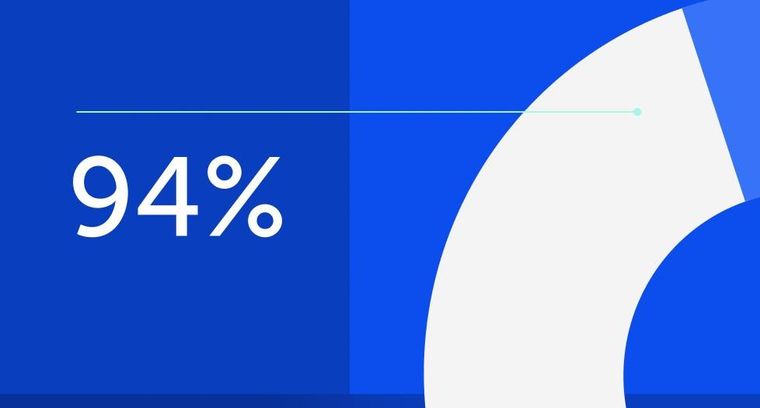
94% of researchers rate our articles as excellent or good
Learn more about the work of our research integrity team to safeguard the quality of each article we publish.
Find out more
REVIEW article
Front. Phys., 15 November 2021
Sec. Optics and Photonics
Volume 9 - 2021 | https://doi.org/10.3389/fphy.2021.751018
This article is part of the Research TopicAdvances in Terahertz Detection and ImagingView all 15 articles
Compared with other typical terahertz (THz) detectors, the quantum-well photodetector (QWP) has the advantages of high detection sensitivity, fast response, mature fabrication, small size, and easy integration. Therefore, it is suitable for high-speed detection and imaging applications at the THz band. Researchers, both domestic and overseas, have systematically studied material design and device performance of the THz QWP. The design of the device is such that the peak frequency error is within 8%, the maximum peak responsibility is 5.5 A/W, the fastest response speed is 6.2 GHz, the best noise equivalent power is ∼10−13 W/Hz0.5, and the spectrum range is 2.5–6.5 THz. In this article, firstly the basic principles and theoretical calculations of the THz QWP are described, and then the research progress of the THz QWP in our research group at imaging and communication is reviewed, which looks forward to its future development.
Terahertz (THz) waves are electromagnetic waves falling between the millimeter and infrared [1, 2] spectrum as shown in Figure 1. This region ranges in frequency from about 0.1 to 10 THz (30–1000 μm). It has the advantages of strong dielectric penetration and weak ionization. It has a broad application prospect in material research, environmental monitoring, nondestructive testing, medical diagnosis, wireless communication, and other fields.
FIGURE 1. The spectrum of THz wave [2].
THz detectors have been studied since 1960s [3]. THz quantum-well photodetector (QWP) is the natural expansion of quantum-well infrared detector (QWIP) at the THz band [4–7]. The THz QWP material system is GaAs/AlGaAs, which is usually composed of many quantum wells. When THz wave radiation is incident on the THz QWP, the electrons in the bound state of the quantum well absorb THz photons and transit to the continuous state, forming a photocurrent under the external bias voltage, so as to complete the detection of the THz waves [8]. Compared with the QWIP, the energy between the bound and the continuous state in the THz QWP is just 20 meV, and the doping in the quantum well is lower, which directly leads to the decreasing absorption efficiency and the responsibility. In 2004, H.C. Liu and coworkers [9] developed a quantum-well detector working in the THz band by reducing the barrier height and doping in the quantum well. Reference 40 used the THz QWP to demonstrate THz communication. Professor Hu Qing, an authoritative expert in the THz field at the Massachusetts Institute of Technology, believes that THz QWP may improve the speed and sensitivity of THz imaging. The main problem of the THz QWP [10, 11] is that the energy difference between the first bound sub-band in the quantum well and the quasi-continuous state is less than 30 meV. The signal-to-noise ratio (SNR) of the device needs to be improved by limiting the thermally excited electrons, such that the device needs to work at a low temperature (<20 K). According to the differences in the active structure, internal charge distribution, and carrier transport mode of the detector, the QWP is divided into photoconductive detector and photovoltaic detector. The active region and conduction band structure of the photoconductive THz QWP are shown in Figure 2.
Due to the high degree of freedom of the multiparticle system, the many-body effect can only be approximated [12, 13]. Considering the interaction between electrons, we established on the basis of density functional theory, combined with the local density approximation (LDA), meanwhile considering including exchange self-energies effect, depolarization-shift effect, and excitonic effect, between the depolarization and exciton interaction contributes to the optical many-body effect. The many-body effect is an electronic collective effect. When the wavelength is extended to the THz region because of the small carrier transition energy involved in the detection process, the influence of the many-body effect cannot be ignored, which needs to be considered during device design.
The THz QWP design needs to satisfy the following Equation (9) [14], [15]:
1) The Schrodinger equation is as follows:
where,
where,
The Poisson equation is as follows:
where,
where,
2) For the THz QWP (consider many-body), the Hamilton function is as follows:
where, p is the momentum,
Considering the condition of the Schrodinger equation,
Electronic charge is
Electronic density is
When the electron density is known, the Hartley potential can be obtained by solving the Poisson equation
In this study, researches were carried out based on the density functional theory. When the energy dispersion relations and wave functions can be obtained by Fermi's golden rule, the relationship between them is as follows:
Compared with electromagnetic waves in other bands, the THz waves have many excellent characteristics, such as good penetrability to nonpolar substances, characteristic spectrum of biological macromolecules, and non-damage to human body [15–21]. THz waves have been used for imaging applications since 1990. The principle of THz imaging [22–26] is to obtain a THz image of the sample by collecting the amplitude, phase, and polarization information reflected or transmitted at different positions of the sample, and transforming the collected THz information into parameters that can reflect the physical and chemical properties of the sample, then corresponding to the spatial position parameters of the sample, combining with image restoration technology. THz imaging has many classification methods. According to the different ways of acting on the sample, it can be divided into transmission imaging and reflection imaging. According to the different working modes of the THz light source, it can be divided into continuous- wave imaging and pulse imaging. According to the imaging speed, it can be divided into scanning imaging and real-time imaging. According to whether the hidden wave effect is considered, it can be divided into far-field imaging and near-field imaging.
Tan Zhiyong et al. from the Shanghai Institute of Microsystem and Information Technology (SIMIT) developed the fast scanning imaging system based on THz quantum devices [27]. The system used 4.3 THz quantum cascade laser (QCL) as the THz light source and a high-speed THz QWP with matching frequency. A rotating scanning platform was used to realize rapid scanning of the sample (Figure 3), so as to improve the imaging speed of the system. The system used a new pulse signal acquisition method and a practical image restoration program to obtain THz images of blades and shaving blades under different shelters within 5 s. In addition, the system also used an image processing algorithm to deal with the noise problem during practical application, which improves the quality of the THz images.
FIGURE 3. The fast scanning imaging system [27].
Wang Chang from SIMIT set up a reflective THz fast confocal imaging system [28](Figure 4). The THz light source used was 4.3 THz QCL, with a THz QWP and fast-rotating translation platform to complete the fast scanning imaging of the sample. The imaging optical path adopts confocal microscopy. Two 200-μm pinholes were placed at the two conjugate focuses to filter out the scattered light of the system and improve the spatial resolution of the imaging system. Finally, lateral resolution better than 110 μm and axial resolution about 320 μm were achieved. The two-dimensional THz imaging of common metal samples and toothpicks was carried out by using the rapid confocal microscopy imaging system, and a high-contrast image of the samples was obtained within 5 s. Finally, using the high axial resolution of the system, three-dimensional slice imaging of the sample was carried out. The imaging results are clearly visible, and the thickness of the sample could be measured.
FIGURE 4. The fast THz confocal microscopic imaging system [28].
Qiu Fucheng [30] established a scattering-type scanning near-field optical microscopy system based on the THz QCL and THz QWP. Using the self-developed THz QCL and THz QWP, the low-bias continuous-wave THz QCL laser source module and THz QWP high-sensitivity detection module were developed for near-field microscopic imaging, and the scattering-type scanning near-field optical microscopic imaging system was built. The demodulation of the THz near-field signal was completed by using signal high-order demodulation technology. The THz imaging system with THz scattering-type scanning near-field optical microscopy was used to image pure gold samples with scratches. The minimum resolution of the system was calculated to be about 200 nm (Figure 5).
FIGURE 5. The THz scanning near-field optical microscopic imaging system [30].
In 2016, Z.L. Fu et al. from SIMIT fabricated the THz frequency up-conversion device (THz QWP-LED) formed by stacking THz QWPs and the light-emitting diode (LED) and using this device for pixel-less imaging. The 45-degree coupled THz QWP-LED imaging system is shown in Figure 6A The peak frequency is 5.2 THz, the peak responsibility is 0.22 A/W, the noise equivalent power is 5.2 × 10−12 W/Hz0.5, and the operating temperature is 3.5 K. The frequency point of THz QCL RF is 4.3 THz. Figure 6B shows the imaging results of THz QWP-LED for THz QCL with different laser powers. The imaging resolution is better than 0.05 mm; the SNR is better than 10,000; and the imaging time is 1 s. At present, the one-dimensional and two-dimensional metal grating coupling devices with normal incidence have achieved real-time imaging. The two-dimensional metal grating coupling device can rapidly image the THz QCL laser spot within 10 µs. The energy of the imaging spot is Gaussian distribution, which is basically consistent with the results of long imaging time. This experiment shows that the frequency up-conversion device based on THz QWP has high-resolution and high-speed imaging ability.
FIGURE 6. (A) Set-up for THz pixel-less imaging system; (B) The focal laser spots of THz QCL imaged by using THz QWP-LED [31].
Terahertz is used in various imaging scenes, such as in intelligence, security, crime detection, biology, and medicine [32–35]. At present, some THz passive imaging security products have been released, such as the ThruVision series released by Digital Barriers. It works at 0.25 THz; the detection distance is 4–10 m; and the imaging speed can reach 6 frames/s. The device can effectively compensate for the limitations of slightly poor light transmittance, macromolecules that cannot penetrate, imaging contrast, and so on when visible light and X-rays are used for imaging. The application of THz imaging can supplement these disadvantages. THz waves have no ionization characteristics, and they can safely and nondestructively detect the human body through clothing. Therefore, these can be used for human security and the security of some prohibited items (Figure 7), and also for the identification of ancient cultural relics. The most popular is THz tomography (THz CT), which can scan molecules such as materials and samples.
FIGURE 7. L3 security and detection systems [32].
In the field of medicine, THz radiation is used for low-energy, nondestructive detection and minimizes radiation damage to the human body. Compared with X-rays, THz waves do little damage to cells and objects and can be applied for organ identification and in gene research. They can also be used in dental treatment, examination of skin cancer and other diseases, real-time imaging in surgery, medical diagnosis, biomedical engineering, and other medical fields.
In terms of environmental monitoring, THz waves can analyze and characterize the chemical components of solid, liquid, gas, and other states. It can also be used for pollutant detection, such as compound leakage, problems caused by microorganisms, food quality problems, and the detection of harmful substances in decoration materials.
Terahertz waves have great potential in high spectrum bandwidth, more information can be carried, low photon energy, and high security, which has the significant advantage of the future communication to achieve ultra-wideband and high rate [35]. Compared with microwave frequency communication, terahertz for high-speed communications application has a very rich frequency resource, has a workable bandwidth as high as dozens of GHz, and is the sixth generation of carrier wave communication technology.
In 2009, H.C. Liu group of the Institute of Microstructure Science of the National Research Institute of Canada built a THz communication system based on THz QCL and THz QWP and realized audio signal transmission at 3.8 THz frequency points. Other researchers also actively follow up the wireless communication project research based on THz QCL and THz QWP and carry out the demonstration experiment of using THz carrier to transmit analog audio signals with THz QCL as the transmitting source and THz QWP as the receiver. The frequency point of THz waves is 4.1 THz. The communication system using THz QCL and THz QWP as transmitter and detector, respectively, has great development potential.
Tan Zhiyong from SIMIT demonstrated a wireless transmission system of THz waves with a transmission distance of 2 m (Figure 8) and detailed the circuit design of the transmitter and receiver. The system uses THz QCL working in continuous-wave mode as the transmitter and THz QWP as the detector. By means of intensity modulation/direct detection, we use this system to transmit analog signals such as sine wave, triangle wave, and audio signal. The system is capable of transmitting signals at a −3 dB cutoff frequency of about 750 kHz. Theoretically, both THz QCL and THz QWP have modulation and detection bandwidths of tens of GHz if the circuit design and packaging of the devices are optimized. But in our experiment, the modulation bandwidth is mainly limited by the external modulation circuit and a detection circuit.
Gu Li et al. from SIMIT used THz QCL and THz QWP working in continuous mode to build the THz communication system, as shown in Figure 9. In this system, the digital signal to be transmitted from the signal generator is sent to the drive circuit to drive THz QCL, and the radiated THz light is transmitted through the optical path and is detected at the receiving end of the THz QWP. The THz QWP converts the received THz light into photocurrent of corresponding intensity, and the receiving end circuit amplifies the photocurrent and converts it into voltage signal, which is output in the oscilloscope.
FIGURE 9. THz communication experiment demonstration system [38].
The framework of the THz wave wireless communication system with transmission distance of 2.2 and 6 m, using THz QCL as transmitter and THz QWP as a detector, is presented in Figure 10. The experimental results show that the rate of the 2.2-m communication system is less than 16 Mbps and the bit error rate is 0. The rate is less than 22 Mbps, and the error rate is less than 10−3. The rate of the 6-m communication system is less than 8 Mbps, and the bit error rate of the transmission system is 0. The maximum transmission rate can reach 14 Mbps. Meanwhile, the OOK modulation real-time digital video transmission is realized by using the 2.2-m THz wireless communication link platform. The transmission rate is 5 Mbps, and the video signal transmission is stable. The video picture can be played smoothly on the display screen of both the transmitter and the receiver.
FIGURE 10. Video display at transmitter and receiver [39].
THz technology is a very important cross-cutting frontier, showing enormous potential application and practical value in astrophysics, plasma physics, spectroscopy, biology, medical imaging, environmental science, and other fields. In this article, the new terahertz detector, THz QWP, is reviewed. The development history, design principle, imaging application, and communication application of the detector are introduced. The THz QWP imaging system indicators are resolution, area, speed, and SNR. In the future, we can study the influence of off-axis parabolic mirrors with different parameters and sub-millimeter holes of different sizes on the spatial resolution of the system, so that the spatial resolution of the imaging system can break through the diffraction limit. In the mechanical scanning module, the accuracy of mechanical scanning can be optimized and the scanning range of mechanical scanning can be improved by controlling image distortion and improving the size of the imaging area. The imaging speed can be further improved by increasing the scanning speed and data acquisition speed. The SNR of imaging is mainly limited by the stability of the light path, stability of light source power, power of the light source, and sensitivity of the detector. Developing high-performance THz devices (lasers and detectors) to solve THz signal modulation and signal processing is the key to THz communication research. In the future, through the efforts of researchers, THz QWPs will become the mainstream detector of THz just as the corresponding band of QIWP and promote the development of THz technology and application.
DS proposed and wrote this manuscript; ZF, ZT, CW modified this manuscript; FQ, LG, WW performed the experiment; JC helped perform the analysis with constructive discussions.
This work was supported by the National Key R&D Program of China (2017YFF0106302, 2017YFA0701005, and 2018YFB1801502), the National Natural Science Foundation of China (Grant Nos. 61927813, 61975225, 61775229, 61704181, and 61875220), and the Fundamental Frontier Scientific Research Program of the Chinese Academy of Sciences (ZDBS-LY-JSC009).
The authors declare that the research was conducted in the absence of any commercial or financial relationships that could be construed as a potential conflict of interest.
All claims expressed in this article are solely those of the authors and do not necessarily represent those of their affiliated organizations, or those of the publisher, the editors, and the reviewers. Any product that may be evaluated in this article, or claim that may be made by its manufacturer, is not guaranteed or endorsed by the publisher.
1. Lee YS. Principles of Terahertz Science and Technology. Masayoshi Tonouchi, 2007 Nat Photon 1 (2009): 97. doi:10.1007/978-0-387-09540-0
2. Ferguson B, Zhang X-C. Materials for Terahertz Science and Technology. Nat Mater (2002) 1(1):26–33. doi:10.1038/nmat708
3. Schneider H, Liu HC. Quantum Well Infrared Photodetectors: Physics and Applications. Berlin: Springer (2006).
4. Cao JC. Semiconductor Terahertz Source Detector and Application. Beijing: Science Press (2012). p. 1.
5. Fu Z, Li R, Li H, Shao D, Cao J. Progress in Biomedical Imaging Based on Terahertz Quantum Cascade Lasers. China laser (2020) 47(2):0207014. doi:10.3788/cjl202047.0207014
6. Zhiyong T, Juncheng C. Photoelectric Characterization Technique Based on Terahertz Semiconductor Quantum-Well Devices and its Applications. China laser (2019) 46(6):0614004. doi:10.3788/cjl201946.0614004
7. Levine BF. Quantum‐well Infrared Photodetectors. J Appl Phys (1993) 74:R1–R81. doi:10.1063/1.354252
8. Liu HC, Buchanan M, Wasilewski ZR. How Good Is the Polarization Selection Rule for Intersubband Transitions. Appl Phys Lett (1998) 72(14):1682–4. doi:10.1063/1.121151
9. Liu HC, Song CY, SpringThorpe AJ, Cao JC. Terahertz Quantum-Well Photodetector. Appl Phys Lett (2004) 84(20):4068–70. doi:10.1063/1.1751620
10. Cao JC. Terahertz Semiconductor Detectors (In Chinese). Physics (2006) 35(11):953–956. doi:10.3321/j.issn:0379-4148.2006.11.013
11. Liu HC, Luo H, Song Cy., Wasilewski ZR, SpringThorpe AJ, Cao JC. Terahertz Quantum Well Photodetectors. IEEE J Select Top Quan Electron. (2008) 14(2):374–7. doi:10.1109/jstqe.2007.910710
12. Shao D, Guo X, Zhang R, Wang F, Fu Z, Wang H, et al. Influence of Many-Body Effect on Terahertz Quantum Well Photodetectors. Acta Opt (2017) 37(10):1004001. doi:10.3788/aos201737.1004001
13. Guo XG, Tan ZY, Cao JC, Liu HC. Many-body Effects on Terahertz Quantum Well Detectors. Appl Phys Lett (2009) 94(20):201101. doi:10.1063/1.3134485
14. Zhang R, Fu ZL, Gu LL, Guo XG, Cao JC. Terahertz Quantum Well Photodetectors with Reflection-Grating Couplers. Appl Phys Lett (2014) 105(23):231123. doi:10.1063/1.4904221
15. Zhang R, Shao DX, Fu ZL, Wang HX, Zhou T, Tan ZY, et al. Terahertz Quantum Well Photodetectors with Metal-Grating Couplers. IEEE J Select Top Quan Electron. (2017) 23(4):1–7. doi:10.1109/jstqe.2016.2608618
16. Abbott D, Zhang XC. Special Issue on T-Ray Imaging, Sensing, and Retection. Proc IEEE (2007) 95(8):1509–13. doi:10.1109/jproc.2007.900894
17. Hu BB, Nuss MC. Imaging with Terahertz Waves. Opt Lett (1995) 20(16):1716–8. doi:10.1364/ol.20.001716
18. Shi SC, Paine S, Yao QJ, Lin ZH, Li XX, Duan WY, et al. Terahertz and Far-Infrared Windows Opened at Dome A in Antarctica[J]. Nat Astron (2016) 1:0001. doi:10.1038/s41550-016-0001
19. Luukanen A, Appleby R, Kemp M, Salmon N. Millimeter-wave and Terahertz Imaging in Security Applications. In: KE Peiponen, A Zeitler, and GM Kuwata, editors. Terahertz Spectroscopy and Imaging. Berlin, Heidelberg: Springer (2012). p. 491–520. doi:10.1007/978-3-642-29564-5_19
20. Liu HC, Buchanan M, Wasilewski ZR. How Good Is the Polarization Selection Rule for Intersubband Transitions. Appl Phys Lett (1998) 72(14):1682–4. doi:10.1063/1.121151
21. Planken PCM, Bakker HJ. Towards Time-Resolved THz Imaging. Appl Phys A: Mater Sci Process (2004) 78:465–9. doi:10.1007/s00339-003-2405-0
22. Dean P, Valavanis A, Keeley J, Bertling K, Lim YL, Alhathlool R, et al. Terahertz Imaging Using Quantum cascade Lasers-A Review of Systems and Applications. J Phys D: Appl Phys (2014) 47(37):374008. doi:10.1088/0022-3727/47/37/374008
23. Chan WL, Deibel J, Mittleman DM. Imaging with Terahertz Radiation. Rep Prog Phys (2007) 70:1325–79. doi:10.1088/0034-4885/70/8/r02
24. Watanabe Y, Kawase K, Ikari T, Ito H, Ishikawa Y, Minamide H. Component Spatial Pattern Analysis of Chemicals Using Terahertz Spectroscopic Imaging. Appl Phys Lett (2003) 83:800–2. doi:10.1063/1.1595132
25. Dobroiu A, Yamashita M, Ohshima YN, Morita Y, Otani C, Kawase K. Terahertz Imaging System Based on a Backward-Wave Oscillator. Appl Opt (2004) 43:5637–46. doi:10.1364/ao.43.005637
26. Tan Z, Li H, Wan W, Fu Z, Wang C, Cao J. Direct Detection of a Fast Modulated Terahertz Light with a Spectrally Matched Quantum‐well Photodetector. Electron Lett (2017) 53(2):91–3. doi:10.1049/el.2016.3099
27. Qiu F, Tan Z, Fu Z, Wan W, Li M, Wang C, et al. Reflective Scanning Imaging Based on a Fast Terahertz Photodetector. Opt Commun (2018) 427:170–4. doi:10.1016/j.optcom.2018.06.030
28. Qiu FC, Fu YZ, Wang C, Tan ZY, Fu ZL, Wan WJ, et al. Fast Terahertz Reflective Confocal Scanning Imaging with a Quantum cascade Laser and a Photodetector. Appl Phys B (2019) 125(5):86. doi:10.1007/s00340-019-7198-8
30. Qiu FC. Fast Microscope Research Based on Terahertz Quantum Devices [D]. University of Chinese Academy of Sciences (2020).
31. Fu ZL, Gu LL, Guo XG, Tan ZY, Wan WJ, Zhou T, et al. Frequency Up-Conversion Photon-type Terahertz Imager. Sci Rep (2016) 6:25383. doi:10.1038/srep25383
32. Hou D, Li X, Cai J, Ma Y, Kang X, Huang P, et al. Terahertz Spectroscopic Investigation of Human Gastric normal and Tumor Tissues. Phys Med Biol (2014) 59(18):5423–40. doi:10.1088/0031-9155/59/18/5423
33. Shiraga K, Ogawa Y, Suzuki T, Naoshi K. Characterization of Dielectric Responses of Human Cancer Cells in the Terahertz Region. J Infrared, Millimeter, Terahertz Waves (2014) 35(5):493–502. doi:10.1007/s10762-014-0067-y
34. Wallace VP, Fitzgerald AJ, Pickwell E, Richard J. Pulsed Spectroscopy of Human Basal Cell Carcinoma. Appl Spectrosc (2006) 60(10):1127–33. doi:10.1366/000370206778664635
35. Kleine-Ostmann T, Nagatsuma T. A Review on Terahertz Communications Research. J Infrared Milli Terahz Waves (2011) 32(2):143–71. doi:10.1007/s10762-010-9758-1
36. Chen Z, Tan ZY, Han YJ, Zhang R, Guo XG, Li H, et al. Wireless Communication Demonstration at 4.1 THz Using Quantum cascade Laser and Quantum Well Photodetector. Electron Lett (2011) 47:1002. doi:10.1049/el.2011.1407
37. Chen Z, Cao J-C. Channel Characterization at 120 GHz for Future Indoor Communication Systems. Chin Phys. B (2013) 22:059201. doi:10.1088/1674-1056/22/5/059201
38. Zhen Chen ZC, Li Gu LG, Zhiyong Tan ZT, Chang Wang CW, Juncheng Cao JC. Real-time Video Signal Transmission over a Terahertz Communication Link. Chin Opt Lett (2013) 11:112001–3. doi:10.3788/col201311.112001
39. Gu L, Tan ZY, Wu QZ, Wang C, Cao JC. Twenty Mbit/s Wireless Communication-Demonstration Using Terahertz Quantum Devices. Chin Opt Lett (2014) 12:120401. doi:10.3788/col201513.081402
Keywords: terahertz detection, terahertz imaging, terahertz communication, terahertz, terahertz quantum-well photodetector
Citation: Shao DX, Fu ZL, Tan ZY, Wang C, Qiu FC, Gu LL, Wan WJ and Cao JC (2021) Research Progress on Terahertz Quantum-Well Photodetector and Its Application. Front. Phys. 9:751018. doi: 10.3389/fphy.2021.751018
Received: 31 July 2021; Accepted: 20 September 2021;
Published: 15 November 2021.
Edited by:
Xinke Wang, Capital Normal University, ChinaReviewed by:
Qiye Wen, University of Electronic Science and Technology of China, ChinaCopyright © 2021 Shao, Fu, Tan, Wang, Qiu, Gu, Wan and Cao. This is an open-access article distributed under the terms of the Creative Commons Attribution License (CC BY). The use, distribution or reproduction in other forums is permitted, provided the original author(s) and the copyright owner(s) are credited and that the original publication in this journal is cited, in accordance with accepted academic practice. No use, distribution or reproduction is permitted which does not comply with these terms.
*Correspondence: Juncheng Cao, amNjYW9AbWFpbC5zaW0uYWMuY24=
Disclaimer: All claims expressed in this article are solely those of the authors and do not necessarily represent those of their affiliated organizations, or those of the publisher, the editors and the reviewers. Any product that may be evaluated in this article or claim that may be made by its manufacturer is not guaranteed or endorsed by the publisher.
Research integrity at Frontiers
Learn more about the work of our research integrity team to safeguard the quality of each article we publish.