schematic and Ramsey fringe of the OMCC is shown in Figure 4.
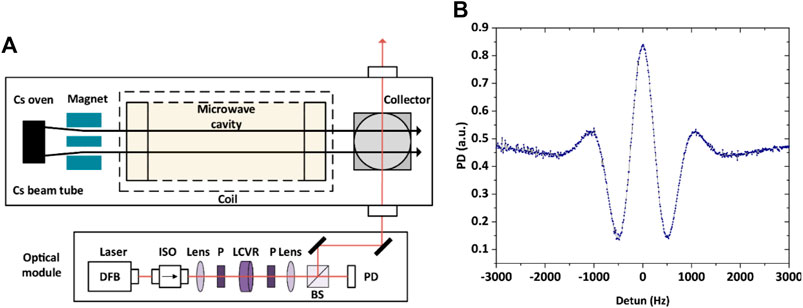
FIGURE 4. (A) Configuration of OMCC. ISO, isolator; P, polarizer; lens, convex lens; BS, beam splitter; PD, photodiode; LCVR, liquid crystal variable retarder. (B) Ramsey fringe.
The fluorescent spectroscopy is adopted to stabilize the laser frequency. Laser frequency is kept resonant with the atomic beam, and the mis-locking problem is avoided.
The frequency stability test result of five OMCCs at the National Institute of Metrology in China is plotted in Figure 5. The frequency reference is an active hydrogen maser and the measurement time is over 15 days. The result shows promising frequency stability and good consistency of OMCCs.
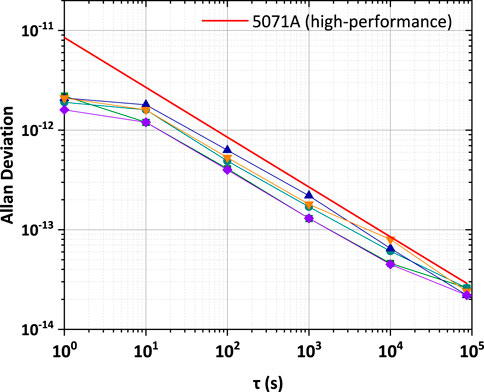
FIGURE 5. Frequency stability test result of five OMCC in comparison with the high-performance version of 5071A.
Laser cooling
In order to improve the accuracy of atomic clocks, it is necessary to reduce the temperature of free atoms. In the 1980s, scientists proposed many methods to cool neutral atoms, among which Steven Chu, Claude Cohen-Tannoudji, and Bill Philips, three outstanding scientists, won the 1997 Nobel Prize in physics. In the 1980s, the research on laser cooling in the world developed rapidly in the world, but the research in China was relatively lagging behind. In 1994, the Chinese Nature Science Foundation (NSFC) began to support the research on laser cooling. Professor Wang Yiqiu was granted funding by the major project of NSFC. The project is: research on the new cesium atomic frequency standard based on the atomic fountain clock. Peking University has set up a laser cooling research group headed by Professor Wang Yiqiu.
There are many technical problems to be solved in the construction of the magneto-optical trap. In addition to vacuum technique and frequency stabilization technique, there are also many techniques such as laser amplification, laser frequency shift, magneto-optical trap laser alignment, and fluorescence detection . These techniques were new challenges for the team. Under the guidance of Prof. Wang, the team successfully solved these techniques problems [62–64].
In the process of building a magneto-optical trap, in addition to frequency stabilization laser, semiconductor laser amplification is also very important. Since the power of the purchased laser diode is about 50 mW, the power is not enough to cool atoms, so it needs to be amplified. But there was no power-amplified diode at that time. The team developed the “large frequency difference injection locking” technique which was used for a high power diode with multiple modes. With this technique, 852 nm wavelength laser injection locking can be realized by changing the laser current or fine-tuning the laser diode temperature at room temperature. In this way, the stable laser output with narrow laser line-width, long-term stable frequency, and power greater than 300 mW is achieved, which meets the light source conditions required by the magneto-optical trap [65, 66].
After the laser and optical system were ready, the research team began to implement the magneto-optical trap experiment. At that time, as deputy president of Peking University, Prof. Wang was very busy during the day, but he came to the laboratory every night . The experiment was mainly conducted by post-doctor, Dr. Chen Xuzong, and doctoral students: Li Yimin and Gan Jianhua. Professor Wang Yiqiu directed the laboratory. After 3 days of continuous experiments, cold atoms were finally obtained by using the magneto-optical trap at 10:30 p.m. on April 16, 1996, which was also the first cesium magneto-optical trap in China (Newspaper Wen Wei Bo and others reported this news) [67]. The photo and atomic distribution are shown in Figure 6.
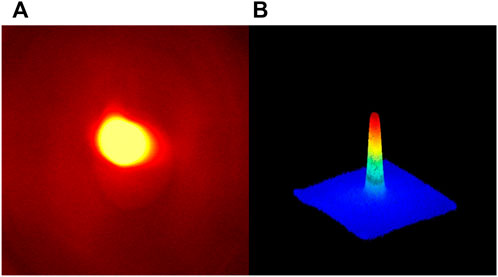
FIGURE 6. (A) First cesium magnetic optical trap in China. (B) Cold atoms with 100 μK and number of 1E+9 at Peking University in 1996.
Later, Professor Wang Yiqiu continued to guide doctoral students Fu Junxian and Dr. Li Yimin to carry out optical molasses experiments, and Hou Jidong, Wang Xiaohui and Chen Shuai to carry out atomic fountain experiments. The first optical molasses in China was obtained in 1997 [68, 69], and the first atomic fountain experiment in China was demonstrated in 2000 [70–72]. After 2000, Prof. Wang Yiqiu’s cold atom group began to turn its research direction to Bose–Einstein condensation. In order to build the atomic fountain microwave atomic clock in China more quickly, Prof. Wang Yiqiu went to the Chinese National Institute of Metrology (NIM) very enthusiastically to help the research group that is building the atomic fountain microwave atomic clock there and introduced the NIM research group about how Peking University realized the magneto-optical trap and optical molasses. It accelerates NIM to build up the first atomic fountain microwave atomic clock in China .
To reduce the atoms to an ultra-low temperature has always been a goal pursued by scientists engaged in atomic cooling research. Due to the influence of the earth’s gravity, when the potential barrier of any potential well that traps atoms is reduced to a certain extent, a leak appears at the bottom of the potential well, which stops evaporative cooling. The space microgravity environment provides a new opportunity to further reduce the atomic temperature. In 2011, China began to develop the space station. It plans to launch the space station and the scientific experiment module from 2021 to 2022, and 14 scientific experimental racks will be launched with the module, including the cold atomic physics rack (CAPR). The development of the space ultra-cold atomic physics rack was jointly undertaken by Peking University and Shanghai Institute of Optics and Fine Mechanics, Chinese Academy of Sciences. Professor Chen Xuzong, who was originally a member of Prof. Wang Yiqiu’s team, was appointed as the chief scientist for CAPR. In 2015, Dr. Xiong Wei joined the project for CAPR project as a deputy chief designer.
CAPR is a very advanced scientific experimental platform for ultra-cold atom physics. At present, only the United States and China are carrying out research in this field. The CAPR will take advantage of the microgravity environment to create a new extreme cold atomic sample, develop the lowest temperature record in the universe (10–12 K, that is, pK level ultra-low temperature), and build an open experimental system of Bose and Fermi quantum degenerate working substances with ultra-low temperature, large scale, high quality, and suitable for long-term precise measurement. The platform will enable the regulation of cold atoms to reach a new parameter region, so that a series of ultra-cold atom physics experiments under extreme conditions can be carried out, including simulation of strongly correlated multi-body quantum systems, exploration of novel quantum states, acoustic black hole simulation and Hawking radiation observation, and verification of basic physical laws. Relevant work will bring breakthroughs to the fields of quantum physics and precision physical measurement, and improve the level of new aerospace technology in China, including the ultra-old atomic vacuum system, ultra-high stability laser system, precision integrated optical system, and related precision intelligent control system suitable for thr space environment.
Peking University has put forward a new scheme of deep cooling under microgravity conditions, which uses an all-optical trap for two-stage cooling [14–17]. The basic idea was to use two cross laser beams with different beam diameters and different powers to carry out evaporative cooling and diffusion cooling, respectively, under special timing control, so as to obtain a quantum gas with a temperature of 100 pK in a short time (such as 10 s). The aforementioned scheme has been verified by ground experiments [18]. In order to obtain a very low limit temperature, it is necessary to reduce the influence caused by the vibration of the space station itself during the experiment on the space station. Therefore, Peking University theoretically studied the influence of the vibration spectrum of the space station on the atomic cooling limit [19], and proposed a solution on this basis. Based on two-stage cooling, further cooling of the mean-field energy and reducing the relative temperature, the new proposals are also given by the PKU group [20]. In 2020, Peking University was invited to publish a review stydy [35] in an internationally renowned magazine, introducing how to break the limit of ground gravity, obtain a temperature of pK under microgravity conditions, and conduct challenging experiments, it also introduced the important applications in future fundamental research [73] based on 100 pK ultra-cold atoms.
In 2021, the ultra-cold atomic physics experiment platform project progressed smoothly, and Peking University and Shanghai Institute of Optics and Precision Machinery, Chinese Academy of Sciences, have made many phased achievements, respectively. The functions of the prototype and the normal prototype of CAPR have been verified on the ground, meeting the requirements of the experiment after the launch at the end of 2022.
In order to further use this platform to carry out a series of scientific experiments, in 2021, the Chinese Ministry of Science and Technology organized a national key research and development program: “Research on the strange physical properties of extremely low-temperature atoms in the space microgravity environment,” with Professor Zhou Xiaoji. The experiment of “superfluid to the model phase transition of one-dimensional to three-dimensional hexagonal optical lattice” [74] proposed by Professor Zhou Xiaoji, will become the first quantum simulation experiment in the series of experiments based on CAPR.
Bose–Einstein condensate
In 1925, Satyendra Nath Bose and Albert Einstein predicted that the Bose gas would behave as an extraordinary phenomenon near absolute zero temperature [75, 76]. Under this condition, the vast majority of atoms occupy the quantum ground state, producing a macroscopic wave function called Bose–Einstein condensate (BEC) [76]. In recent years, with the development of laser cooling technologies, people have had the opportunity to cool atoms to very low temperatures. In 1995, Eric Cornell and Carl Wieman at the University of Colorado at Boulder NIST-JILA laboratory, and Wolfgang Ketterle at Massachusetts Institute of Technology prepared and observed BEC in the rubidium atomic system and sodium atomic system, respectively [77, 78]. For this, Cornell, Wieman, and Ketterle won the 2001 Nobel Prize in physics. Professor Wang Yiqiu of Peking University is also one of the first scientists in China to start the research on Bose–Einstein condensates and prepared China’s second Bose–Einstein condensates in 2004 [79, 80]. Prof. Wang Yiqiu was interested in building up an atom laser, which is a coherent atomic beam coupled out from the BEC trap. The pulsed and quasi-continuous atom laser was realized in 2005 [81], as shown in Figure 7. In 2000, under the instruction of Prof. Wang Yiqiu, doctoral student Zhou Xiaoji proposed a scheme for realizing the long-term continuous output of an atom laser. In this scheme, the cold atoms in several subsidiary Bose–Einstein condensates (BECs) would be coupled one by one, in turn, into one main BEC, which served for the continuous output of a coherent atomic beam [82, 83]. This kind of scheme was realized in the Ketterle group at MIT by using the sophisticated technique of optical tweezers [84]. In 2001, Prof. Wang Yiqiu and Prof. Chen Xuzong led the group to build up the setup for BEC, the key members were associate professor Zhou Xiaoji, doctoral students: Chen Shuai, Yang Fan, and Xia Lin. They used RF evaporative cooling technology to obtain a 87 Rb Bose–Einstein condensate in a QUIC trap, and used Majorana technology to obtain a multi-component BEC. In 2004, the team published first studies on BEC experiments in international journals [85, 86], and obtained the first atom laser in China in 2005 [81, 87]. [88] studied intensity fluctuations of the pulsed atom laser. They found that the atomic loss of the condensate due to atom laser output leads to fluctuations of the laser pulses [88].
Up to now, in Wang’s laboratory, there are two groups led by Prof. Chen Xuzong and Prof. Zhou Xiaoji, respectively, and they have successfully prepared BECs on four platforms. In these systems, they have performed a lot of research, such as the atom laser, multi-component BEC, superradiance, and quantum simulation, one-dimensional gas and Bose–Fermi mixing. In 2008, [89] realized the superradiant Rayleigh scattering in an elongated Bose–Einstein condensate, where the pump laser pulse travels along the long axis. After that, the team conducted more in-depth research on superradiance. For example, they studied sequential scattering in superradiance from a Bose–Einstein condensate pumped by a two-frequency laser beam, which revealed that the frequency overlap between the end-fire modes related to different side modes plays an essential role in the dynamics of sequential superradiant scattering [90]. [91, 92] studied the high-order momentum modes and mode competition in the superradiant scattering of matter waves. [93] studied superradiant scattering from a Bose–Einstein condensate using a pump laser incident at a variable angle and showed the presence of asymmetrically populated scattering modes.
In 2002, [94] used the optical lattice to confine the BECs for the first time and observed the phase transition from a superfluid to a Mott insulator. Since then, BECs in optical lattices have gradually become a powerful tool for quantum simulation, quantum calculation, and precision measurement. Wang’s laboratory has conducted a lot of research on ultra-cold atoms in optical lattices. In 2010, Prof. Zhou Xiaoji, developed a shortcut method to quickly manipulate Bloch states of optical lattices with high fidelity [95]. The shortcut is composed of lattice pulse sequences imposed on the system before the lattice is switched on. The time duration and interval in each step are optimized in order to reach the target state with high fidelity and robustness. This process usually takes tens of seconds, which is much shorter than the traditional adiabatic loading process [95]. The idea of the shortcut to controlling Bloch states of optical lattices was first proposed in 2011 [96, 97]. At first, Liu Xinxing used a single light pulse, with conditions where the scattering of photons can be resonantly amplified by an atomic density grating, to explore multiband excitations of interacting Bose gases in a one-dimensional optical lattice [97]. Then they presented a scheme for nonadiabatically loading a Bose–Einstein condensate into the ground state of a one-dimensional optical lattice within a few tens of microseconds, which is much shorter than the traditional adiabatic loading time scale. This scheme is based on sequences of pulsed perturbations [96]. At the same time, Xiong Wei analyzed the effects of sequences of standing-wave pulses on a Bose–Einstein condensate in experiments and manipulated the momentum state of condensates by these sequences [98]. In 2013, Zhai Yueyang extended this method to load the atoms to the third band (D band) of the one-dimensional optical lattice and studied the collisional decay of atomic condensates in the D band [99]. Next, Wang Zhongkai successfully loaded atoms into the higher Bloch band, the G band. They were able to control the Bragg reflections at the Brillouin-zone edge up to the third order and observed quantum dynamical oscillations of ultra-cold atoms in the F and D bands of an optical lattice [100]. For the Bloch states at zero quasi-momentum in the ground band (S band), D band, and G band with even parity, the shortcut method is effective. However, this method cannot prepare atoms into odd parity states [95], such as the Bloch states in the second band (P band). In 2015, Hu Dong improved the method by using a shift pulse to load atoms into the P band and studied the long-time nonlinear dynamical evolution and quantum equilibration for P-band ultra-cold atoms in the optical lattice [101, 102]. In recent years, Chen and Zhou’s group have extended this shortcut method to two-dimensional and three-dimensional optical lattices, such as the square lattice and the triangular lattice [95, 103]. So far, this method can realize the preparation and manipulation of any Bloch state in any dimensional optical lattice. In 2016, Chen’ group achieved first 3D optical lattice in China and implemented quantum collapse and revival dynamics in 3D optical lattice [104, 105], they used the 3D lattice to form the one-dimensional gas and conducted serial experiments to study how an integrable system approaches thermal equilibrium [106, 107]. This is an interesting problem that has been puzzling everyone since D. Weiss’ famous Newton pendulum experiment was performed in 2006 [108].
Based on the method of manipulating BECs in optical lattices, the team carried out considerable research on quantum simulation, quantum precision measurement, and quantum computation. For example, [109] constructed a momentum filter for BECs based on a designed Talbot–Lau interferometer, which was an effective method for rapidly purifying the momentum width of atomic gas and has great potential for achieving ultra-low temperatures. [102] investigated the multiphoton process between different Bloch states in an amplitude-modulated optical lattice and realized a large-momentum-transfer beam splitter. [110] produced narrower momentum patterns by designing the shortcut pulses on a superposed state, and these narrower momentum modes were helpful in precision measurement and quantum control. [111] improved the band-mapping method to investigate the quantum phase transition from superfluid to Mott insulators and observed the critical behaviors of quantum phase transitions in both the dynamical steady-state-relaxation and the phase-oscillation regions. Based on various observables, two different values for the same quantum critical parameter were observed. This result was beyond a universal-scaling-law description of quantum phase transitions known as the Kibble–Zurek mechanism and suggests that multiple quantum critical mechanisms were completed in many-body quantum phase transition experiments in inhomogeneous systems [111]. BEC in optical lattice can also be used to simulate and prepare novel quantum states. For example, in 2018, [112] loaded the BECs into the P band at zero quasi-momentum of a one-dimensional optical lattice, which can be viewed as a series of “pancake”-shaped atomic samples. For this far-out-of-equilibrium system, they found an intermediate time window where the atomic ensemble exhibited superfluid phase coherence in the pancake directions but no coherence in the lattice direction, which implied a dynamical sliding phase superfluid. This experiment opened up a novel venue to search for exotic dynamical phases [112]. In 2021, [113] constructed a staggering hexagonal optical lattice and transferred BECs from the ground band into the second band. They observed a three-state quantum nematic order, dubbed “Potts-nematicity,” in a system described by an sp2-orbital hybridized model. This discovery paves the way to investigate quantum vestigial orders in multiorbital atomic superfluids [113]. The BECs in an optical lattice can also be used in quantum computation. For example, [114] proposed and realized an atom-orbital qubit by manipulating s and d orbitals of BECs in an optical lattice. They also achieved noise-resilient single-qubit gates by performing holonomic quantum control. This work was the first time for qubit based on the atom-orbitals in optical lattices and opened up broad opportunities for atom-orbital-based quantum information processing of vital importance to programmable quantum simulations of multiorbital physics in molecules and quantum materials [114].
Recently, BECs have also been used for precise measurements. Zhou’s group has constructed a compact BEC gravimeter based on the Ramsey–Bordé approach by two pairs of/2 Raman pulses to get a small volume [115]. Between the two pairs of/2 Raman pulses, the Bloch oscillation caused by the accelerated optical lattice was carried out. This BEC gravimeter is expected to reach sub-μGal with atoms falling a distance of 2 cm [115]. In addition, Zhou’s group is also building a BEC gyroscope based on the momentum states of the optical lattice. Under the same loop area, its theoretical sensitivity is much higher than that of the traditional gyroscope.
Optical tweezers
In 1986, A. Ashikin proposed the optical tweezers concept, that is, a single focused laser beam forms a three-dimensional optical well to trap a single biological particle such as single bacteria and viruses. The optical tweezers concept has many useful applications, and A. Ashikin was awarded the Noble prize for its invention in 2018. In the 1990s, as the leader of the laser trapping atom project, Professor Wang Yiqiu paid keen attention to laser tweezers technology and its potential application in biomedicine. He strongly advocated and supported the development of optical tweezers technology and application in his laboratory [116] and personally guided a graduate student Sun Wei to build a simple optical tweezers system using a wavelength of 852 nm semiconductor laser and successfully trapped a single cell. In 2000, Ye Anpei, a Professor of the same institute, inherited the research of optical tweezers and continued carrying out comprehensive studies on laser tweezers technology and its application. Ye’s work was greatly supported by the foundation of the Peking University Frontier Cross Fund project. Soon after, Prof. Ye established the Nanobiophotonics Group and focused on developing biomedical photonic techniques and applications in biological single molecule and single-cell detection. First, Ye made a great effort to develop the optical tweezers technology and set up a multi-function optical platform which included automatic dual-optical tweezers constructed using a 1064 nm CW solid laser and an inverted optical microscope. Soon, a high-precision four-quadrant photodetector (QPD) was successfully developed in the group, thus they achieved accurate position measurement with nanometer precision. Meanwhile, [117] calibrated the stiffness of optical tweezers based on an acousto-optic deflector and field programmable gate array, and therefore laid the foundation for quantitative measuring weak force of a single molecule. [118] built the first dynamic holographic optical tweezers (HOT) based on a commercial twisted-nematic liquid crystal display (TN-LCD) which was from a waste video projector, and therefore first provided easier and lower-cost access to the set-up of a HOT system which can manipulate multiple particles simultaneously. Ten years later, Prof. Ye’s group coupled optical tweezers and optical scissors together to construct a laser micro-manipulating workstation using Labview automatic control software that automatically controls each part of the entire platform with a computer, while the laser tweezers’ spatial resolution reached the sub-nanometer level. Furthermore, they coupled confocal micro-Raman spectroscopy to laser tweezers so that they formed a so-called laser tweezers-Raman spectral (LTRS) system. Therefore, Ye’s group can both trap single suspension living cells in vitro and meanwhile identify the type or state of the cell.
At the same time as technology development, Prof. Ye placed emphasis on its application in biomedical fields. Intermolecular interactions dominate the behavior of signal transduction in various physiological and pathological cellular processes. However, assessing these interactions remains a challenging task. Ye’s laboratory developed a single-molecule force spectroscopic method and analyzed cellular signal transduction with single-molecule force spectroscopy on a piconewton-scale, thus established single-molecule force spectroscopy as an effective platform for evaluating the piconewton-level interaction of signaling molecules and predicting the behavior outcome of signal transduction. For example, [119] investigated the activation of living monocytic U937 cells induced by interleukin-6 (IL-6) at the single cell level. [120] measured low affinity between IL-6 and IL-6R on living cells at a single molecular level, the affinity was 42.1 ± 11.7 pN at the loading rate of 3100 pN nm/s. Using the single-molecule force spectroscopy of RAS–RAF interaction in vitro, [121] illustrated the interaction conformation of RAF protein and its upstream G-protein (RAS) at a single-molecule level, they demonstrated that RAS has different numbers of binding domains between wild-type and mutant-type RAF. [122] measured single-molecule force via optical tweezers and revealed different kinetic features of two BRaf mutants responsible for cardio-facialcutaneous (CFC) syndrome. [123] investigated the specific single-interaction between apoA-I molecules and ABCA1 on living cells. [124] regulated cell migration in a myosin light chain kinase, a phosphorylation-independent mechanism regulatory light chain phosphorylation-independent mechanism. In addition, [125] investigated the mechanical property changes of red blood cells with the stored time and experimentally demonstrated that the deformability of red blood cells was impaired with the stored time.
The ability to discriminate between single cells in a label-free and noninvasive fashion is important for the classification of cells, especially to identify similar morphological cells from different origins. Ye’s group investigated single living cells and individual floating-particle using the homemade LTSR system, identified different types of single cells in aqueous media, and discriminated them among the same types of cells from different donors using a novel LTRS technique. [126] developed a novel anti-cancer drug sensitivity testing (DST) approach based on in vitro single-cell Raman spectrum intensity (RSI), which provided a powerful tool for simple and rapid detection of the sensitivity of tumor cells to anti-cancer drugs and the heterogeneity of their responses to these drugs. Also, [127] investigated drug resistance and heterogeneous characteristics of human gastric carcinoma cells (BGC823) under the treatment of paclitaxel (PTX) meanwhile, indicated that single-cell RS may be useful in systematically and dynamically characterizing the drug response of cancer cells at the single-cell level. [128] investigated chondrocyte dedifferentiation during in vitro proliferation. [129] reported laser-trapped metallic nanoparticles (NPs) as a novel approach for enhancing Raman spectroscopy in aqueous media. Recently, [130] combined LTRS and a specifically designed sample pool to achieve nondestructive identification and accurate isolation of single cells, which paves the pathway for multimodel analysis of a single cell. Furthermore, [131] reported a single-cell multimodal analytical approach by integrating Raman optical tweezers and RNA sequencing. Very recently, [132] realized fast label-free recognition of NRBCs by deep learning visual object detection and single-cell Raman spectroscopy, in order to rapidly and automatically analyze the single-cell Raman spectra based on AI techniques, Ye’s group meanwhile developed applied software, which can quickly and accurately identify the Raman spectroscope of a single cell, especially in single bacteria. For instance, [133] explored one-dimension convolutional neural networks (1CNNs) and applied them to identify marine microbes with single-cell Raman spectroscopy. [134] furtherly developed the CNN model using the residual network (ResNet) model and succeeded in rapidly and accurately identifying 15-species pathogenic bacteria with the accuracy of 94.53% at the single-cell level.
Moreover, Ye’s group recently developed an aerosol optical tweezers Raman spectroscopy technique and used it to levitate individual atmospheric aerosol droplets. For example, [135] realized single aerosol droplet capture for a long time without contact and simulated the hygroscopic-volatilization thermodynamic evolution process of aerosol droplets in the actual atmosphere via changing the ambient relative humidity around aerosol particles. By measuring the cavity resonance Raman signal of the single droplet particle and combining it with the appropriate physical models, [135] accurately measured the particle size, refractive index, diffusion coefficient, volatile flux, and other important physicochemical parameters of sodium chloride, sucrose, and citric acid in the hygroscopic-volatile process. Moreover, the effects of relative humidity on the hygroscopic-volatilization process for organic and inorganic aerosols as well as the possible phase transitions such as glassy and gel transitions are investigated. This work provides an important reference for understanding the hygroscopic-volatilization process of actual atmospheric aerosol. Also, [136] measured the viscosity of single suspended aerosol droplets under different RH conditions. They trapped and levitated a pair of quasi atmospheric aerosol droplets composed of organic and inorganic chemical substances by dual laser beams, respectively, and then collided and coalesced them. By recording the backscattering light signals and bright-field images of the dynamic coalescence process to infer the morphological relaxation time and the diameter of the composited droplet, and therefore measured the viscosity of the droplet. Furthermore, to give a deep insight into the relationship between viscosity and the mass transfer process, they researched the hygroscopicity of droplets with a solute of organic/inorganic mixtures. This work provided a robust approach for investigating the viscosity and hygroscopicity of actual individual liquid PM10 aerosols. Very recently, [136] investigated the pH-dependent liquid–liquid phase separation (LLPS) of a single artificial aerosol particle by self-developed optical tweezer-Raman spectroscopy (OTRS). This study provides the first comprehensive account of the pH-dependent LLPS in a single levitated microdroplet and brings a possible implication on phase separation in actual atmospheric aerosol particles.
Optical atomic clocks
In order to detect atomic transition spectral lines with a linewidth on the order of mHz, the linewidth of the local oscillator laser must be narrow enough. Generally, Pound–Drever–Hall frequency stabilization technology [137] is used to lock the frequency of the local oscillator laser to an ultra-stable optical resonator to obtain a highly coherent ultra-narrow-linewidth laser, but it is still limited by the thermal noise of the cavity length. In order to essentially solve the cavity length thermal noise problem of the resonator and with the support of Prof. Wang Yiqiu, the innovative concept of the active optical clock was proposed by Prof. Chen Jingbiao, who was formerly a doctoral student of Prof. Yang Donghai. In 2005, the concept of the active optical clock was formally proposed [138–140]. Active optical clock forms of polyatomic coherent stimulated radiation between atomic transition energy levels in the optical resonator. The stimulated radiation of the quantum reference system can be directly used as the clock laser signal, which can break through the limit of the Schawlow–Townes quantum linewidth [79]. In April 2013, the stimulated emission output of the active optical clock transition in the deep bad cavity regime (cavity mode linewidth-gain linewidth proportional coefficient a≫1) was experimentally realized for the first time [141]. In the experiment, using a 455 nm laser as the pumping laser, atomic population inversion was realized on the 1469.9 nm clock transition line of the Cs atom four-level active optical clock. The research team further experimentally measured the cavity-pulling coefficient of the four-level active optical clock up to 40, and the output laser linewidth was 590 Hz [142]. In the four-level active optical clock scheme, the frequency standard used as a quantum reference and the gain medium for stimulated emission are all from the same atomic system. Since it is difficult to experimentally achieve stimulated emission output using both long-lived atomic energy levels and bad cavity structures, this requirement greatly limits the quantum systems that can be used for the active optical frequency standard. In principle, the Faraday active optical clock separates the gain medium from the frequency reference. The semiconductor laser provides a larger gain, and the ultra-narrow bandwidth Faraday atomic filter provides the frequency reference to realize the optical frequency standard signal. The output laser linewidth obtained experimentally is 281 Hz, which is 19000 times narrower than the natural linewidth of the 852 nm transition of the Cs atom [143]. In order to eliminate the influence of the residual cavity-pulling effect on the active optical frequency standard, the dual-wavelength good-bad-cavity scheme was carried out [144]. In the experiment, two sets of dual-wavelength good-bad-cavity systems were built, and the phase locking of the good cavity laser was used to reduce the influence of the asynchronous cavity-length variation between two dual-wavelength laser systems on the linewidth broadening of the 1470 nm clock laser. The linewidth of the realized 1470 nm active optical clock signal reached the level of Hz. In theory, [145] demonstrated the state-of-the-art technique of an active clock to provide a continuous superradiant lasing signal using an ensemble of trapped Cs atoms in an optical lattice, which can achieve a fractional uncertainty in the clock frequency of about 10–15 and a linewidth of a few mHz.
After the active optical clock scheme was proposed, it attracted the attention of international counterparts. Several research groups have carried out experimental verifications of it and conducted research on active optical clock schemes based on different atomic systems. The Niels-Bohr Institute (University of Copenhagen, Denmark) used the 88Sr atom trapped in the magneto-optical trap working in the bad cavity region as the quantum reference and realized a pulsed superradiant light [146]. The JILA research group realized a superradiant light emitted from the ultra-narrow, 1-mHz-linewidth optical clock transition in an ensemble of cold 87Sr atoms. They observed a fractional Allan deviation of 6.7 × 10–16 at 1 s of averaging, established absolute accuracy at the 2 Hz (4 × 10–15 fractional frequency) level, and demonstrated insensitivity to key environmental perturbations [147]. Using the two-level scheme, the University of Hamburg observed a superradiant emission of hyperbolic secant-shaped pulses into the cavity with an intensity proportional to the square of the atom number based on the 657 nm transition of cold Ca atoms [148]. [149] investigated the full model for the collective decay process and aimed at direct implications for the laser linewidth of a magic wavelength lattice laser in the superradiant regime. Using the Raman transition of the cold 87Sr atom in bad cavity, JILA obtained a superradiant laser, in which FWHM of the Gaussian fitting was 350 Hz and the FWHM of the Lorentzian fitting was 4.5 Hz [150]. Based on the narrow quantum transition line of the 87Sr atom, the active optical frequency standard of the order of mHz can be realized theoretically [151]. In terms of theoretical research, the Department of Physics and Astronomy of Aarhus University in Denmark analyzed the 87Sr atoms trapped in the one-dimensional optical lattice in the bad cavity region. Under the action of a magnetic field, this system can output superradiant pulsed light with a linewidth of less than 2 Hz [152]. [153] studied two-frequency-detuned active atomic clocks which are coupled in a cascaded setup and demonstrated that synchronization still occurs in cascaded setups but exhibits distinctly different phase diagrams. Georgy A. [154] investigated the spectral properties and the stability of active clocks, including homogeneous and inhomogeneous broadening effects. By using realistic numbers for an active clock with 87Sr, it is possible to realize an optimized stability of σy (τ)≈4 × 10–18/√τ [154]. The active optical clock is gaining more international attention. Specifically, at the 2015 IEEE International Frequency Control Symposium, the active optical clock was listed as one of the three most talked about emerging technologies in this field.
In addition to the active optical clock, many of Professor Wang Yiqiu’s ideas, such as Cs beam tube optical frequency standards and Ca atom compact optical clocks, have been realized. For the cesium beam tube optical frequency standard, [155] reported a compact optical clock scheme based on Cs atomic beam. Under the condition of a 1.4 MHz narrow-linewidth laser spectrum and 100,000 signal-to-noise ratio (SNR), the limited stability of the compact Cs optical clock is expected to be 2.2 × 10–14 at 1 s. The performance of this Cs optical clock is superior to that of the hydrogen atomic clock. For the Ca atom compact optical clock, [156] implemented a miniaturized Ca beam optical frequency standard whose fractional instability reached 1.8 × 10–15 after 1600 s of averaging. It was the first time to realize the optical frequency standard using a fully-sealed vacuum tube. For the Cs atom compact optical clock, using the modulation transfer spectrum stabilization technique with a high signal-to-noise ratio, the clock laser was directly locked on the transition spectrum line of the Cs atom, and a compact high-performance Cs atomic optical frequency standard with a frequency stability of 1.4 × 10–14/√τ was developed [157]. Then, the 1470 nm active optical frequency standard was realized by using this small optical frequency standard as the pumping laser, which can go beyond the limits of the Schawlow–Townes formula and has the advantage of the suppressed cavity-pulling effect.
Other contributions by Prof. Wang
“The Principles of Quantum Frequency Standards,” the first book on the principle of frequency standard in the world, was written by Wang Yiqiu in 1986 [24]. This book mainly describes the physical principles of quantum frequency standards and includes new achievements at home and abroad, which promotes the research work on the quantum frequency standard and the development of quantum metrology. In the field of laser cooling and trapping of atoms, there was a fundamental and comprehensive book of “Laser Cooling and Trapping of Atoms” also written by Wang Yiqiu (published at the beginning of the new century by Peking University Press). In addition to the aforementioned two important monographs, Wang Yiqiu translated the first and second volumes of the French original book “Atomic Physics,” providing an excellent textbook for teachers and students of atomic and molecular physics, optics, quantum electronics, and other majors. Furthermore, under the personal attention of Prof. C. Cohem-Tannoudji, Prof Wang Yiqiu organized the translation of Cohem-Tannoudji’s famous book (with Guéry-Odelin) of “Advances in Atomic Physics, an Overview” in Chinese and made the compilation and revision by himself. Figure 8 shows several books written or translated by Prof. Wang Yiqiu.
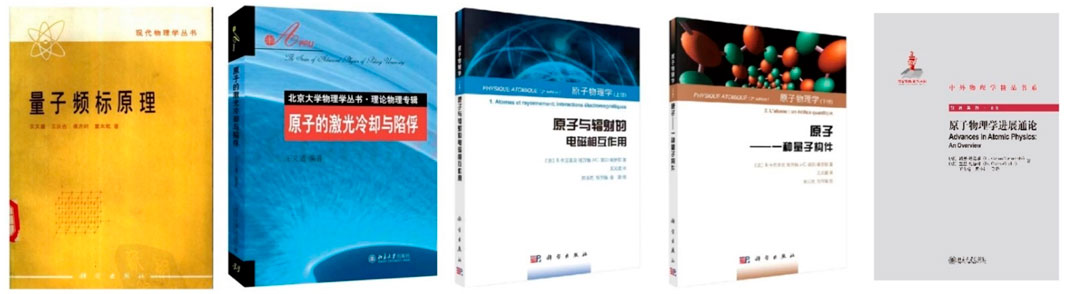
FIGURE 8. Books written or translated by Prof. Wang Yiqiu. From left to right: “The Principles of Quantum Frequency Standards,” “Laser Cooling and Trapping of Atoms,” “Atomic Physics: Volume 1” (originally in French), “Atomic Physics: Volume 2” (originally in French), and “Advances in Atomic Physics: an Overview” (originally in French).
Conclusion
This review gives an account of the scientific career and contributions of Prof. Wang Yiqiu, who is a prominent figure in China’s research on quantum precision measurement and cold atom physics. During his long scientific career, Prof. Wang has contributed greatly to the fields of research such as nuclear magnetic resonance, microwave atomic clocks, laser cooling of atoms, Bose–Einstein condensate, optical tweezers, and optical atomic clocks. We list the major scientific achievement by Prof. Wang, as well as advances in the relevant research fields inspired by Prof. Wang’s work, roughly in a chronological order.
Author contributions
XC wrote the sections “Nuclear Magnetic Resonance” and “Laser cooling,” and participated in the writing of the section “Microwave Atomic Clocks;” XZ wrote the section “Bose–Einstein Condensate;” AY wrote the section “Optical Tweezers;” YW participated in the writing of the section “Microwave Atomic Clocks;” JC wrote the abstract, introduction, conclusion, the sections “Optical Atomic Clocks” and “other contributions by Prof. Wang,” and participated in the writing of the section “Microwave Atomic Clocks.”
Funding
The work was supported by the National Natural Science Foundation of China (Grants Nos. 11920101004, 11334001, 61727819, 61475007), and the National Key Research and Development Program of China (Grant No. 2021YFA1400900, 2021YFA0718300).
Conflict of interest
The authors declare that the research was conducted in the absence of any commercial or financial relationships that could be construed as a potential conflict of interest.
Publisher’s note
All claims expressed in this article are solely those of the authors and do not necessarily represent those of their affiliated organizations, or those of the publisher, the editors, and the reviewers. Any product that may be evaluated in this article, or claim that may be made by its manufacturer, is not guaranteed or endorsed by the publisher.
References
3. Lauterbur PC. Anisotropy of theC13Chemical shift in calcite. Phys Rev Lett (1958) 1:343–4. doi:10.1103/physrevlett.1.343
4. Scripov FI, Wang Y, 20. USA (1964). p. 41. (in Chinese); also in “Chinese Physics” in.Acta Physica Sinica
6. Deverell C, Schaumburg K, Bernstein HJ. 19F nuclear magnetic resonance chemical shift of alkali fluorides in light‐ and heavy‐water solutions. J Chem Phys (1968) 49(3):1276–83. doi:10.1063/1.1670220
7. Vaughan RW, Elleman DD, Rhim WK, Stacey LM. 19F chemical shift tensor in group II difluorides. J Chem Phys (1972) 57(12):5383–90. doi:10.1063/1.1678236
8. Sears REJ. Fluorine chemical shifts in the alkali fluorides. J Chem Phys (1974) 61(10):4368–9. doi:10.1063/1.1681750
9. Burum DP, Elleman DD, Rhim WK. A multiple pulse zero crossing NMR technique, and its application to 19F chemical shift measurements in solids. J Chem Phys (1978) 68(3):1164–9. doi:10.1063/1.435806
10. Ramsey NF. Magnetic shielding of nuclei in molecules. Phys Rev (1950) 78:699–703. doi:10.1103/physrev.78.699
11. Kondo J, Yamashita J. Nuclear quadrupolar relaxation in ionic crystals. J Phys Chem Sol (1959) 10:245–53. doi:10.1016/0022-3697(59)90001-0
13. Deverell C. Chapter 4 Nuclear magnetic resonance studies of electrolyte solutions. Prog Nucl Magn Reson Spectrosc (1969) 4:235–334. doi:10.1016/0079-6565(69)80005-1
14.Author Anonymous. Proposal for atomic cooling approach in space and a cold atom experimental system in Chinese space station. Noordwijk, Netherlands: Chen X,book of abstract (2013). p. 22–3. page 11, STE-QUEST Workshop.
15. Lu W, Peng Z, Xu-Zong C, Zhao-Yuan M. Generating a picokelvin ultracold atomic ensemble in microgravity. J Phys B: Mol Opt Phys (2013) 46:195302. doi:10.1088/0953-4075/46/19/195302
16. Hepeng Y, Tian L, Chen L, Yin Z, Zhaoyuan M, Xuzong C. Comparison of different techniques in optical trap for generating picokelvin 3D atom cloud in microgravity. Opt Commun (2016) 359:123. doi:10.1016/j.optcom.2015.09.065
17. Luan T, Yao H, Wang L, Li C, Yang S, Chen X, et al. Two-stage crossed beam cooling with 6Li and 133Cs atoms in microgravity. Opt Express (2015) 23:11378. doi:10.1364/OE.23.011378
18. Tian L, Yufan L, Zhang X, Chen X, Xuesong Z, Xuzong C. Realization of two-stage crossed beam cooling and the comparison with Delta-kick cooling in experiment. Rev Scientific Instr (2018) 89:123110. doi:10.1063/1.5046815
19. Bo F, Luheng Z, Yin Z, Jingxin S, Wei X, Jinqiang C, et al. Numerical study of evaporative cooling in the space station. J Phys. B: Mol. Opt. Phys. (2021) 54:015302. doi:10.1088/1361-6455/abc72d
20. Xuzong C, Bo F. The emergence of picokelvin physics. Rep Prog Phys (2020) 83:076401. doi:10.1088/1361-6633/ab8ab6
21. Wang X, Zhang C, Wang Y. Proc. Of the national conf. Of optical and radio frequency spectroscopy. ShanghaiBeijiing: Chinese Physical Society (1965).
22. Ramsey NF. History of atomic clocks. J Res Natl Bur Stand (1977) (1983) 88:301–20. doi:10.6028/jres.088.015
23. Ramsey NF. Experiments with separated oscillatory fields and hydrogen masers. Science (1990) 248:1612–9. doi:10.1126/science.248.4963.1612
24. Wang Y, Wang Q, Fu J, Dong T. Principles of quantum frequency standards. Beijing: Science Press (1986).
26. Yi-qiu W. The causes of Majorana transitions in one type of small cesium beam tube. Acta Metrologica Sinica (1983) 4:86–95.
27. Bauch A, Schröder R. Frequency shifts in a cesium atomic clock due to Majorana transitions. Ann Phys (1993) 2:421–49. doi:10.1002/andp.19935050502
28. Wynands R, Schroder R, Weyers S. Majorana transitions in an atomic fountain clock. IEEE Trans Instrum Meas (2007) 56(2):660–3. doi:10.1109/tim.2007.891116
29. Wang Y. A proposal for a laser deceleration atomic standard. Chin J Magn Reson (1988) 5(1):1. in Chinese.
30. Wang Y, Li Y, Gan J, Chen X, Yang D. Some considerations on the cesium atomic fountain standard. Proc Intern Freq Stand Metrology, Woods Hole (1995) 74–80.
31. Xin-xin W, Yue-ting X, Kun-yu T, Shu-tong Y, Lin-zhen X, Yi-qiu W. Main aspects in the design of optically pumped cesium beam tube. Acta Metrologica Sinica (1985) 6:15–23.
32. Wang DP, Xie LZ, Wang YQ, Guo SJ. GaAlAs laser diode pumped cesium beam frequency standard. Chin Phys Lett (1988) 5(3):97. doi:10.1088/0256-307X/5/3/001
33. Wang YQ, Xie LZ, Wang DP, Yao ST. Preliminary results and some considerations on the optically pumped Cs beam frequency standard. Proc 4th Symp Frequency Stand Metrology (1988) 126–31. doi:10.1007/978-3-642-74501-0_23
34. Yang DH, Wang YQ. Detection by cycling transition in an optically pumped cesium beam frequency standard. Opt Commun (1990) 73:285–8. doi:10.1016/0030-4018(89)90218-6
35. Yang DH, Wang YQ. Preliminary results of an optically pumped cesium beam frequency standard at Peking University. IEEE Trans Instrum Meas (1991) 40(6):1000–2. doi:10.1109/19.119781
36. Zhang J, Chen J, Wang F, Yang D, Wang Y. Influence of a static magnetic field on the detected atomic velocity distribution in an optically pumped cesium beam frequency standard. IEEE Trans Ultrason Ferroelectr Freq Control (2003) 50(9):1210–3. doi:10.1109/tuffc.2003.12353339
37. Yang D-H, Wang Y-Q. A novel design of optically pumped cesium beam frequency standards. In: Proceedings of the Conference on Precision Electromagnetic Measurements; 11-14 June 199; Ottawa, ON, Canada (1990). p. 244–5.
38. Yang DH, Wang YQ. The effective velocity distribution of atoms and the improvement of the accuracy for an optically pumped cesium beam frequency standard with sharp angle incidence of probing laser beam. Opt Commun (1991) 85:319–25. doi:10.1016/0030-4018(91)90484-u
39. Dong-Hai Y, En-Xian L, Le-Ming C, Bing-Ying H, Yi-Qiu W, Wang YQ. Experiment on optically pumped cesium beam frequency standard with sharp angle incidence probing laser beam. Opt Commun (1991) 84:275–8. doi:10.1016/0030-4018(91)90086-s
40. Dong-Hai Y, Yi-Qiu W. Long-term operation and frequency stability of an optically pumped cesium beam frequency standard with sharp-angle incidence of probing laser beam. Jpn J Appl Phys (2008) (1994) 33:1674–5. doi:10.1143/jjap.33.1674
41. de Clercq E, Rovera GD, Bouzid S, Clairon A. The LPTF optically pumped primary frequency standard. IEEE Trans Instrum Meas (1993) 42:457–61. doi:10.1109/19.278604
42. Lee HS, Yang SH, Kim JO, Kim YB, Baek KJ, Oh CH, et al. Frequency stability of an optically pumped caesium-beam frequency standard at the KRISS. Metrologia (1998) 35:25–31. doi:10.1088/0026-1394/35/1/5
43. McFerran JJ, Luiten AN. Fractional frequency instability in the 10^−14 range with a thermal beam optical frequency reference. J Opt Soc Am B (2010) 27:277–85. doi:10.1364/josab.27.000277
44. Wang F, Yang D, Wang Y. A portable diode laser frequency locking system for optically pumped cesium beam frequency standard. Acta Metrologica Sinica (1994) 15:155–8.
45. Yang DH, Wang FZ, Wang YQ. A compact diode laser system for small optically pumped cesium beam frequency standard. In: Proceedings of the 50th IEEE International Frequency Control Symposium; 05-07 June 1996; Honolulu, HI, USA (1996).
46. Liu C, Zhou S, Wang Y, Hou S. Rubidium-beam microwave clock pumped by distributed feedback diode lasers. Chin Phys B (2017) 26:113201. doi:10.1088/1674-1056/26/11/113201
47. Liu C, Wang S, Chen Z, Wang Y, Hou S. Optical detection in magnetic state-selection Cs beam tubes for transportable Cs beam clocks. Meas Sci Technol (2019) 30:075004. doi:10.1088/1361-6501/ab11af
48. Xing-wen Z, Qiang W, Dong-xu L, Yu-ao L, Lin Y. Progress on optically pumped cesium beam frequency standard. J Time Frequency (2021) 45:1–8.
49. Chen J, Ma P, Wang J, Guo L, Tu JH, Yang W. Test of magnetic-selected cesium atomic clock LIP Cs3000C. J Time Frequency (2018) 41(3):1674–0637.
50. Chen J, Ma P, Wang J, Guo L, Liu ZD, Yang J, et al. Progress in commercialization of compact magnetically selected cesium atomic clocks. J Astronautic Metrology Meas (2020) 40:12–6.
51. Xie W, Wang Q, He X, Chen N, Xiong Z, Fang S, et al. Frequency instability of a miniature optically pumped cesium-beam atomic frequency standard. Rev Scientific Instr (2020) 9:074705. doi:10.1063/5.0001749
52. Shang H, Zhang T, Miao J, Shi T, Pan D, Zhao X, et al. Laser with 10−13 short-term instability for compact optically pumped cesium beam atomic clock. Opt Express (2020) 28:6868. doi:10.1364/oe.381147
53. Liu T, Pan D, Chen J. Optical pumped cesium atomic clock with multi-pole magnet. In: Proceedings of the 2020 Joint Conference of the IEEE International Frequency Control Symposium and International Symposium on Applications of Ferroelectrics (IFCS-ISAF); 19-23 July 2020; Keystone, CO, USA (2020). p. 1–4.
54. Yan Y, Chen H, Feng J, Pan D, Liu T, Chen J. Enhancing the signal-to-noise ratio in optically pumped cesium beam tubes using a hexapole magnetic system. In: Proceedings of the 2021 Joint Conference of the European Frequency and Time Forum and IEEE International Frequency Control Symposium (EFTF/IFCS); 07-17 July 2021; Gainesville, FL, USA (2021). p. 1–7.
55. Chen X, Wang Q, Yu J, Zhao W, Zhang Y, Qi X, et al. Theoretical analysis on optical pumped cesium atomic clock. Acta Metrology Sinica (2015) 36:108.
56. Wang Q, Qi X, Liu S, Yu J, Chen X. Laser frequency stabilization using a dispersive line shape induced by Doppler Effect. Opt Express (2015) 23:2982. doi:10.1364/oe.23.002982
57. Xie WB, Wang Q, He X, Xiong ZZ, Chen N, Fang SW, et al. A linewidth locking method to control the microwave power in optically pumped cesium-beam clocks. Rev Sci Instrum (2020) 91:094708. doi:10.1063/1.5144478
58. Xie WB, Wang Q, He X, Chen N, Xiong ZZ, Fang SW, et al. Frequency instability of a miniature optically pumped cesium-beam atomic frequency standard. Rev Sci Instrum (2020) 91:074705. doi:10.1063/5.0001749
59. He X, Fang S, Yuan Z, Xie W, Chen N, Xiong Z, et al. Compact optically pumped cesium beam atomic clock with a 5-day frequency stability of 7×10−15. Appl Opt (2021) 60:10761–5. Editors’ Pick. doi:10.1364/ao.443812
60. He X, Wang Q, Yuan Z, Qi X, Chen X. Observation of a hole in the laser-induced cesium beam spectrum. IEEE Access (2021) 9, 49974–9. doi:10.1109/access.2021.3069031
61. Liu C, Wang S, Chen Z, Wang Y, Hou S. Optical detection in magnetic state-selection Cs beam tubes for transportable Cs beam clocks[J]. Measurement Science and Technology, United Kingdom, 2019.
62. Yimin L, Xuzong C, Jianhua G, Wangxi J, Jingshan H, Donghai Y, et al. Intensity dependence of Cs D2-line saturation spectra. Chin Phys.Lett (1996) 13(6):424. doi:10.1088/0256-307X/13/6/007
63. Gan J, Chen X, Liyimin JW, Hua J, Yao J, Yang D, et al. The dependence of Cs saturated absorption spectra on the intensity of pumping light, Acta Physica Sinica (1996) 45(10), 1622–8.
64. Chen X, Yao J, Li Y, Yang D, Wang Y. A narrow linewidth diode laser for high resolution spectroscopy and its properties. Acta Optica Sinica (1996) 10.
65. Wang X, Chen X, Hou J, Yang D, Wang Y. Side-mode injection locking characteristics of 150 mW AlGaAs semiconductor lasers. Opt Commun (2000) 178:165–73. doi:10.1016/s0030-4018(00)00617-9
66. Wang X, Chen X, Hou J, Yang D, Wang Y. Theoretic and experimental study on large-frequency-difference side-mode infection locking of high power semiconductor lasers of laser cooling. Acta Physica Sinica (2000) 49:85–93.
67. Gan J, Li Y, Chen X, Liu H, Yang D, Wang Y. Magneto-optical trap for Cs atoms. Chin.Phys.Lett. (1996) 13(11):821. doi:10.1088/0256-307X/13/11/006
68. Fu J, Li Y, Chen X, Yang D, Wang Y. Experimental study on the properties of magneto optical trap and the collective behaviors of cold atoms. Acta Sinica Puantum Optica (1998) 03.
69. Hou J, Wang X, Chen X, Yang D, Wang Y. Obtaining ultra-cold atoms by optical molasses directly from vapor. Acta Scientiarum Naturalium Universitatis Pekinensis (2001) 37(5):716–20.
70. Wang X, Hou J, Chen X, Yang D, Wang Y. Design and setting up of a practical optical system for cesium atomic fountain. Acta Optica Sinica (2000) 11.
71. Fu J, Li Y, Chen X, Yang D, Wang Y. A new method for measuring density and temperature of cold atoms in magneto-optical trap. Acta Optica Sinica (2001) 414–6.
72. Fu J, Chen S, Chen X, Yang D, Wang Y. Realization of an atomic fountain using a novel launching method. Chin Phys Lett (2001) 18:1457–9. doi:10.1088/0256-307x/18/11/310
73. Tino1 GM, Angelo Bassi A, Bianco G, Bongs K, Bouyer P, Cacciapuoti L, et al. SAGE, A proposal for a space atomic gravity explorer. Eur Phys J (2019) D73:22. doi:10.1140/epjd/e2019-100324-6
74. Jin S, Guo X, Peng P, Chen X, Li X, Zhou X. Finite temperature phase transition in a cross-dimensional triangular lattice. New Jou Phys (2019) 21:073015. doi:10.48550/arXiv.1805.08790
75. Bose SN. Plancks Gesetz und Lichtquantenhypothese. Z Physik (1924) 26:178–81. doi:10.1007/bf01327326
76. Einstein A. Quantentheorie des einatomigen idealen Gases. Sitzungsber Kgl Preuss Akad Wiss (1924) XXII:261. doi:10.1002/3527608958.ch27
77. Anderson MH, Ensher JR, Matthews MR, Wieman CE, Cornell EA. Observation of bose-einstein condensation in a dilute atomic vapor. Science (1995) 269:198–201. doi:10.1126/science.269.5221.198
78. Davis KB, Mewes MO, Andrews MR, van Druten NJ, Durfee DS, Kurn DM, et al. Bose-einstein condensation in a gas of sodium atoms. Phys Rev Lett (1995) 75:3969–73. doi:10.1103/physrevlett.75.3969
79. Shuai C, Xiao-Ji Z, Fan Y, Lin X, Yi-Qiu W, Xu-Zong C. Optimization of the loading process of the QUIC magnetic trap for the experiment of bose–einstein condensation. Chin Phys Lett (2004) 21:2227–30. doi:10.1088/0256-307x/21/11/042
80. Shuai C, Xiao-Ji Z, Fan Y, Lin X, Ya-Ya S, Yi-Qiu W, et al. Analysis of runaway evaporation and bose–einstein condensation by time-of-flight absorption imaging. Chin Phys Lett (2004) 21:2105–8. doi:10.1088/0256-307x/21/11/009
81. Fan Y, Lin X, Xiao-Ji Z, Xiu-Quan M, Xu-Zong C. Strong outcoupling from spin-2 87Rb Bose{Einstein condensates. Chin Phys Lett (2005) 22:1596.
82. Yiqiu W, Xiaoji Z, Donghai Y. Realization of the long-term continuous output for an atom laser: A proposal. In: Z Xu, S Xie, S-Y Zhu, and MO Scully, editors. Frontiers of laser physics and quantum optics. Berlin, Heidelberg: Springer Berlin Heidelberg (2000). p. 473–6.
83. Xiao-Ji Z, Yi-Qiu W, Dong-Hai Y. A scheme for realizing the continuous wave atom laser. Chin Phys Lett (2000) 17:784–6. doi:10.1088/0256-307x/17/11/002
84. Chikkatur AP, Shin Y, Leanhardt AE, Kielpinski D, Tsikata E, Gustavson TL, et al. A continuous source of bose-einstein condensed atoms. Science (2002) 296:2193–5. doi:10.1126/science.296.5576.2193
85. Ma X, Xia L, Yang F, Zhou X, Wang Y, Guo H, et al. Population oscillation of the multicomponent spinor Bose-Einstein condensate induced by nonadiabatic transitions. Phys Rev A (Coll Park) (2006) 73:013624. doi:10.1103/physreva.73.013624
86. Xia L, Xu X, Guo R, Yang F, Xiong W, Li J, et al. Manipulation of the quantum state by the Majorana transition in spinor Bose-Einstein condensates. Phys Rev A (Coll Park) (2008) 77:043622. doi:10.1103/physreva.77.043622
87. Lin X, Wei X, Fan Y, Lin Y, Xiao-Ji Z, Xu-Zong C. High field seeking state atom laser and properties of flux. Chin Phys Lett (2008) 25(7):2374. doi:10.1088/0256-307x/25/7/012
88. Xia L, Yang F, Zhou X, Chen X. Intensity fluctuations of the 87Rb pulsed atom laser. Phys Lett A (2009) 373:1429–33. doi:10.1016/j.physleta.2009.02.042
89. Li J, Zhou X, Yang F, Chen X. Superradiant Rayleigh scattering from a Bose–Einstein condensate with the incident laser along the long axis. Phys Lett A (2008) 372:4750–3. doi:10.1016/j.physleta.2008.04.049
90. Yang F, Zhou X, Li J, Chen Y, Xia L, Chen X. Resonant sequential scattering in two-frequency-pumping superradiance from a Bose-Einstein condensate. Phys Rev A (Coll Park) (2008) 78:043611. doi:10.1103/physreva.78.043611
91. Zhou X, Fu J, Chen X. High-order momentum modes by resonant superradiant scattering. Phys Rev A (Coll Park) (2009) 80:063608. doi:10.1103/physreva.80.063608
92. Vogt T, Lu B, Liu X, Xu X, Zhou X, Chen X. Mode competition in superradiant scattering of matter waves. Phys Rev A (Coll Park) (2011) 83:053603. doi:10.1103/physreva.83.053603
93. Lu B, Zhou X, Vogt T, Fang Z, Chen X. Laser driving of superradiant scattering from a Bose-Einstein condensate at variable incidence angle. Phys Rev A (Coll Park) (2011) 83:033620. doi:10.1103/physreva.83.033620
94. Greiner M, Mandel O, Esslinger T, Hänsch TW, Bloch I. Quantum phase transition from a superfluid to a Mott insulator in a gas of ultracold atoms. Nature (2002) 415:39–44. doi:10.1038/415039a
95. Zhou X, Jin S, Schmiedmayer J. Shortcut loading a Bose-Einstein condensate into an optical lattice. New J Phys (2018) 20:055005. doi:10.1088/1367-2630/aac11b
96. Liu X, Zhou X, Xiong W, Vogt T, Chen X. Rapid nonadiabatic loading in an optical lattice. Phys Rev A (Coll Park) (2011) 83:063402. doi:10.1103/physreva.83.063402
97. Liu X, Zhou X, Zhang W, Vogt T, Lu B, Yue X, et al. Exploring multiband excitations of interacting Bose gases in a one-dimensional optical lattice by coherent scattering. Phys Rev A (Coll Park) (2011) 83:063604. doi:10.1103/physreva.83.063604
98. Xiong W, Yue X, Wang Z, Zhou X, Chen X. Manipulating the momentum state of a condensate by sequences of standing-wave pulses. Phys Rev A (Coll Park) (2011) 84:043616. doi:10.1103/physreva.84.043616
99. Zhai Y, Yue X, Wu Y, Chen X, Zhang P, Zhou X. Effective preparation and collisional decay of atomic condensates in excited bands of an optical lattice. Phys Rev A (Coll Park) (2013) 87:063638. doi:10.1103/physreva.87.063638
100. Wang Z, Yang B, Hu D, Chen X, Xiong H, Wu B, et al. Observation of quantum dynamical oscillations of ultracold atoms in theFandDbands of an optical lattice. Phys Rev A (Coll Park) (2016) 94:033624. doi:10.1103/physreva.94.033624
101. Hu D, Niu L, Yang B, Chen X, Wu B, Xiong H, et al. Long-time nonlinear dynamical evolution forP-band ultracold atoms in an optical lattice. Phys Rev A (Coll Park) (2015) 92:043614. doi:10.1103/physreva.92.043614
102. Niu L, Hu D, Jin S, Dong X, Chen X, Zhou X. Excitation of atoms in an optical lattice driven by polychromatic amplitude modulation. Opt Express (2015) 23:10064–74. doi:10.1364/oe.23.010064
103. Guo X, Yu Z, Peng P, Yin G, Jin S, Chen X, et al. Dominant scattering channel induced by two-body collision of D-band atoms in a triangular optical lattice. Phys Rev A (Coll Park) (2021) 104:033326. doi:10.1103/physreva.104.033326
104. Li C, Zhou T, Zhai Y, Yue X, Xiang J, Yang S, et al. Optical Talbot carpet with atomic density gratings obtained by standing-wave manipulation. Phys Rev A (2017) 95:033821. doi:10.1103/PhysRevA.95.033821
105. Zhou T, Yang K, Zhu Z, Yu X, Yang S, Xiong W, et al. Observation of atom-number fluctuations in optical lattices via quantum collapse and revival dynamics. Phys Rev A (Coll Park) (2019) 99:013602. doi:10.1103/physreva.99.013602
106. Chen L, Zhou T, Mazets I, Hans-Peter S, Frederik M, Zijie Z, et al. Relaxation of bosons in one dimension and the onset of dimensional crossoverSciPost. Phys (2020) 9:058. doi:10.21468/SciPostPhys.9.4.058
107. Frederik M, Chen L, Igor M, Hans-Peter S, Tianwei Z, Zijie Z, et al. Extension of the generalized hydrodynamics to the dimensional crossover regime. Phys Rev Lett (2021) 126:090602. doi:10.1103/physrevlett.126.090602
109. Xiong W, Zhou X, Yue X, Zhai Y, Chen X. A momentum filter for atomic gas. New J Phys (2013) 15:063025. doi:10.1088/1367-2630/15/6/063025
110. Yang B, Jin S, Dong X, Liu Z, Yin L, Zhou X. Atomic momentum patterns with narrower intervals. Phys Rev A (Coll Park) (2016) 94:043607. doi:10.1103/physreva.94.043607
111. Huang Q, Yao R, Liang L, Wang S, Zheng Q, Li D, et al. Observation of many-body quantum phase transitions beyond the kibble-zurek mechanism. Phys Rev Lett (2021) 127:200601. doi:10.1103/physrevlett.127.200601
112. Niu L, Jin S, Chen X, Li X, Zhou X. Observation of a dynamical sliding phase superfluid with P-band bosons. Phys Rev Lett (2018) 121:265301. doi:10.1103/physrevlett.121.265301
113. Jin S, Zhang W, Guo X, Chen X, Zhou X, Li X. Evidence of potts-nematic superfluidity in a hexagonal sp2 optical lattice. Phys Rev Lett (2021) 126:035301. doi:10.1103/physrevlett.126.035301
114. Shui H, Jin S, Li Z, Wei F, Chen X, Li X, et al. Atom-orbital qubit under nonadiabatic holonomic quantum control. Phys Rev A (Coll Park) (2021) 104:L060601. doi:10.1103/physreva.104.l060601
115. Dong X, Jin S, Shui H, Peng P, Zhou X. Improve the performance of interferometer with ultra-cold atoms. Chin Phys B (2021) 30:014210. doi:10.1088/1674-1056/abcf33
116. Ji W, Wang Y. Recent developments in the application of optical tweezers. Physics (1996) 25(12):707–12.
117. Song Q, Wen C, Zhang Y, Wang G, Ye A. Calibration of optical tweezers based on acousto-optic deflector and field programmable gate array. Chin Opt Lett (2008) 6(8):600–2. doi:10.3788/col20080608.0600
118. Wang G, Wen C, Ye A. Dynamic holographic optical tweezers using a twisted-nematic liquid crystal display. J Opt A: Pure Appl Opt (2006) 8:703–8. doi:10.1088/1464-4258/8/8/013
119. Ye A, Zhang Y. Micro-Raman spectroscopy of optically trapped single cell, 2009 international conference on optical instruments and technology: Optical trapping and microscopic imaging. Proc SPIE (2009) 7507:750705–1. doi:10.1117/12.838232
120. Ye A, Yuan F, Wen C, Zhang Y. Single-molecule force measurement of IL-6 and IL6-receptor interaction in living cells. Biophys. J. Jan (2008):350a.
121. Shu H, Cheng W, Ye A. Analysis of RAS-RAF interaction domains by single-molecule force spectroscopy measurements. ACTA BIOPHYSICA SINA (2011) 27(11):941–7.
122. Wen C, Ye A. single-molecule force measurement via optical tweezers reveals different kinetic features of two BRaf mutants responsible for cardio-facialcutaneous (CFC) syndrome. Biomed Opt Express (2013) 4(12):2835–45. doi:10.1364/boe.4.002835
123. Yu J, Tong X, Li C, Huang Y, Ye A. Using optical tweezers to investigate the specific single-interaction between apoA-I molecule and ABCA1 on living cells. Chin Opt Lett (2013) 11(9):091701–4. doi:10.3788/col201311.091701
124. Chen C, Tao T, Wen C, He W, Yn Q, Yq G, et al. Myosin light chain kinase (MLCK) regulates cell migration in a myosin regulatory light chain phosphorylation-independent mechanism. J Biol Chem (2014) 13:28478–88. doi:10.1074/jbc.m114.567446
125. Li Y, Wen C, Xie H, Ye A, Yin Y. Mechanical property analysis of stored red blood cell using optical tweezers. Colloids Surf B: Biointerfaces (2009) 70:169–73. doi:10.1016/j.colsurfb.2008.11.012
126. Zhang Y, Xu J, Yu Y, Shang W, Ye A. Anti-cancer drug sensitivity assay with quantitative heterogeneity testing using single-cell Raman spectroscopy. Molecules (2018) 23:2903. doi:10.3390/molecules23112903
127. Zhang Y, Jin L, Xu J, Yu Y, Shen L, Gao J, et al. Dynamic characterization of drug resistance and heterogeneity of the gastric cancer cell BGC823 using single-cell Raman spectroscopy,Dynamic characterization of drug resistance and heterogeneity of the gastric cancer cell BGC823 using single. -cell Raman spectroscopy,Analyst (2018) 143:164–74. doi:10.1039/c7an01287j
128. Lu-Di J, Jing-jing X, Yong Z, Yue-Zhou Y, Chang L, Dong-Ping Z, et al. YE an-pei, Raman spectroscopy analysis of chondrocyte dedifferentiation during in vitro proliferaion. Acta Phys -Chim Sin (2017) 33(12):2446–53. doi:10.3866/PKU.WHXB201706133
129. Jingjing X, Anpei Y. Laser trapping of Ag nanoparticles to enhance Raman spectroscopy in aqueous media. OPTICS EXPRESS (2019) 11(27):15528–39. doi:10.1364/OE.27.015528
130. Fang T, Shang W, Liu C, Xu J, Zhao D, Liu Y, et al. Nondestructive identification and accurate isolation of single cells through a chip with Raman optical Tweezers,Nondestructive identification and accurate isolation of single cells through a chip with Raman optical Tweezers,Anal. Anal Chem (2019) 91:9932–9. doi:10.1021/acs.analchem.9b01604
131. Fang T, Shang W, Liu C, Liu Y, Ye A. Single-cell multimodal analytical approach by integrating Raman optical tweezers and RNA Sequencing,Anal. Chem (2020) 92:10433–41. doi:10.1021/acs.analchem.0c00912
132. Fang T, Yuan P, Gong C, Jiang Y, Yu Y, Shang W, et al. Fast label-free recognition of NRBCs by deep-learning visual object detection and single-cell Raman spectroscopy. Analyst (2022) 147:1961–7. doi:10.1039/d2an00024e
133. Liu Y, Xu J, Tao Y, Fang T, Du W, Ye A. Rapid and accurate identification of marine microbes with single-cell Raman spectroscopy. Analyst (2020) 145:3297–305. doi:10.1039/c9an02069a
134. Zhou B, Sun L, Fang T, Li H, Zhang R, Ye A. Rapid and accurate identification of pathogenic bacteria at the single-cell level using laser tweezers Raman spectroscopy and deep learning.J. J Biophotonics (2022) 15:e202100312. doi:10.1002/jbio.202100312,
135. Tong Y, Fang T, Liu Y, Zhao D, Ye A. Research on hygroscopicity and volatility of single aerosol droplet. J Atmos Environ Opt (2020) 15:486–95.
136. Tong Y, Liu Y, Meng X, Wang J, Zhao D, Wu Z, et al. The relative humidity-dependent viscosity of single quasi aerosol particles and possible implications for atmospheric aerosol chemistry. Phys Chem Chem Phys (2022) 24:10514–23. doi:10.1039/D2CP00740A
137. Drever RWP, Hall JL, Kowalski FV, Hough J, Ford GM, Munley AJ, et al. Laser phase and frequency stabilization using an optical resonator. Appl Phys B (1983) 31:97–105. doi:10.1007/bf00702605
138. Chen J, Chen X. Optical lattice laser. In: Proceedings of the 2005 IEEE International Frequency Control Symposium and Exposition; 29-31 Aug. 2005; Vancouver, Canada. IEEE (2005).
139. Chen J. Active optical clock. Sci Bull (Beijing) (2009) 54:348–52. doi:10.1007/s11434-009-0073-y
140. Wang Y. Optical clocks based on stimulated emission radiation. Sci Bull (Beijing) (2009) 54:347. doi:10.1007/s11434-009-0064-z
141. Xu Z, Zhuang W, Wang Y, Wang D, Zhang X, Xue X, et al. Lasing of Cesium four-level active optical clock. In: Proceedings of the 2013 Joint European Frequency and Time Forum & International Frequency Control Symposium (EFTF/IFC); 21-25 July 2013; Prague, Czech Republic (2013). p. 395–8.
142. Pan D, Xu Z, Xue X, Zhuang W, Chen J. Lasing of cesium active optical clock with 459 nm laser pumping. In: Proceedings of the IEEE International Frequency Control Symposium (IFCS); 19-22 May 2014; Taipei, Taiwan (2014). p. 1–4.
143. Zhuang W, Chen J. Active Faraday optical frequency standard. Opt Lett (2014) 39:6339–42. doi:10.1364/ol.39.006339
144. Shi T, Pan D, Chen J. Realization of phase locking in good-bad-cavity active optical clock. Opt Express (2019) 27:22040–52. doi:10.1364/oe.27.022040
145. Pan D, Arora B, Yu Y, Sahoo BK, Chen J. Optical-lattice-based Cs active clock with a continual superradiant lasing signal. Phys Rev A (Coll Park) (2020) 102:041101. doi:10.1103/physreva.102.041101
146. Schäffer SA, Tang M, Henriksen MR, Jorgensen AA, Christensen BTR, Thomsen JW. Lasing on a narrow transition in a cold thermal strontium ensemble. Phys Rev A (Coll Park) (2020) 101:013819. doi:10.1103/physreva.101.013819
147. Norcia MA, Cline JRK, Muniz JA, Robinson JM, Hutson RB, Goban A, et al. Frequency measurements of superradiance from the strontium clock transition. Phys Rev X (2018) 8:021036. doi:10.1103/physrevx.8.021036
148. Laske T, Winter H, Hemmerich A. Pulse delay time statistics in a superradiant laser with calcium atoms. Phys Rev Lett (2019) 123:103601. doi:10.1103/physrevlett.123.103601
149. Maier T, Krämer SS, Ostermann L, Ritsch H. A superradiant clock laser on a magic wavelength optical lattice. Opt Express (2014) 22:13269–79. doi:10.1364/oe.22.013269
150. Bohnet JG, Chen Z, Weiner JM, Meiser D, Holland MJ, Thompson JK. A steady-state superradiant laser with less than one intracavity photon. Nature (2012) 484:78–81. doi:10.1038/nature10920
151. Meiser D, Ye J, Carlson DR, Holland MJ. Prospects for a millihertz-linewidth laser. Phys Rev Lett (2009) 102:163601. doi:10.1103/physrevlett.102.163601
152. Debnath K, Zhang Y, Mølmer K. Lasing in the superradiant crossover regime. Phys Rev A (Coll Park) (2018) 98:063837. doi:10.1103/physreva.98.063837
153. Roth A, Hammerer K. Synchronization of active atomic clocks via quantum and classical channels. Phys Rev A (Coll Park) (2016) 94:043841. doi:10.1103/physreva.94.043841
154. Kazakov GA, Dubey S, Bychek A, Sterr U, Bober M, Zawada M. Ultimate stability of active optical frequency standards. At Phys (physics.atom-ph) [Preprint] (2022). doi:10.48550/arXiv.2205.14130
155. Zhang S, Zhang X, Jiang Z, Pan D, Peng X, Chen H, et al. A compact optical clock scheme based on caesium atomic beam. In: Proceedings of the IEEE International Frequency Control Symposium (IFCS); 09-12 May 2016; New Orleans, LA, USA (2016). p. 1–4.
156. Shang H, Zhang X, Zhang S, Pan D, Chen H, Chen J. Miniaturized calcium beam optical frequency standard using fully-sealed vacuum tube with 10^−15 instability. Opt Express (2017) 25:30459–67. doi:10.1364/oe.25.030459
Keywords: quantum precision measurement, cold atom physics, nuclear magnetic resonance, Bose–Einstein condensate, optical tweezers, atomic clock, optical clock
Citation: Chen X, Zhou X, Ye A, Wang Y and Chen J (2022) The scientific career and contributions of Prof. Wang Yiqiu. Front. Phys. 10:1012446. doi: 10.3389/fphy.2022.1012446
Received: 05 August 2022; Accepted: 31 August 2022;
Published: 15 September 2022.
Edited by:
Joan Marler, Clemson University, United StatesReviewed by:
Yin Cai, Xi’an Jiaotong University, ChinaYoubin Yu, Zhejiang Sci-Tech University, China
Copyright © 2022 Chen, Zhou, Ye, Wang and Chen. This is an open-access article distributed under the terms of the Creative Commons Attribution License (CC BY). The use, distribution or reproduction in other forums is permitted, provided the original author(s) and the copyright owner(s) are credited and that the original publication in this journal is cited, in accordance with accepted academic practice. No use, distribution or reproduction is permitted which does not comply with these terms.
*Correspondence: Xuzong Chen, eHV6b25nY2hlbkBwa3UuZWR1LmNu