- 1Center for Memory and Recording Research, University of California San Diego, San Diego, CA, United States
- 2Department of Electrical and Computer Engineering, University of California San Diego, San Diego, CA, United States
We report on the spin Hall effect in epitaxial Pt films with well-defined crystalline (200), (220), and (111) orientations and smooth surfaces. The magnitude of the spin Hall effect has been determined by spin–torque ferromagnetic resonance measurements on epitaxial Pt/Py heterostructures. We observed a 54% enhancement of the charge-to-spin conversion efficiency of the epitaxial Pt when currents are applied along the in-plane
Introduction
Over the past decade, significant research efforts have been devoted to investigating magnetization manipulation in the heavy metal (HM)/ferromagnetic material (FM) heterostructure via spin–orbit torque (SOT) [1–6]. By engineering the bulk spin Hall effect (SHE) in HMs [7, 8] and interfacial Rashba–Edelstein effect (REE) [9–11], enhanced SOT values can be achieved that have the potential for developing novel energy-efficient magnetic memory [12], logic [13], and neuromorphic computing devices [14]. Conventional SOT studies mainly focus on textured HMs such as Pt [15], Au [16, 17],
In this letter, we detail the growth of epitaxial Pt thin films and Pt/FM heterostructures with (200), (220), and (111) crystalline orientations. In epitaxial films, symmetries of the magnetic interactions will reflect the underlying crystal and interface symmetries where the three orientations studied have four-fold, two-fold, and three-fold surface symmetries, respectively. The symmetries should be reflected in fundamental properties such as interfacial anisotropy (both in-plane and out-of-plane) [37] and Dzyaloshinskii–Moriya interaction (DMI) [38, 39]. For low-symmetry systems such as Pt (220) with C2v, the strength of the DMI may vary in magnitude or sign along different directions [40–44]. Such anisotropic DMI and anisotropy can stabilize novel phases such as antiskyrmions [41].
In this study, we focus on the SHE with the current flowing in various symmetry directions in Pt. By quantitatively evaluating the SOT along in-plane crystalline orientations via spin torque–FMR (ST-FMR) measurements, isotropic and anisotropic SHE have been observed and the role of the crystal symmetry enumerated. Moreover, by performing temperature-dependent harmonic measurements, we further reveal the intrinsic and extrinsic mechanisms underlying the SHE in epitaxial and polycrystalline Pt films. By combining directional-dependent SOT and anisotropic magnetic properties, we anticipate energy-efficient magnetization manipulation in novel spin structures.
Sample Growth and Characterization
Epitaxial Pt films were grown onto single-crystalline MgO (200), MgO (220), and Al2O3
The crystallographic properties of as-deposited Pt films were evaluated by X-ray diffraction (XRD) measurements. The out-of-plane symmetric
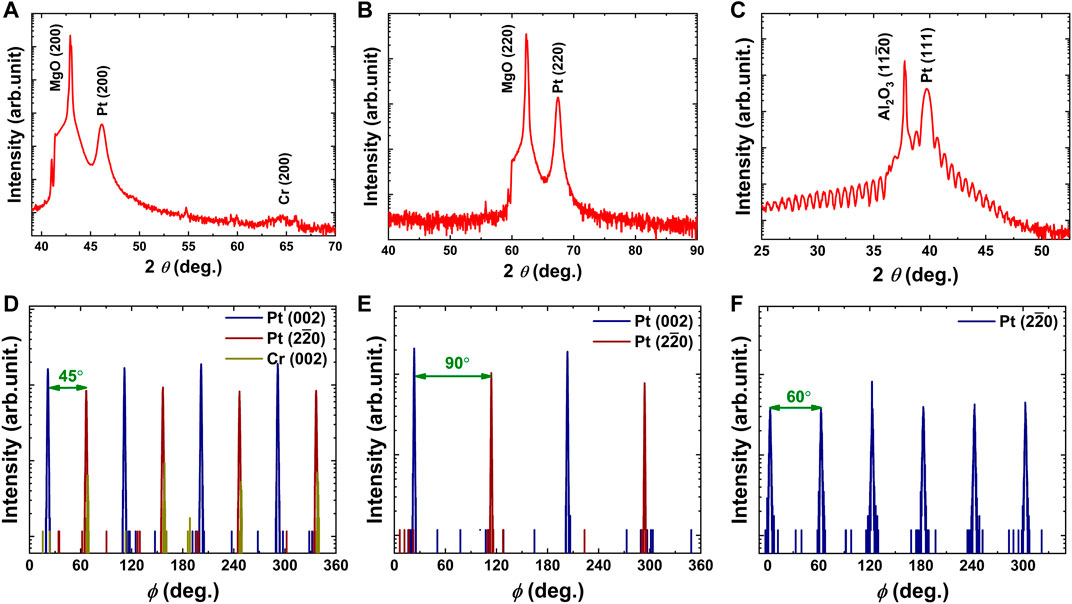
FIGURE 1. Out-of-plane XRD scan of epitaxial Pt films: (A) MgO (200)/Cr (200)/Pt (200). (B) MgO (220)/Pt (220). (C) Al2O3
Experimental Results and Discussion
ST-FMR Measurements
We first introduce our ST-FMR measurement technique to characterize the charge-to-spin conversion efficiency
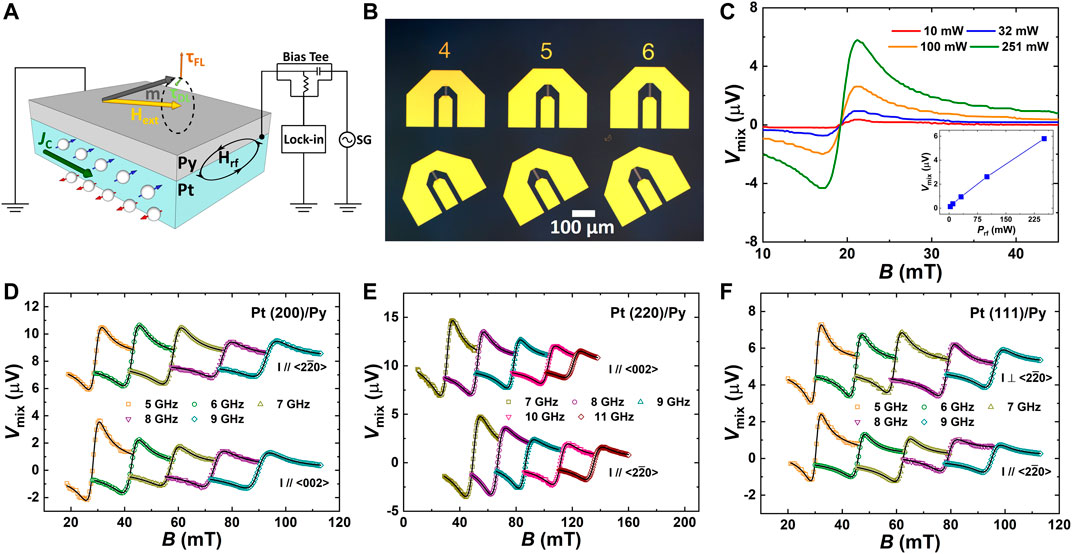
FIGURE 2. (A) Schematic diagram of ST-FMR measurement setup. (B) Optical microscope image of patterned Pt/Py CPWs with ground–signal–ground electrodes. (C) ST-FMR spectra measured at different RF power. Insert: the ST-FMR signal Vmix with a linear dependence on the input microwave power. (D)–(F) The measured ST-FMR spectra (open dots) with fitting curves (solid lines) on Pt (200)/Py, Pt (220)/Py, and Pt (111)/Py, respectively. The curves are offset for visual clarity.
Figure 2B shows the optical image of the photolithographically patterned microstrips with varied aspect ratios for impendence matching and two different orientations of the current. Coplanar wave guide (CPW) channels are patterned in certain angles to align with the crystalline orientations in the prepared Pt/Py films. Ti (6)/Au (200) pads are fabricated for symmetric ground–signal–ground contact electrodes by a standard sputtering and lift-off technique. The RF current is applied to the CPW channels via wire bonding from transmission lines to the ground–signal–ground electrodes. The in-plane external magnetic field is oriented 45° relative to the CPWs to improve the magnitude of the measured ST-FMR signals [46]. Measurement of the induced DC voltages takes advantage of a bias tee which separates the input RF microwave currents and the ST-FMR signals. All the ST-FMR measurements presented in this work were performed at room temperature. The measured DC voltage follows a linear dependence on the applied microwave power, as shown in Figure 2C, suggesting the marginal role of the Joule heating effect in our measurements.
The ST-FMR technique provides a quantitative measurement of the
where the parameter
where
Figures 2D–F show the experimental ST-FMR resonance spectrum measured on Pt (200)/Py, Pt (220)/Py, and Pt (111)/Py samples with microwave frequencies varying from 5 to 11 GHz. Due to the larger saturation field of Py, measurements of the Pt (220)/Py sample are mainly focused in the high-frequency regime. For Pt (220)/Py, the shift of the resonance fields when the RF current applied along different crystalline directions is attributed to the in-plane anisotropy of Py induced by Pt (220) with low symmetry (C2v), while in high-symmetry systems (C4v and C3v) of Pt (200) and Pt (111) samples, nearly isotropic Py magnetic properties make the resonance fields independent of the in-plane direction. The experimental results (open dots) were well-fitted with Eq. 1 (solid lines). We note that
To independently verify the results obtained from the lineshape method, we also performed linewidth modulation measurements. By applying a DC current to the patterned Pt/Py microstrip, a static anti-damping torque effectively modulates the Gilbert damping of Py, resulting in a systematic current-dependent variation of the linewidth of the obtained ST-FMR spectra. Based on the spin-transfer torque (STT) model [56], the injected DC spin currents effectively increase (decrease) the damping of the Py layer when the spin polarization is parallel (antiparallel) to the Py magnetization, leading to a broadened (reduced) ST-FMR linewidth [15, 60]. Furthermore, reversing the polarity of the external magnetic field that saturates Py magnetization will also lead to the sign change of the observed signals, as illustrated in Figure 3. Quantitatively,
where
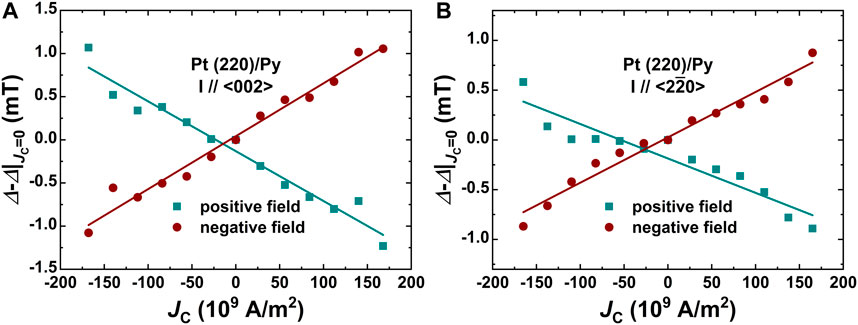
FIGURE 3. Variation of FMR linewidth as a function of applied DC current at a microwave frequency f = 7 GHz for current along (A)
To summarize our ST-FMR results, Table 1 shows the obtained

TABLE 1. Crystalline orientation–dependent longitudinal resistivities and charge-to-spin conversion efficiencies of epitaxial Pt films and Pt/FM structures.
As the thickness of the measured Pt films is greater than the spin diffusion length
Temperature-Dependent Harmonic Measurements
To further understand the resistivity-dependent SHE of the epitaxial Pt films, we performed temperature-dependent harmonic measurements on patterned Pt/Co(0.8)/Ni(1) Hall devices, as illustrated in Figure 4A. Figure 4B shows the characteristic first and second harmonic Hall results measured in the prepared device. For bulk SHE, when a charge current
and the
where
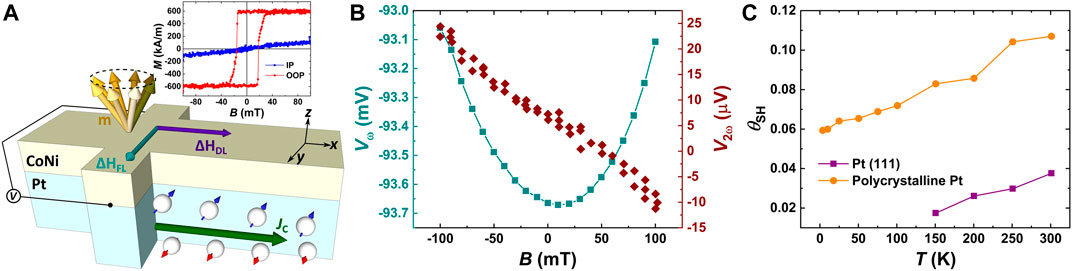
FIGURE 4. (A) Schematic diagram of harmonic measurements of Pt/Co(0.8)/Ni(1) samples. The aspect ratio of the Hall cross is 1:3 to minimize Joule heating effects. Insert: M vs B curves on Pt (111)/Co(0.8)/Ni(1). Measured in-plane longitudinal field dependence of (B) first-harmonic Hall signal and second-harmonic Hall signal in Pt/Co(0.8)/Ni(1) sample. (C) Temperature dependence of the charge-to-spin conversion efficiency measured on polycrystalline Pt and epitaxial Pt (111).
Figure 4C presents the obtained temperature-dependent
Conclusion
In summary, we have prepared high-quality epitaxial Pt thin films on a series of substrates. Systematic ST-FMR measurements demonstrate the isotropic nature of SHE in the high-symmetry Pt (200) and Pt (111) films. In contrast, the low-symmetry system such as (220) orientated Pt exhibits the anisotropic SHE behavior that is correlated to the anisotropic resistivity. The temperature-dependent harmonic measurements further suggest that SOT can be a hint for “cleaner” metals with more extrinsic contribution to SHE. The observed crystalline orientation–dependent
Data Availability Statement
The raw data supporting the conclusions of this article will be made available by the authors, without undue reservation.
Author Contributions
YX and EF conceived the idea and designed the project. YX fabricated devices and performed characterization. YX and HW performed the measurements. All authors discussed the results and contributed to the final manuscript.
Funding
This research was supported as part of Quantum Materials for Energy Efficient Neuromorphic Computing, an Energy Frontier Research Center (QMEEN-C EFRC) funded by the United States DOE, Office of Science under Award No. DE-SC0019273. Device microfabrication was performed at the San Diego Nanotechnology Infrastructure at University of California, San Diego (UCSD), a member of the National Nanotechnology Coordinated Infrastructure, which is supported by the National Science Foundation (Grant ECCS-1542148).
Conflict of Interest
The authors declare that the research was conducted in the absence of any commercial or financial relationships that could be construed as a potential conflict of interest.
Publisher’s Note
All claims expressed in this article are solely those of the authors and do not necessarily represent those of their affiliated organizations, or those of the publisher, the editors, and the reviewers. Any product that may be evaluated in this article, or claim that may be made by its manufacturer, is not guaranteed or endorsed by the publisher.
Acknowledgments
The authors thank R. Descoteaux for technical assistance.
Supplementary Material
The Supplementary Material for this article can be found online at: https://www.frontiersin.org/articles/10.3389/fphy.2021.791736/full#supplementary-material
References
1. Miron IM, Garello K, Gaudin G, Zermatten P-J, Costache MV, Auffret S, et al. Perpendicular Switching of a Single Ferromagnetic Layer Induced by In-Plane Current Injection. Nature (2011) 476:189–93. doi:10.1038/nature10309
2. Miron IM, Moore T, Szambolics H, Buda-Prejbeanu LD, Auffret S, Rodmacq B, et al. Fast Current-Induced Domain-wall Motion Controlled by the Rashba Effect. Nat Mater (2011) 10:419–23. doi:10.1038/nmat3020
3. Liu L, Pai C-F, Li Y, Tseng HW, Ralph DC, Buhrman RA. Spin-Torque Switching with the Giant Spin Hall Effect of Tantalum. Science (2012) 336:5. doi:10.1126/science.1218197
4. Liu L, Lee OJ, Gudmundsen TJ, Ralph DC, Buhrman RA. Current-Induced Switching of Perpendicularly Magnetized Magnetic Layers Using Spin Torque from the Spin Hall Effect. Phys Rev Lett (2012) 109:096602. doi:10.1103/physrevlett.109.096602
5. Han J, Richardella A, Siddiqui SA, Finley J, Samarth N, Liu L. Room-Temperature Spin-Orbit Torque Switching Induced by a Topological Insulator. Phys Rev Lett (2017) 119:077702. doi:10.1103/physrevlett.119.077702
6. Jiang M, Asahara H, Sato S, Kanaki T, Yamasaki H, Ohya S, et al. Efficient Full Spin-Orbit Torque Switching in a Single Layer of a Perpendicularly Magnetized Single-Crystalline Ferromagnet. Nat Commun (2019) 10:2590. doi:10.1038/s41467-019-10553-x
8. Tanaka T, Kontani H, Naito M, Naito T, Hirashima DS, Yamada K, et al. Intrinsic Spin Hall Effect and Orbital Hall Effect In4dand5dtransition Metals. Phys Rev B (2008) 77:165117. doi:10.1103/physrevb.77.165117
9. Rashba EI. Properties of Semiconductors with an Extremum Loop. I. Cyclotron and Combinational Resonance in a Magnetic Field Perpendicular to the Plane of the Loop. Sov Phys Solid State (1960) 2:1109.
10. Rashba EI, Bychkov YA. Oscillatory Effects and the Magnetic Susceptibility of Carriers in Inversion Layers. J Phys C (1984) 17:6039.
11. Edelstein VM. Spin Polarization of Conduction Electrons Induced by Electric Current in Two-Dimensional Asymmetric Electron Systems. Solid State Commun (1990) 73:233–5. doi:10.1016/0038-1098(90)90963-c
12. Lau Y-C, Betto D, Rode K, Coey JMD, Stamenov P. Spin-orbit Torque Switching without an External Field Using Interlayer Exchange Coupling. Nat Nanotech (2016) 11:758–62. doi:10.1038/nnano.2016.84
13. Khitun A, Bao M, Wang KL. Magnonic Logic Circuits. J Phys D: Appl Phys (2010) 43:264005. doi:10.1088/0022-3727/43/26/264005
14. Grollier J, Querlioz D, Camsari KY, Everschor-Sitte K, Fukami S, Stiles MD. Neuromorphic Spintronics. Nat Electron (2020) 3:360–70. doi:10.1038/s41928-019-0360-9
15. Liu L, Moriyama T, Ralph DC, Buhrman RA. Spin-Torque Ferromagnetic Resonance Induced by the Spin Hall Effect. Phys Rev Lett (2011) 106:036601. doi:10.1103/physrevlett.106.036601
16. Mihajlović G, Pearson JE, Garcia MA, Bader SD, Hoffmann A. Negative Nonlocal Resistance in Mesoscopic Gold Hall Bars: Absence of the Giant Spin Hall Effect. Phys Rev Lett (2009) 103:166601.
17. El Hadri MS, Gibbons J, Xiao Y, Ren H, Arava H, Liu Y, et al. Large Spin-to-Charge Conversion in Ultrathin Gold-Silicon Multilayers. Phys Rev Mater (2021) 5:064410. doi:10.1103/physrevmaterials.5.064410
18. Pai C-F, Liu L, Li Y, Tseng HW, Ralph DC, Buhrman RA. Spin Transfer Torque Devices Utilizing the Giant Spin Hall Effect of Tungsten. Appl Phys Lett (2012) 101:122404. doi:10.1063/1.4753947
19. Ren H, Wu S-Y, Sun JZ, Fullerton EE. Ion Beam Etching Dependence of Spin-Orbit Torque Memory Devices with Switching Current Densities Reduced by Hf Interlayers. APL Mater (2021) 9:091101. doi:10.1063/5.0060461
20. Kim J, Sinha J, Hayashi M, Yamanouchi M, Fukami S, Suzuki T, et al. Layer Thickness Dependence of the Current-Induced Effective Field Vector in Ta|CoFeB|MgO. Nat Mater (2013) 12:240–5. doi:10.1038/nmat3522
21. Chen T-Y, Wu C-T, Yen H-W, Pai C-F. Tunable Spin-Orbit Torque in Cu-Ta Binary alloy Heterostructures. Phys Rev B (2017) 96:104434. doi:10.1103/physrevb.96.104434
22. Ou Y, Ralph DC, Buhrman RA. Strong Enhancement of the Spin Hall Effect by Spin Fluctuations near the Curie Point of FexPt1–x Alloys. Phys .Rev .Lett (2018) 120:097203. doi:10.1103/physrevlett.120.097203
23. Zhang W, Jungfleisch MB, Freimuth F, Jiang W, Sklenar J, Pearson JE, et al. All-electrical Manipulation of Magnetization Dynamics in a Ferromagnet by Antiferromagnets with Anisotropic Spin Hall Effects. Phys Rev B (2015) 92:144405. doi:10.1103/physrevb.92.144405
24. Ryu J, Avci CO, Karube S, Kohda M, Beach GSD, Nitta J. Crystal Orientation Dependence of Spin-Orbit Torques in Co/Pt Bilayers. Appl Phys Lett (2019) 114:142402. doi:10.1063/1.5090610
25. Wang H, Meng K-Y, Zhang P, Hou JT, Finley J, Han J, et al. Large Spin-Orbit Torque Observed in Epitaxial SrIrO3 Thin Films. Appl Phys Lett (2019) 114:232406. doi:10.1063/1.5097699
26. Seki T, Iihama S, Taniguchi T, Takanashi K. Large Spin Anomalous Hall Effect in L10−FePt : Symmetry and Magnetization Switching. Phys Rev B (2019) 100:144427. doi:10.1103/physrevb.100.144427
27. Li H, Wang G, Li D, Hu P, Zhou W, Ma X, et al. Spin-orbit Torque-Induced Magnetization Switching in Epitaxial Au/Fe4N Bilayer Films. Appl Phys Lett (2019) 114:092402. doi:10.1063/1.5078395
28. Gabor MS, Petrisor T, Nasui M, Nath J, Miron IM. Spin-Orbit Torques and Magnetization Switching in Perpendicularly Magnetized Epitaxial Pd/Co2FeAl/MgO Structures. Phys Rev Appl (2020) 13:054039. doi:10.1103/physrevapplied.13.054039
29. Thompson R, Ryu J, Du Y, Karube S, Kohda M, Nitta J. Current Direction Dependent Spin Hall Magnetoresistance in Epitaxial Pt/Co Bilayers on MgO(110). Phys Rev B (2020) 101:214415. doi:10.1103/physrevb.101.214415
30. Thompson R, Ryu J, Choi G, Kohda M, Nitta J, et al. Anisotropic Spin-Orbit Torque through Crystal-Orientation Engineering in Epitaxial Pt. Phys Rev Appl (2021) 15:014055. doi:10.1103/physrevapplied.15.014055
31. Ryu J, Kohda M, Nitta J. Observation of the D'yakonov-Perel' Spin Relaxation in Single-Crystalline Pt Thin Films. Phys Rev Lett (2016) 116:256802. doi:10.1103/physrevlett.116.256802
32. Gamou H, Du Y, Kohda M, Nitta J. Enhancement of Spin Current Generation in Epitaxial α -Ta/CoFeB Bilayer. Phys Rev B (2019) 99:184408. doi:10.1103/physrevb.99.184408
33. Hong D, Anand N, Liu C, Liu H, Arslan I, Pearson JE, et al. Large Anomalous Nernst and Inverse Spin-Hall Effects in Epitaxial Thin Films of Kagome Semimetal Mn3Ge. Phys Rev Mater (2020) 4:094201. doi:10.1103/physrevmaterials.4.094201
34. Khang NHD, Ueda Y, Hai PN. A Conductive Topological Insulator with Large Spin Hall Effect for Ultralow Power Spin-Orbit Torque Switching. Nat Mater (2018) 17:808–13. doi:10.1038/s41563-018-0137-y
35. Jamali M, Lee JS, Jeong JS, Mahfouzi F, Lv Y, Zhao Z, et al. Giant Spin Pumping and Inverse Spin Hall Effect in the Presence of Surface and Bulk Spin−Orbit Coupling of Topological Insulator Bi2Se3. Nano Lett (2015) 15:7126–32. doi:10.1021/acs.nanolett.5b03274
36. Zhang W, Han W, Yang S-H, Sun Y, Zhang Y, Yan B, et al. Giant Facet-dependent Spin-Orbit Torque and Spin Hall Conductivity in the Triangular Antiferromagnet IrMn 3. Sci Adv (2016) 2:1600759. doi:10.1126/sciadv.1600759
37. Engel BN, England CD, van Leeuwen RA, Wiedmann MH, Falco CM. Interface Magnetic Anisotropy in Epitaxial Superlattices. Phys Rev Lett (1991) 67:1910–3. doi:10.1103/physrevlett.67.1910
38. Dzyaloshinskii I. A Thermodynamic Theory of Weak Ferromagnetism of Antiferromagnetics. J Phys Chem Sol (1958) 4:241.
39. Moriya T. Anisotropic Superexchange Interaction and Weak Ferromagnetism. Phys Rev (1960) 120:91.
40. Nembach HT, Shaw JM, Weiler M, Jué E, Silva TJ. Linear Relation between Heisenberg Exchange and Interfacial Dzyaloshinskii-Moriya Interaction in Metal Films. Nat Phys (2015) 11:825–9. doi:10.1038/nphys3418
41. Hoffmann M, Zimmermann B, Müller GP, Schürhoff D, Kiselev NS, Melcher C, et al. Antiskyrmions Stabilized at Interfaces by Anisotropic Dzyaloshinskii-Moriya Interactions. Nat Commun (2017) 8:1. doi:10.1038/s41467-017-00313-0
42. Camosi L, Rohart S, Fruchart O, Pizzini S, Belmeguenai M, Roussigné Y, et al. Anisotropic Dzyaloshinskii-Moriya Interaction in Ultrathin Epitaxial Au/Co/W(110). Phys Rev B (2017) 95:214422. doi:10.1103/physrevb.95.214422
43. Raeliarijaona A, Nepal R, Kovalev AA. Boundary Twists, Instabilities, and Creation of Skyrmions and Antiskyrmions. Phys Rev Mater (2018) 2:124401. doi:10.1103/physrevmaterials.2.124401
44. Tsurkan S, Zakeri K. Giant Dzyaloshinskii-Moriya Interaction in Epitaxial Co/Fe Bilayers with C2v Symmetry. Phys Rev B (2020) 102:060406. doi:10.1103/physrevb.102.060406
45. Fan Y, Upadhyaya P, Kou X, Lang M, Takei S, Wang Z, et al. Magnetization Switching through Giant Spin-Orbit Torque in a Magnetically Doped Topological Insulator Heterostructure. Nat Mater (2014) 13:699–704. doi:10.1038/nmat3973
46. Wang Y, Deorani P, Qiu X, Kwon JH, Yang H. Determination of Intrinsic Spin Hall Angle in Pt. Appl Phys Lett (2014) 105:152412. doi:10.1063/1.4898593
47. Mellnik AR, Lee JS, Richardella A, Grab JL, Mintun PJ, Fischer MH, et al. Spin-transfer Torque Generated by a Topological Insulator. Nature (2014) 511:449–51. doi:10.1038/nature13534
48. Nan T, Emori S, Boone CT, Wang X, Oxholm TM, Jones JGG, et al. Comparison of Spin-Orbit Torques and Spin Pumping across NiFe/Pt and NiFe/Cu/Pt Interfaces. Phys Rev B (2015) 91:214416. doi:10.1103/physrevb.91.214416
49. Xu JW, Kent AD. Charge-To-Spin Conversion Efficiency in Ferromagnetic Nanowires by Spin Torque Ferromagnetic Resonance: Reconciling Lineshape and Linewidth Analysis Methods. Phys Rev Appl (2020) 14:014012. doi:10.1103/physrevapplied.14.014012
50. Müller M, Liensberger L, Flacke L, Huebl H, Kamra A, Belzig W, et al. Growth Optimization of TaN for Superconducting Spintronics. Phys Rev Lett (2021) 126:087201.
51. Daalderop GHO, Kelly PJ, den Broeder FJA. Prediction and Confirmation of Perpendicular Magnetic Anisotropy in Co/Ni Multilayers. Phys Rev Lett (1992) 68:682–5. doi:10.1103/physrevlett.68.682
52. Brock JA, Montoya SA, Im M-Y, Fullerton EE. Energy-efficient Generation of Skyrmion Phases in Co/Ni/Pt-Based Multilayers Using Joule Heating. Phys Rev Mater (2020) 4:104409. doi:10.1103/physrevmaterials.4.104409
53. McMichael RD, Twisselmann DJ, Kunz A. Localized Ferromagnetic Resonance in Inhomogeneous Thin Films. Phys Rev Lett (2003) 90:227601. doi:10.1103/physrevlett.90.227601
54. Zhu L, Ralph DC, Buhrman RA. Effective Spin-Mixing Conductance of Heavy-Metal-Ferromagnet Interfaces. Phys Rev Lett (2019) 123:057203. doi:10.1103/physrevlett.123.057203
55. Lairson BM, Visokay MR, Sinclair R, Hagstrom S, Clemens BM. Epitaxial Pt(001), Pt(110), and Pt(111) Films on MgO(001), MgO(110), MgO(111), and Al2O3(0001). Appl Phys Lett (1992) 61:1390–2. doi:10.1063/1.107547
56. Slonczewski JC. Current-driven Excitation of Magnetic Multilayers. J Magnetism Magn Mater (1996) 159:L1–L7. doi:10.1016/0304-8853(96)00062-5
57. Sankey JC, Braganca PM, Garcia AGF, Krivorotov IN, Buhrman RA, Ralph DC. Spin-Transfer-Driven Ferromagnetic Resonance of Individual Nanomagnets. Phys Rev Lett (2006) 96:227601. doi:10.1103/physrevlett.96.227601
58. Sankey JC, Cui Y-T, Sun JZ, Slonczewski JC, Buhrman RA, Ralph DC. Measurement of the Spin-Transfer-Torque Vector in Magnetic Tunnel Junctions. Nat Phys (2008) 4:67–71. doi:10.1038/nphys783
59. Kittel C. On the Theory of Ferromagnetic Resonance Absorption. Phys Rev (1948) 73:155–61. doi:10.1103/physrev.73.155
60. Petit S, Baraduc C, Thirion C, Ebels U, Liu Y, Li M, et al. Spin-Torque Influence on the High-Frequency Magnetization Fluctuations in Magnetic Tunnel Junctions. Phys Rev Lett (2007) 98:077203. doi:10.1103/physrevlett.98.077203
61. Guillemard C, Petit-Watelot S, Andrieu S, Rojas-Sánchez J-C. Charge-spin Current Conversion in High Quality Epitaxial Fe/Pt Systems: Isotropic Spin Hall Angle along Different In-Plane Crystalline Directions. Appl Phys Lett (2018) 113:262404. doi:10.1063/1.5079236
62. Song C, Zhang R, Liao L, Zhou Y, Zhou X, Chen R, et al. Spin-orbit Torques: Materials, Mechanisms, Performances, and Potential Applications. Prog Mater Sci (2021) 118:100761. doi:10.1016/j.pmatsci.2020.100761
63. Ciccarelli C, Anderson L, Tshitoyan V, Ferguson AJ, Gerhard F, Gould C, et al. Room-temperature Spin-Orbit Torque in NiMnSb. Nat Phys (2016) 12:855–60. doi:10.1038/nphys3772
64. Simon E, Szilva A, Ujfalussy B, Lazarovits B, Zarand G, Szunyogh L. Anisotropic Rashba Splitting of Surface States from the Admixture of Bulk States: Relativisticab Initiocalculations Andk⋅pperturbation Theory. Phys Rev B (2010) 81:235438. doi:10.1103/physrevb.81.235438
65. Hayashi M, Kim J, Yamanouchi M, Ohno H. Quantitative Characterization of the Spin-Orbit Torque Using Harmonic Hall Voltage Measurements. Phys Rev B (2014) 89:144425. doi:10.1103/physrevb.89.144425
66. Garello K, Miron IM, Avci CO, Freimuth F, Mokrousov Y, Blügel S, et al. Symmetry and Magnitude of Spin-Orbit Torques in Ferromagnetic Heterostructures. Nat Nanotech (2013) 8:587–93. doi:10.1038/nnano.2013.145
67. Zheng Z, Zhang Y, Feng X, Zhang K, Nan J, Zhang Z, et al. Enhanced Spin-Orbit Torque and Multilevel Current-Induced Switching in W/Co−Tb/Pt Heterostructure. Phys Rev Appl (2019) 12:044032. doi:10.1103/physrevapplied.12.044032
68. Pai C-F, Mann M, Tan AJ, Beach GSD. Determination of Spin Torque Efficiencies in Heterostructures with Perpendicular Magnetic Anisotropy. Phys Rev B (2016) 93:144409. doi:10.1103/physrevb.93.144409
69. Seung Ham W, Kim S, Kim D-H, Kim K-J, Okuno T, Yoshikawa H, et al. Temperature Dependence of Spin-Orbit Effective fields in Pt/GdFeCo Bilayers. Appl Phys Lett (2017) 110:242405. doi:10.1063/1.4985436
70. Nagaosa N, Sinova J, Onoda S, MacDonald AH, Ong NP. Anomalous Hall Effect. Rev Mod Phys (2010) 82:1539. doi:10.1103/revmodphys.82.1539
Keywords: spin Hall effect, spin torque ferromagnetic resonance, harmonics, epitaxial, platinum
Citation: Xiao Y, Wang H and Fullerton EE (2022) Crystalline Orientation–Dependent Spin Hall Effect in Epitaxial Platinum. Front. Phys. 9:791736. doi: 10.3389/fphy.2021.791736
Received: 08 October 2021; Accepted: 06 December 2021;
Published: 05 January 2022.
Edited by:
Weiwei Lin, Southeast University, ChinaReviewed by:
Cheng Song, Tsinghua University, ChinaGuoqiang Yu, Institute of Physics (CAS), China
Shiheng Liang, Hubei University, China
Copyright © 2022 Xiao, Wang and Fullerton. This is an open-access article distributed under the terms of the Creative Commons Attribution License (CC BY). The use, distribution or reproduction in other forums is permitted, provided the original author(s) and the copyright owner(s) are credited and that the original publication in this journal is cited, in accordance with accepted academic practice. No use, distribution or reproduction is permitted which does not comply with these terms.
*Correspondence: Eric E. Fullerton, ZWZ1bGxlcnRvbkB1Y3NkLmVkdQ==