- Faculty of Physics and Earth Science, Peter Debye Institute of Soft Matter Physics, Biological Physics Division, University of Leipzig, Leipzig, Germany
Cell migration and invasion play a role in many physiological and pathological processes and are therefore subject of intensive research efforts. Despite of the intensively investigated biochemical processes associated with the migration and invasion of cells, such as cancer cells, the contribution of mechanobiological processes to the migratory capacity of cells as well as the role of physical polymeric phase transitions is not yet clearly understood. Unfortunately, these experiments are not very informative because they completely disregard the influence of the three-dimensional cell environment. Despite this data situation, it was possible to adequately demonstrate that there exists a direct mechanical interplay between cells and their microenvironment in both directions, where both elements can be mechanically altered by one another. In line with these results, it has turned out that the mechanobiological molecular processes through which cells interact with each other and additionally sense their nearby microenvironment have an impact on cellular functions such as cellular motility. The mechanotransduction processes have become the major focus of biophysical research and thereby, diverse biophysical approaches have been developed and improved to analyze the mechanical properties of individual cells and extracellular matrix environments. Both, the cell mechanics and matrix environment mechanics regulate the cell migration types in confined microenvironments and hence it seems to be suitable to identify and subsequently present a common bidirectional interplay between cells and their matrix environment. Moreover, hallmarks of the mechanophenotype of invasive cells and extracellular matrices can be defined. This review will point out how on the one hand the intracellular cytoskeletal architecture and on the other hand the matrix architecture contribute to cellular stiffness or contractility and thereby determines the migratory phenotype and subsequently the emergence of a distinct migration mode. Finally, in this review it is discussed whether universal hallmarks of the migratory phenotype can be defined.
1 Introduction to Cells and Their Environment
Specific migratory phenotypes are exhibited by cells and the speed of migration and invasion can be dynamically adapted to the physical characteristics of their microenvironment [1,2]. Thereby the physical constraints, cell adhesion, matrix rigidity and topology are key issues of the extracellular matrix environment that consequently impact the migratory capacity of cells [3,4]. It has been seen that the mechanical properties of cells contribute to their migratory capacity and seem to determine their migration mode. The mechanical properties of cells define their overall mechanophenotype. Similar to cells, the extracellular matrix mechanical properties can also define the matrix mechanophenotype of the microenvironment of cells and tissues. Since mechanophenotypes may be addressed in a more quantitative and comparable manner compared to shape or structural phenotypes, they seem to be more suitable to determine the migratory capacity of cells or collections of cells. However, it is still unclear whether the mechanophenotype of the cell relies on the mechanophenotype of the matrix and adapts accordingly to changes in the mechanophenotype in the environment. Eukaryotic cells promote the migration and invasion through linkage of the intracellular force and the actin cytoskeleton toward the microenvironment. Whereas the force coupling mechanics employed by mesenchymal migrating cells is generally conducted through transmembrane adhesion receptors, such as primarily those of the well-known integrin family, amoeboid cells, such as leukocytes can manage to move with highspeed, since there exists very weak cell-matrix adhesion forces [5,6]. Living organisms are subjected to a broad array of mechanical stimuli on different length scales, which cover universal forces, such as gravity, and microscopically localized forces, such as fluid shear stress within blood vessels [7], compression through adjacent tissues [8,9] or the rigidity of the extracellular matrix scaffold [10]. Specifically, it can be inferred that the displacement of the balance equilibrium among actin protrusion, actomyosin contraction, and adhesion to the extracellular substrate can account for the diverse modes of amoeboid locomotion, and the fact that blebbing and gliding are scarcely extreme varieties of a commonly adopted migratory pattern [5].
When enclosed in three-dimensional (3D) environments, cells exploit the topographic characteristics of the subsurface to move around. In particular, the retrograde flow of the actin cytoskeleton tends to have a pattern that closely matches the texture of the substrate, generating retrograde shear forces that are adequate to propel the cell body onward. It is noteworthy that adhesion-dependent and adhesion-independent migration are not necessarily contradictory, and instead are versions of the identical principle of linkage of retrograde actin flow to the surroundings, and thus can operate prospectively and interchangeably at the same time [6]. Univariate maps and phase diagrams provide an insight into how physical characteristics impact cell migration. Moreover, the phase transition may offer a simple explanation for a phase shift within cell populations, such as the unjamming-jamming phase transition or epithelial-mesenchymal transition [11]. Computational modeling allows systematic reconnaissance of the phase space to emphasize strategies for experimental investigations [1]. The phase transition may serve as a mechanical hallmark for cancer and its malignant progression.
2 Cells Impact Their Microenvironment
There are commonly physiological and pathological processes where the migration and invasion of cells plays a crucial role. Cancers develop to handle environmental stress or face and overcome all kinds of challenges, including nutrient deficiencies, lack of survival factors, and out-of-balance mechanical forces. The runaway growth and anomalous deregulation of central homeostatic cellular tracts resulting from genetic mutations establishes a stressful milieu [11]. Adjustments of cancers to the evolving surroundings can cause alterations in the motility engine of cells that impact migration, invasion, and metastasis. Cancer cells may enter singly or in groups, or may be ejected out of surrounding epithelium. These mechanisms are assumed to represent modulations of normal events that arise either in the course of development or tissue repair or in inflammatory responses [3,11]. The Plausibility of Life [12], which posits that every system in a cell or organism has developed to incorporate built-in distinct moduli of variability that can be triggered under stress or in the light of new capabilities, seems to be applicable for cells that need to adapt to the environmental cues. In turn, cells can alter the extracellular matrix surroundings, which may represent one such mechanism of adaption. Thereby, the cells can either secrete matrix metalloproteinases (MMPs), such as MT1-MMP toward their local environment [13], expresses sheddases on their cell surface or release matrix crosslinking molecules or secrete growth factors, cytokine or matrix-degrading enzymes regulatory molecules that are stored within the microenvironmental cavities.
Cancer cells overcome steric obstacles that represent enormous tissue barriers through the exertion of small actin-rich membrane protrusions, which are referred to as invadopodia. These invadopodia play a role in many steps of the metastatic cascade (Figure 1). The full maturation of invadopodia relies on extrusion and elongation of protrusions and the timed release of the matrix metalloproteinase MT1-MMP through endosomal trafficking involving unidentified mechanisms. The endoplasmatic reticulum (ER) protein protrudin can be demonstrated to foster the maturation and function of invadopodia. Protrudin assembles interaction sites for MT1-MMP-positive endosomes that are filled with the RAB7-binding kinesin-1 adaptor FYCO1 [14]. However, the lack of RAB7, FYCO1, or protrudin impaired the MT1-MMP-dependent extracellular matrix break-down and consequently, the invasion of cancer cells through blockage of the anterograde translocation and the release MT1-MMP through exocytosis [14]. In the event that endosome translocation or exocytosis has been hindered through depletion of protrudin or synaptotagmin VII, respectively, the invadopodia failed to extend and lengthen. In contrast, when protrudin has been overexpressed, noncancerous cells exhibited prominent invadopodia-like protrusions and displayed enhanced levels of matrix breakdown and elevated invasion.
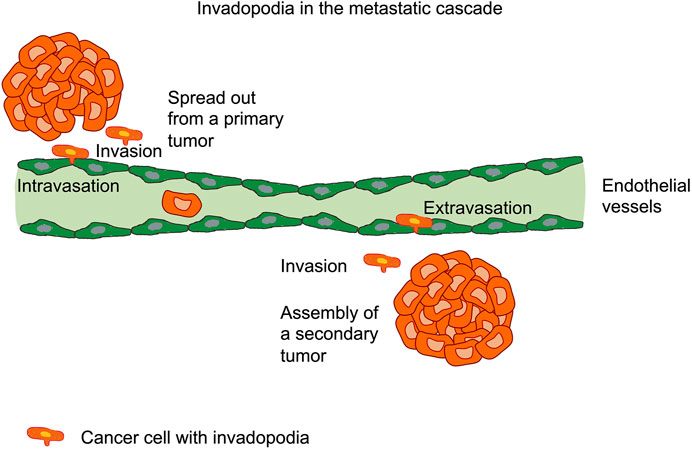
FIGURE 1. Cancer cells form invadopodia in many steps of the metastatic cascade, such as the spreading out of cancer cells from the primary tumor, the invasion through the extracellular matrix tissue environment, the intravasation through the endothelial cell layer into vessels, such as blood or lymphoid vessels, the extravasation of cancer cells through the endothelium and subsequent invasion through the extracellular matrix of targeted tissue. Finally, secondary tumors can be assembled and the metastasis cascade is completed.
Stabilization of progenitors promotes the maturation of invadopodia, which proceeds by a dual-track mechanism. On the one hand, actin polymerization and cortactin-dependent ramification permit the extension and elongation of the invadopodium. There is on the other hand fusion of MMP-containing vesicles with the plasma membrane of invadopodia, which results in the breakdown of the extracellular matrix. Curiously, both stages of invadopodia maturation abide on membrane plasticity and vesicle trafficking. While lysosomes have been proposed to act as membrane feeders for invadopodium outgrowth [15], late endosomes and lysosomes (henceforth collectively designated LE/Lys) have an well-established function in the supply of transmembrane (MT)1-MMP, synonymously referred to as MMP14, to the plasma membrane of invadopodia [16]. Thus, protrudin-facilitated ER-endosome contact sites encourage the invasion of cells through driving the translocation of MT1-MMP-laden endosomes toward the plasma membrane, which then fosters the outgrowth of invadopodia outgrowth and the exocytosis of MT1-MMPs.
Evidence suggests that invasive cancer cells overcome these tissue barriers producing specialized F-actin-based protrusions referred to as invadopodia, which focally breakdown the extracellular matrix to accommodate cell invasion [17–19]. In light of this, MT1-MMP is highly expressed within invadopodia and is found to be a key component of pericellular matrix breakdown, which labels the invasion of carcinoma cells across the basement membrane and through dense, collagen-rich tissue confinements [20–24]. While by definition all invadopodia types break down the matrix and are dependent on the catalytic activity of MT1-MMP, their composition and activity may vary according to the molecular composition and mechanical characteristics of the matrix microenvironment [25–27]. In the conventional model employed to examine invadopodia assembly, cancer cells are seeded atop a thin quasi-2D substrate of denatured collagen, such as gelatin, where deterioration activity is focused within 0.5–1 μm-sized, actin-rich spots [18]. In contrary, when confronted with a truer physiological matrix construction of collagen type I fibers [24,28], cancer cells form cortactin/F-actin-positive constructs that continue to mature into breakdown-competent invadopodia, together with focal MT1-MMP shedding and aggregating in conjunction with the subjacent matrix of collagen fibers [16,26,29,30]. Typically, these structures, extended in the plane of the plasma membrane, may be multiple micrometers long [26,29,30]. It has been seen that cancer cells penetrating through the collagen gel with a nucleus-at-the-back conformation [31,32], favorably develop prolonged invadopodia at the advance level of the invasive protrusion in front of the nucleus and dismantle the matrix-constricting fibers to aid in invasive pathway formation [24,28,33].
However, another more common mechanism of cell adaption may either alter their own mechanical characteristics or induce a phase separation due to modified assemblies of proteins.
Fundamental concepts of cell locomotion have been first uncovered in the 1970s [34–36]. Specifically, the 2D locomotion of fibroblasts that migrate over a flat substratum have been examined. Nevertheless, cell migration on the 2D surface insufficiently mirrors cell migration in vivo, as the cells are permanently subjected to the physical restraints of the extracellular matrix [13]. The fundamental stages of cell migration through the extracellular matrix can be identified by the three-stage model of invasion [37]. According to this theory, during migration, cells first acknowledge the extracellular matrix and adhere to it by adhesion molecules such as integrins [37]. Subsequently, proteinases are conscripted to induce local breakdown of the extracellular matrix. Ultimately, the cell body relocates to the deteriorated extracellular matrix cavity [37]. Repeating the three steps effectively empowers the cells to traverse over the extracellular matrix. This type of migration is termed mesenchymal type and demands the harmonious orchestration of integrin-facilitated cell adhesion, cytoskeletal rearrangement, and proteinase activity [13]. In reaction to the presence of sufficiently large, porous matrices, cells can also embrace a protease-independent regime of movement commonly referred to as amoeboid locomotion [38]. However, when cells break down extracellular matrix moieties and need to find a pathway to migrate, they engage MMPs, a set of proteinases that feature prominently in the breakdown of the extracellular matrix [39,40].
2.1 Cells Act on the Extracellular Matrix
Apart from biochemical signal transduction processes, cellular functionality and fate critically rely on the mechanophenotype of the surrounding extracellular matrix environment [2,3,41]. The extracellular matrix represents an acellular element of tissues that builds a matrix scaffold for the adhesion of cells and fosters multiple mechanotransduction events, which play a role in physiological processes encompassing morphogenesis and homeostasis [42]. Alterations of the mechanical characteristics of the extracellular matrix in in vivo and in vitro models through reimplanting tissues or alterations of the rigidity of the adherent substrate seem to be relevant in the reversion of the aging process [43], enhance developmental events [8] or modify the malignancy of cancers [44,45]. Restructuring of extracellular matrix scaffold and their compositional proteins seems to be relevant for the organization of entire tissues and organs. In this light, it is essential to gain an insight into the cellular and molecular stages that occur when individual collagen fibers move [46]. The movement of single collagen fibers may be either a cause of cell movement in 3D confinement or a prerequisite for the cell movement by deposition of misplaced collagen fibers (Figure 2).
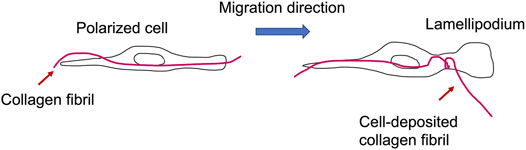
FIGURE 2. Migrating cells can bend and deposit a collagen fibril during the formation of lamellipodia in the course of cell migration.
2.1.1 Effects of the Dimensionality Affects Transportation of Collagen Fibers
The dimensionality of the cell culture systems impacts the mechanism of collagen fiber transportation. In specific detail, cell propagation on glass and other flat 2D substrates seems not to be strictly involved in the motion of fibers in 3D matrices. A limited level of crossover is seen between proteins resident at 3D matrix interfaces and those incident at 2D adhesions [47,48]. Specifically, the shape of fibroblasts in a 3D collagen matrix shares scant similarity with their morphology on a 2D collagen-coated interface [49,50]. Therefore, an essentially alternative mode of motility exists on 3D fibers in comparison to 2D surfaces [4,51,52]. Precisely regulated motions of actin and myosin filaments form the basement of multiple migration events of cells [53–55]. In line with this, it has been proposed that the cell locomotion system creates the cortical cytoskeleton. Due to coupling with extracellular matrix proteins, integrin receptors trigger an intricate signal transduction cascade leading to the activation of contraction through motor proteins empowering cell migration [56]. Thereby, the integrin–extracellular matrix interferences connect physically the extracellular microenvironment with the cell’s actin cytoskeleton and consequently couples it to microtubules and intermediate filaments. It has been explored what type of myosin and signal transduction pathways are engaged. In general, non-muscle myosin II (NM II) represents an actin-binding protein that can crosslink actin filaments and obeys contractile characteristics and its regulation is performed through the phosphorylation of its light and heavy chains. Specifically, myosin molecules are capable of moving longitudinally along actin filaments, driving their sliding or creating tension on them. While this demands energy supplied through the hydrolysis of ATP, it also necessitates that myosins contain catalytic sites possessing ATPase activity.
Apart from the linkage through integrins and the extracellular matrix scaffold, zyxin can localize to and dissociate from focal adhesions due to forces imposed by the extracellular matrix scaffold [57]. In contrast, the focal adhesion kinase (FAK), a prominent adhesion-associated tyrosine kinase, is required for durotaxis, which is based on the tendency of cells to move toward more rigid substrates [58]. Hence, these two molecules play also a crucial role in the process of cell migration and force generation [59–61].
Lack of non-muscle myosin heavy chain II (NMHC II) in cells does not impact numerous motile events, such as 2D migration on glass, however, it causes a reduction of the cell’s capacity to generate large forces [62,63]. Since large forces are commonly associated with the restructuring of collagen scaffolds, NMHC II seems to play a role in this process [64,65].
The remodeling of collagen carried out by fibroblasts fulfills a prominent function in the optimization of tissue architecture, which is fundamental for motility in the course of wound healing, developmental processes and the control of cell growth. Nevertheless, the mechanism of collagen fiber locomotion in 3D matrices has not been fully elucidated. Fibroblast lamellipodia project along retained collagen fibers, engage them, and retract them in a “hand-over-hand” cycle engaging α2β1-integrin [46]. Wild-type fibroblasts propel collagen fibers three to four times farther in each cycle than fibroblasts deficient in myosin II-B, referred to as myosin II-B−/−. In a similar manner, myosin II-B−/− fibroblasts shrink 3D collagen gels threefold less compared to controls. Nevertheless, on 2D substrates, the propagation rates of collagen beads and cells are not influenced by loss of myosin II-B. Green fluorescent protein (GFP)-labeled myosin II-B, while not myosin II-A, re-establishes the normal function in knockout cells and becomes locally distributed at cell processes, whereas myosin II-A is distributed rather at a central location. In this regard, GFP myosin II-B travels to the peripheral region and returns to the central region during hand-over-hand fiber locomotion, while on 2D collagen, myosin II-B tends to be more centrally dispersed. Consequently, it has been hypothesized that a cyclic formation of myosin II-B and contraction within lamellipodia foster the locomotion of 3D fibers [46].
NMHC II is instrumental in the contractility of actin upon migration, cytokinesis, and formation/sustainment of the cell shape. There exist three isoforms of NMHC II in mice and humans, NMHC II-A, II-B and II-C [66–68] that exhibit various expression profiles [67]. Even though NMHC II-A and NMHC II-B exhibit 85% amino acid sequence identity within the motor domain, they seem to have nonoverlapping distinct functions [69–71]. Ablation of NMHC II-B is embryonically fatal in mice due to serious defects in the heart and brain [72,73]. NMHC II-B−/− fibroblasts that express NMHC II-A but not II-C have normal appearance on tissue culture plastic and other 2D supports, although they display more haphazard locomotion and marginally elevated on-momentum motion rates [46,74,75].
NMIIA is linked to complement receptor-driven but not FcγR-driven phagocytosis, which has been suggested to engage the polymerization of actin [76]. NMIIA filaments may also coordinate phagocytosis [77] through either fostering the depolymerization of cortical actin [78] or its fluidization [79]. NMIIA filaments resemble exactly toward collagen adhesion sites and are needed for interference and capture of the small GTPase Rap1 within focal adhesions [80,81], which is critical for governing the activation of β1 integrins [82] and, correspondingly, for the phagocytosis of collagen. All of which has been revealed through siRNA-knock-down of NMIIA [81]. Apart from collagen remodeling through phagocytosis, there exists another collagen remodeling process that seems to be based on collagen transportation.
To enlighten the last process of collagen remodeling, single fibers of Cy5-labelled or TAMRA-labelled collagen type I can be positioned on top of the apical plasma membrane of adherent cells. The labeling of collagen fibers provided the opportunity to monitor individual cells that interfere with individual collagen fibers, and record the local collagen displacement or transport, whereby the capacity of cells to adhere to or act on fluorescent collagen fibers is not altered [46,83]. It has been reported that the displacements of individual collagen fibers are subject to the cycles of cell elongation, retraction, and liberation at the anterior surface of a polarized cell [46].
When examining the movement of the cell edge and the fiber displacement over time, it has been seen that the fiber is stalled upon the cell extension at the cell’s leading edge. When the lamellipodium expanded parallel to the fiber, the contact is maintained and no motions in any other direction can be seen. After the extension phase in the range of 20–40 s, the lamellipodium pulled back; concurrently with this contraction, the fiber traveled inwards at the identical velocity as the leading edge. After the fiber has been carried into the endoplasmic region, it became liberated from the cell. While this behavioral rhythm pattern of motility in 3D is akin to the cycles of cell motility observed in 2D models [53], it is distinct from 2D motions [46]. The behavioral pattern of fiber motions through the cell is intermittent; the lamellipodium stretches along a retained fiber, attaches, and pulls backward in a set of distinctive steps that is what is referred to as an event. Several events appear hand in hand and produce large fiber dislocations. Therefore, the main mechanism for collagen remodeling entails a sequence of elongation/retraction events propelled through lamellipodia. To quantify fiber motion, the frequency of remodeling events for each cell, the speed and duration can be determined. The location of the fibers has been monitored over time that leads to displacement over time curves, which can be subdivided into distinct individual events, The events start when the fibers initiate their movements, and are terminated when the movements are stalled. From the collagen fiber displacements, it can be deduced that wild-type cells exhibit multiple high velocity phases and display long duration periods.
Since the fiber motion seem to rely on NMHC II-B, the fiber movements have been determined in NMHC II-B−/− fibroblasts and wild-type controls, whereby the knock-out cells exhibited less motion. Employing a more precise quantitative examination of the events pointed out that the duration and velocity of fiber motion is reduced that leads to the reduction of displacements for each event appearing subsequently at a lower frequency in NMHC II-B−/− compared to NMHC II-B+/+ cells. Restoration of NMHC II-B content in NMHC II-B−/− cells through transient or stable transfection with GFP-NMHC II-B [84] augmented fiber shift per event; however, expression of GFP-NMHC II-A failed to increase the fiber shift per event.
To assess whether reduced fiber motion at the single-cell level mirrors the capacity of 3D collagen matrix remodeling, the contraction of collagen matrices through a suspension of a standard amounts of fibroblasts has been determined. Wild-type cells can contract the matrix to about 25% of the original area, whereby NMHC II-B−/− cells solely to about 75% original area. Stable transfection of GFP–NMHC II-B is able to rescue capability of the NMHC II-B−/− cells to contract the matrix, while transfection of GFP–NMHC II-A cannot restore it. This finding indicates that NMHC II-B is necessary for the contraction of collagen matrices, while NMHC II-A is not required. However, NMHC II-A possesses a different distribution from NMHC II-B with merely little colocalization of both.
To move collagen fibers, cells must bend them, which necessitates a pronounced force [70], and fibers under tension experience the same type of movement. The high force demand and failure of NMHC II-A to balance the loss of II-B is in accordance with the higher pulse duty cycle of NMHC II-B (F. [71]). Consequently, the reduction of fiber trafficking through the hand-over-hand cycle and 3D matrix contraction in NMHC II-B−/− cells seems to directly rely on the lack of NMHC II-B. Thereby, it has been ruled out that the divergent effects of the two cell types are based on different adhesion strength toward collagen matrices through the binding to the α2β1 integrin [49,85]. Moreover, the expression of α2β1 integrin is not challenged in the two cell types. Therefore, NMHC II-B is intimately affiliated with collagen contact points in 3D matrices, traveling into the extending lamellipodia and migrating posteriorly in concert with actin back transport [53–55] as the fiber contracts.
Finally, the process of collagen fiber motility has been shown to entail repeated hand-to-hand retraction of the fiber by parts of the cell lamellipodia. Lamellipodia elongate whereas the fiber is steady-state, and fiber trafficking tends to substantially correspond to lamellipodia retraction. Even though this is parallel in multiple ways to the process of cell movement on glass [86], the two processes differ significantly in their reliance on NMHC II-B.
It has been figured out that contractility of cells may be related with specific localization of NMHC II-B, which is almost distinct from the localization of NMHC II-A [87–89]. This discrepancy in their specific localizations may be attributable to their different behavior within various organisms or cell types that possess different integrin types, undergo different phases of motility or are either cultured in a 2D environment or within a 3D matrix. There is an increasingly agreement on the fact that cellular interplay inside 3D matrices is pronouncedly diverse compared to traditional cell cultures on top of 2D substrates. In specific, when cells are seeded on pure coverslips for 18 h, NMHC II-A can be found at the edges of polarized cells, while NMHC II-B is located farther at the rear end of the cell [89]. However, when cells spread and polarize for solely 1.5 h on a dense 2D collagen matrix, the localizations are reversed. NMHC II-B−/− cells cannot compensate for defect of II-B through re-localizing II-A toward the periphery. Instead, II-A is located in the endoplasm. In line with this finding, transfected GFP–NMHC II-A and II-B proteins can be found to the identical regions as endogenous proteins. Finally, these results strongly point out toward a NMHC II-B-driven mechanism of collagen remodeling process.
When cells in 3D collagen matrices are grown, NMHC II-B can be detected in the periphery of the cell, and most prominent when thin, dendritic protrusions alongside collagen fiber interactions are exerted. However, NMHC II-A is still centrally focused. These findings further contribute the hypothesis that dimensionality impacts strongly the localization of NMHC II. Hence, it can be hypothesized that NMHC II-B is targeted toward cell–collagen adhesion sites through a process involving integrin activation via collagen.
Moreover, NMHC II-B dynamic response activity is found to be accompanied by episodes of cellular expansion and contraction. As the leading edge extended, NMHC II-B translocalizes in the lamellipodia. Directly before membrane retraction, ripples of NMHC II-B traveled posteriorly, while the collagen fiber underwent posterior traction. The process persisted all the way until the lamellipodia became fully retracted, at which point NMHC II-B started to be carried forwards back into the freshly expanded lamellipodia. Conversely, the dynamics of NMHC II-B in cells that moved on non-treated substrates appeared to be distinct. Cells plated on non-treated 2D glass substrates expanded and contracted lamellipodia ordinarily, but GFP-NMHC II-B remained invariably lacking in the expanding or contracting lamellipodia. The same pattern of GFP-NMHC II-B locus formation has been consistently seen in cells migrating within a 3D collagen matrix; however, cells migrating on 2D collagen fail to obey this type of pattern [46].
These findings provide evidence that NMHC II-B plays a dedicated, immediate role in the trafficking of collagen fibers through fibroblasts in the 3D matrix that it is distinctly dissimilar to its involvement in 2D substrate motility. A number of alternative explanations for the decline in fiber movement have been examined. To begin with, there has been no change in NMHC II-B−/− cell migration on 2D substrates [90,91] or in the rate of cell spreading on fibronectin. In addition, the existence of a collagen fiber on the top surface in no way modified the migration levels of NMHC II-B−/− and NMHC II-B+/+ cells on the medium. Lastly, the speed of the collagen-coated beads on the lamellipodia on control and NMHC II-B−/− cells remained the identical. Thus, the lack of collagen fiber and 3D cell motility in NMHC II-B−/− cells is by no means the consequence of a variation in 2D migration speed, backward transport of actin, or the spreading of lamellipodia on interfaces.
Motility in 3D collagen matrices has been found to be heavily reliant on NMHC II-B, while motion on 2D surfaces is unaffected. Because NMHC II-B−/− cells can move collagen spheres posteriorly on a 2D surface while not moving collagen fibers, the fiber architecture appears to be indicative of NMHC II-B engagement. These findings imply that NMHC II-B is substantial for correct cellular rearrangement of collagen matrices in vivo, which is in accordance with the aberrant cardiac performance in the knockout mouse [71]. While transfection of GFP-NMHC II-B into NMHC II-B−/− cells resembled and rescued the wild-type phenotype, which failed to do so when GFP-NMHC II-A has been expressed in these cells. This outcome offers complementary circumstantial support that NMHC II-A and II-B perform nonredundant functions in the production of contractile forces throughout the reorganization of the collagen matrix. The cycling of NMHC II-B motion into the lamellipodia, contraction, and breakdown to fuel the locomotion of collagen fibers is subject to a sophisticated, stepwise process to generate whole-cell motion in three dimensions.
2.1.2 Degradation of Collagen Fibers and Networks
A Disintegrin And Metalloproteinases (ADAMs) are a transmembrane protease family that function in the regulation of inflammatory reactions [92]. Emerging in their role in cardiovascular disease/atherosclerosis is that ADAM10 regulates the assembly of atherosclerotic plaques [93], whereas ADAM15 participates in plaque lesion evolution [94] and ADAM17 is linked to atherosclerosis resistance [95,96]. Of multiple members of this family, ADAM8 possesses sheddase activity that facilitates the scission of cell surface proteins related to atherosclerosis, such as the inflammatory adhesion receptor molecules L-selectin, P-selectin glycoprotein ligand-1 (PSGL-1), vascular cell adhesion molecule 1 (VCAM-1), tumor necrosis factor (TNF) and TNF receptor 1 [97,98]. ADAM8 is strongly expressed in the majority of cells of hematopoietic origin and also in brain, bone, lung and thymus [99–104]. Although ADAM8 expression is widespread, mice lacking ADAM8 have a normal evolution with no conspicuous phenotype [103]. In terms of pathologies, ADAM8 expression is raised in cancer and inflammatory diseases of the lung, central nervous system, bone, and joints, and its expression correlates strongly with the seriousness of disease [97,100,105,106].
Membrane type 1 matrix metalloproteinase (MT1-MMP) represents a type I transmembrane proteinase that is part of the matrix metalloproteinase (MMP) family. In fact, MT1-MMP is a powerful cellular microenvironment remodeler and enhances cell migration and invasion of a broad range of cell types under both physiological and pathological circumstances. Therefore, it encourages cell migration through breaking down the extracellular matrix on the cell surface and establishing a migration trail, through altering the characteristic of cell adhesion through shedding cell adhesion molecules to improve cell motility, and through modifying cellular consumption metabolism. Consequently, MT1-MMP is a multipurpose cell motility amplifier [107].
MMPs comprise a set of zinc-dependent metalloproteases that are capable of breaking down all constituents of the extracellular matrix. In humans, a total of 23 MMPs are available, and they can be categorized on the grounds of substrate sensitivity or domain architecture [108]. Optionally, they can be divided into soluble MMPs or membrane-type MMPs (MT-MMPs) (Figure 3). The characteristic domain structure of MMPs is composed of a signal peptide, a pro-domain, a domain of catalytic activity, a coupling peptide, also referred to as a hinge region, and a hemopexin domain [108] (Figure 3). The soluble MMPs are frequently released as inactive zymogens and have to be activated extracellularly by additional proteinases. MT-MMPs have a domain structure in common with other MMPs, but they are bound to the plasma membrane either by a transmembrane domain trailed with a small cytoplasmic tail (CT) (MT1-, MT2-, MT3-, and MT5-MMPs) or a glycosylphosphatidylinositol (GPI) anchor (MT4-, MT6-MMPs) at their C-terminus [109] (Figure 3). Intracellularly, all MT-MMPs are being activated through proprotein convertases such as furin and expressed as active species on the cell surface. The proprotein convertases identify and subsequently scission a basic amino acid motif of RX(K/R)R at the C-terminus of the pro-domain, which is shared between all MT-MMPs and some soluble MMPs such as MMP-11, -21, -23, and -28 [109]. Transmembrane-type MT-MMPs can also be identified as having an eight-amino-acid loop in their catalytic domain, referred to as MT-loop [109].
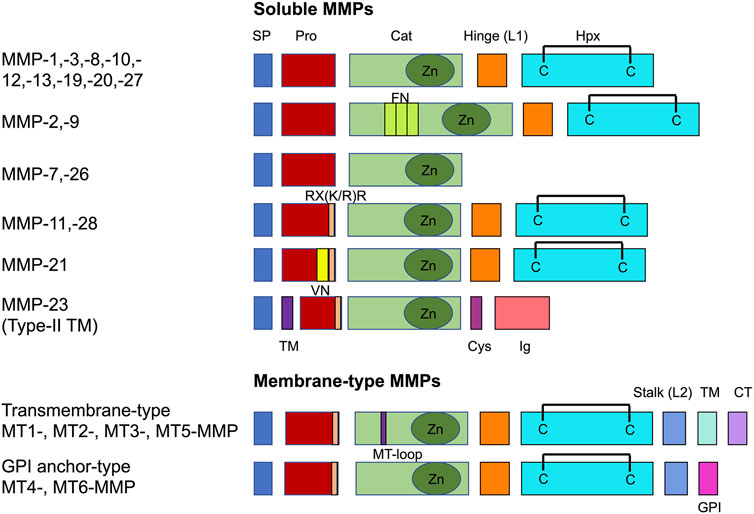
FIGURE 3. Common domain structure of the MMP family. There are two major classes, such as soluble MMPs and membrane-bound membrane-type MMPs. Catalytic domain (Cat), cysteine-rich domain (Cys), cytoplasmic tail (CT), fibronectin type II repeats (FN), GPI-anchor signal sequence (GPI), hemopexin domain with a C-C disulfide bond (Hpx), immunoglobulin-like domain (Ig), linker 1 (L1), linker 2 (L2), MT-loop = eight-amino acid insertion characteristic to TM-type MT-MMPs, pro-domain (Pro), RX(K/R)R = PC recognition sequence, signal peptide (SP), transmembrane domain (TM), vitronectin-type domain (VN).
Membrane type I MMP (MT1-MMP) has been found to be the unique MMP among these that enhances cell migration in a collagen-rich setting [21]. MT1-MMP-facilitated the migration and invasion of cells and hence have been associated with various disease processes, encompassing inflammation [110], atherosclerosis [111], rheumatoid arthritis [112], invasion of cancer and malignant progression of cancer, such as metastasis [113]. Consequently, insight into the mechanisms of MT1-MMP-directed cell migration/invasion is critical to gain an appreciation of the pathogenesis of various diseases. There is ample evidence that MT1-MMP enhances cellular invasion both in response to and independently of proteolytic activity [107]. In the following the three different mechanisms for the migration modes of MT1-MMP-dependent cell migration/invasion are presented and discussed. These three mechanisms comprise direct proteolytic extracellular matrix degradation, indirect extracellular matrix proteolysis and modification of cell adhesion molecules.
2.1.3 Cross-Linking of Fibers
The extracellular matrix contains primarily collagen type I fibers. Collagen-made scaffolds have turned out to function as ideal biomaterial for the purpose of tissue engineering and development of in vivo implants. For multiple biomedical techniques, collagen can be crosslinked to strengthen the strength, rigidity and stability of the overall mechanical construct. This can be done by chemical, biomaterial and cell-derived cross-linkers, however, for the in vivo situation, the cell-derived cross-linkers are relevant. The other cross-linkers, such as chemical substances, biophysical techniques and biomolecules from other species than the cells or tissues under investigation can solely be employed to mimic the in vivo situation in in vitro cell or tissue culture assays.
Collagen is omnipresent, self in tissues that are biologically and structurally diverse. The natural layering and positioning of cells in the body is controlled through biochemical and biomechanical indications of the extracellular matrix and the physiological circumstances at the location of the tissue. Whereas some of these indications can be customized in a framework through the use of additive fabrication, some changes result in a natural way from the decisions encountered during the process of synthesizing the nucleus of a framework. Therefore, the structural and property alterations imparted by the underlying manufacturing processes over the length scales simply are not possible to oversee. Specifically, the networking process not merely enhances the mechanical characteristics of a collagen skeleton, however, it also leads to additional alterations in the molecular framework. In the following seven most frequently employed substances for the cross-linking of collagen type I fibers are presented and discussed. There are three major classes of collagen cross-linkers, such as chemical, biomaterial and cell-derived cross-linkers. A special focus is placed on cell-derived cross-linkers, since they are present in vivo and possess no potential toxic effects on cells and tissues. However, for experimentalists the chemical cross-linkers and the techniques to cross-link extracellular matrices are important for future studies and are mentioned below. The biological cross-linkers may have the advantage that they are less toxic for the cells and tissues and hence they are included in this part.
2.1.3.1 Chemical Cross-Linkers
The basic goal of chemical crosslinking is to enhance the mechanical characteristics and stability of the processed collagen end product. The choice of a suitable cross-linker, by contrast, may vary according to a number of factors. Collagen is commonly utilized in biomedical implementations to mimic the biochemical environment encountered in vivo, so amine-based cross-linkers [114–118] are frequently selected to imitate the lysine-based linkages inherently occurring in collagen [119]. The mode of selection and mechanism of crosslinking may also unintentionally alter different structural properties and the relevant biological reaction. Chemical cross-linkers, for example, can be roughly categorized according to their ability to integrate the cross-linker straight into the protein. This yields the “zero-length” cross-linkers, where they do not stay within the protein structure after crosslinking, or the “non-zero-length” cross-linkers, where a portion or the entire cross-linker is built-in (Figure 4). Whereas zero-length cross-linkers can alter the local chemical composition, leading to non-native such as cellular interactions [120], it also raises some issues of concern regarding the specific nature of the potential for non-zero-length crosslinked collagen profiles to liberate cytotoxic compounds when subject to metabolism [121]. The main cross-linkers in use, most of which are amine-based and span a variety of cross-linkers with and without zero length [122]. Among them are conventional cross-linkers including glutaraldehyde, which is a non-zero length cross-linker, and 1-ethyl-3-(3-dimethylaminopropyl) carbodiimide-N-hydroxysuccinimide (EDC-NHS), ultraviolet radiation (UV), dehydrothermal treatment (DHT), that are zero length cross-linkers. New and upcoming crosslinking techniques involve the utilization of genipin as a non-zero length cross-linker, and riboflavin and tissue transglutaminase 2 (TG2) as zero length cross-linkers.
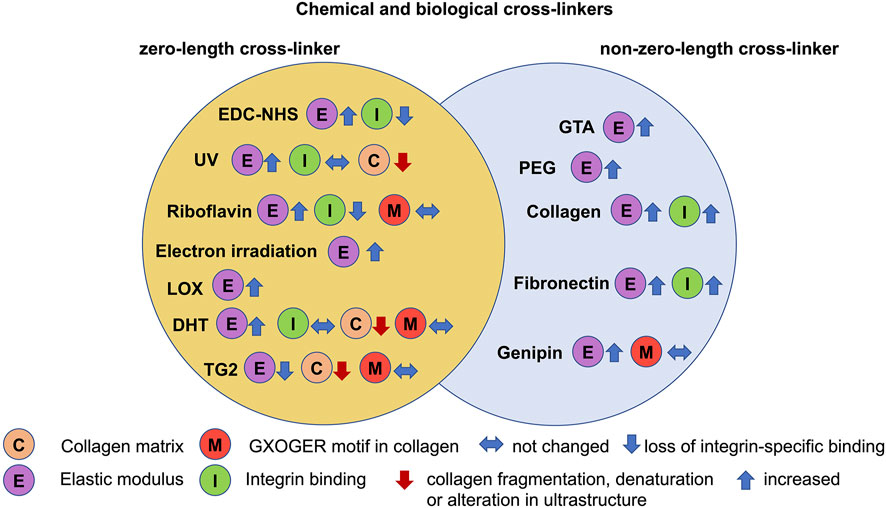
FIGURE 4. Chemical and biological cross-linkers serve as modifiers for 3D collagen matrices. Among them are zero-length (yellow circle), including 1-ethyl-3-(3-dimethylamino propyl) carbodiimide hydrochloride (EDC)-N-hydroxysuccinimide (NHS), ultraviolet radiation (UV), riboflavin, electron irradiation, dehydrothermal treatment (DHT), lysyl oxidase (LOX) and transglutaminase 2 (TG2) and non-zero-length cross-linkers (blue circle), such as glutaraldehyde (GTA), polyethyleneglycol (PEG), collagen, fibronectin and genipin.
2.1.3.1.1 Glutaraldehyde
Glutaraldehyde represents a chemical cross-linker that is frequently investigated in tissue engineering due to its exceptional capacity to increase the elastic modulus of scaffolds [123]. Glutaraldehyde utilizes the generation of imide as a mechanism to crosslink collagen fibers (Figure 4). As evident from the collagen interaction mechanism, glutaraldehyde is not a zero-length cross-linker and the final crosslinked collagen includes portions of the linker molecule in the resulting structure. Glutaraldehyde has also proven capable of attaining an unusually high level of crosslinking, with full (amine-based) crosslinking exceeding 0.12 wt% obtained in porcine dermal telocollagen-depleted collagen gels [124]. The concern with this, nonetheless, is that crosslinks can be formed both within and across collagen fibrils, and that an advance in crosslink density is not necessarily accompanied with a comparable rise in mechanical characteristics [116]. Glutaraldehyde crosslinking of dermal sheep collagen has been determined to raise the modulus at low strain due to crosslinking from 1.7 to 3.5 MPa at 0.5 wt/wt%, even though the modulus at high strain drops slightly from 32.7 to 21.0 MPa with rising crosslinking concentration [116]. In addition, cell sowing and proliferation has been found to be enhanced by glutaraldehyde crosslinking through hindering cell-mediated contraction of a rat tail atelocollagen-derived type I scaffold, whereas differentiation was markedly impeded compared with the noncrosslinked scaffolds [125]. The byproducts of breakdown in subsequent metabolic pathways also led to the cellular cytotoxicity seen, even though there is no indication of carcinogenicity or mutagenicity associated with the usage of glutaraldehyde [121].
2.1.3.1.2 1-ethyl-3-(3-dimethylaminopropyl) carbodiimide-N-hydroxysuccinimide (EDC-NHS)
EDC-NHS represents a general collagen cross-linker which has a non-cytotoxic, zero-length crosslinking alternative to glutaraldehyde. Zero-length cross-linking leads to restricted access to “adjacent” free amines [126], which obeys a reaction mechanism (Figure 4). The cross-links are between glutamates and arginines/lysines and in each case involve the GXOGER sequence detected by integrins acting to intercede cell binding on collagen, where the amino acid X is usually phenylalanine (F). The EDC-NHS is referred to as the standard concentration of 100% [117,127] and is frequently written as a precise molar ratio of 5:2:1 EDC:NHS:COO−, where COO− denotes the carboxylate groups in the protein.
EDC-NHS crosslinking provides a lower density of the crosslinks compared to classical cross-linkers such as glutaraldehyde. However, it delivers a more hydrophilic surface, which is favorable for fibronectin activity and enables a higher degree of swelling than traditional cross-linkers on type I bovine dermal swollen gel-derived collagen fibers, such as those exhibiting chondroitin-6-sulfate additives [126]. In addition, EDC-NHS has been demonstrated to trigger self-assembly of collagen bundles with a width of approximately 300 nm with both acid-soluble and insoluble type I bovine dermal collagen, implying the feasibility of both intra-fibril and inter-fibril binding, although it is a zero-length cross-linker. The non-soluble collagen fragments exhibited amplification and localization of the piezoelectric response alongside these self-assembled fiber bundles [128].
In the past, cross-linking of collagen up to 200%, synonymously referred to as 10:4:1 EDC:NHS:COO- of the standard composition, however, substantial research on EDC-NHS constitution and its impact on cell migration and mechanics [127,129–132] indicate that far lower concentrations (10–100%) can maintain the enhancements in mechanical characteristics imparted by crosslinking. Extruded collagen fibers, such as bovine dermal acid-swollen gel collagen type I, have been analyzed after crosslinking at three specific EDC-NHS concentrations (0.02 w/w EDC%-0.006 w/w% NHS, 0.002 w/w EDC%-0.0006 w/w% NHS and 0.0002 w/w EDC%-0.00006 w/w% NHS) [129] for alteration of the ultimate tensile strengths, which revealed to be not impacted through the levels of EDC-NHS cross-linker. Human tenocytes of the anterior cruciate ligaments can adhere to these extruded collagen fibers at lower levels, when these fibers are strongly crosslinked after 1 day [129]. After 3 weeks of culturing, the tenocyte proliferation on the heavily crosslinked fibers has been decreased. The results implicate that the crosslinking conditions can be lowered by roughly two orders of magnitude with no impact on the tensile characteristics [129].
Similar results of impaired attachment of C2C12 mouse cardiomyocytes, platelets and HT1080 fibrosarcoma cells have been obtained with the EDC-NHS cross-linker for collagen films, such as type I microfibrillar bovine dermal and Achilles tendon, whereas there is not effect for crosslinked gelatin films of bovine dermal sources [127,130]. The hypothesis connected the perceived decrease in cellular attachment to the ablation of GXOGER motifs in collagen by the carbodiimide crosslinking procedure. Based on the access of RGD motifs for cell binding in gelatin that is cryptic in collagen, similar reductions in cell attachment are not seen with crosslinked gelatin.
The impact of EDC-NHS crosslinking on integrin binding has been explored to obtain a mechanistic comprehension how the cross-linkers alter the collagen skeleton to generate the drop in cell adhesion [120]. Moreover, biochemical receptors involved in binding with the crosslinked type I collagen substrates of bovine Achilles tendon have been identified by analyzing the binding of two integrin I domains (α1 and α2) and classifying four different model cell lines, such as platelets, HT1080 human fibrosarcomas, Rugli rat glioma cells, and C2C12 mouse fibroblasts with transfected integrin I domains that express distinct collagen-binding integrins. Through isolated integrin domain binding and cellular attachment experiments to collagen, it has been revealed that four collagen integrins, such α1β1, α2β1, α10β1, and α11β1, are impacted through the EDC-NHS crosslinking. Thus, the mechanism has been proposed to delineate the inhibition of integrin binding through the engagement of glutamic acids in the crosslinks created by EDC-NHS. Because I-domain binding in an integrin relies on pairing with a divalent cation aided by metal ion dependent adhesion site (MIDAS) motifs, depletion of glutamates upon EDC-NHS cross-linking is hypothesized to ablate GXOGER motifs on collagen. At high EDC-NHS crosslinking (≥10%), the extent of GXOGER ablation evoked through the carbodiimide seems to facilitate non-native cellular interferences with the substrate [120].
2.1.3.1.3 Dehydrothermal Treatment
DHT utilizes LeChatelier’s principle of advancing a reaction accomplished through the removal of the crosslinking by-product, water, using heat and vacuum (Figure 4). The impact of DHT sustained at a variety of temperatures (110, 120, 140, 160, 160, 180°C) at 30–50 Torr for 24 h after a 1-h ramp has been examined on scaffolds of bovine type I dermal collagen. It can be seen that the compressive elastic modulus of DHT-treated films rose with the employed DHT temperature, in accordance with the interlacing density of the films [133]. The treated collagen films have also been prone to denaturation in the process, exhibiting a significant denaturation (57.84%), crumbling, and embrittlement at elevated temperatures (180°C) [133]. The crosslink density of DHT-treated collagen obtained from cow skin has been observed to rise with temperature from 105 to 125°C, although not with time beyond 3 days [134]. Even though some denaturation of collagen is claimed to enhance crosslink density by providing physical adhesion to hidden groups, beyond 145°C or 5 days, a significant amount of denaturation and diminished mechanical characteristics have been noted [134].
An in vivo evaluation of DHT-treated microfibrillar type I collagen-chondrotin-6-sulfate scaffolds has been performed within transected peripheral rat nerves [135]. Thereby, the treatments at higher times and temperatures aided nerve regeneration more effectively, offering an optimal time frame for breakdown that corresponded to the tissue regeneration speed. In addition, an examination of the in vitro cell reaction of DHT-treated type I collagen-choindroitin-6-sulfate collagen scaffolds has been performed employing MC3T3-E1 mouse preosteoblasts at higher temperatures. Specifically, these scaffolds have been modified with four temperatures, such as 105, 120, 150 and 180°C, under a vacuum of 37.5 Torr. DHT-treated scaffolds exhibited both extreme priming and proliferation of preosteoblasts when compared to EDC-NHS or glutaraldehyd-cross-linked scaffolds, which is especially seen at the higher temperature of 150°C [131]. Although the findings of an in vivo and an in vitro assay cannot be directly matched, it is reasonable to assume that the utilization of DHT treatment to evoke high cellular activity can be restricted to lower temperature regimens at which the chance of denaturation is low.
2.1.3.1.4 Ultraviolet Radiation
The utilization of UV to crosslink collagen is a fairly new technique that is based on the generation of highly reactive radicals to assist in the creation of crosslinks throughout the microstructure (Figure 4). Due to the nonspecificity of radical-based reactions, no rigorous chemical mechanism exists, although amino acid analyses indicate cross-links through aromatic residues, such as phenylalanine and tyrosine [127,136], which may act to stabilize the radicals inside their delocalized π-systems.
UV-irradiated films of acid-soluble rat tail tendon collagen exhibit a decrease in thermal stability and generate of surface flaws, such as wrinkles and micro-cracks upon UV crosslinking at λ = 254 nm, 0.196 J cm−2min−1, for 2, 4 and 8 h [137]. It was hypothesized that the applied UV radiation breaks the hydrogen bonds within and transverse to the collagen in these samples, initiating the liberation of water and the creation of collagen fragments [137]. The UV-dependent fragmentation of collagen has been demonstrated to be restricted through the addition of glucose that impairs the unwrapping of the triple helices in bovine insoluble dermal and Achilles tendon collagen polymers [138].
An evaluation of the physical characteristics of UV-irradiated collagen films and scaffolds in the presence and absence of glucose has been carried out [127]. Bovine type I skin scaffolds and bovine Achilles tendon collagen scaffolds and films have been prepared and subsequently exposed to various UV treatments, such as λ = 254 nm for 30 min with a defined spectrum of intensities ranging from 0.06 to 0.96 J cm−2 for scaffolds and either 0.42 or 0.96 J cm−2 for films. The crosslink strengths attained remained extremely low regardless of the extended exposure times, resulting in a maximum Young’s modulus of less than 2 kPa for the glucose-treated tendon collagen, and 0.5 kPa in the absence of any supplements [138]. In addition, the effect of UV crosslinking on breakdown resistance in water has been determined to be related to the collagen source. UV cross-linking enhanced tendon collagen strength and exhibited no relationship to irradiation level, while dermal collagen strength strengthened at the lowest intensity and deteriorated at higher intensities. All this became balanced through the rival actions of collagen crosslinking and collagen breakdown encountered throughout UV exposure [138].
However, what type of in vitro cellular integrin-driven response is expected after UV cross-linking? Specifically, α2β1-integrin, which facilitates HT1080 cell and platelet binding to collagen, and HT1080 spreading and proliferation have been shown to be not impaired upon UV cross-linking, indicating that GXOGER sequences remained intact upon UV treatment [138]. On the basis of these findings, the synergistic crosstalk effects of UV crosslinking and EDC-NHS crosslinking on insoluble bovine Achilles tendon collagen type I have been examined. UV irradiation has been determined to restrain adhesion of α2-I domains beyond the anticipated impact of EDC-NHS on its own, with EDC-NHS concentration-dependent hindrance of HT1080 cell adhesion and cell coverage. It has been theorized that this is due to the engagement of phenylalanine (F) in UV crosslinking, leading to the depletion of GFOGER crosslinking motifs, which is the tightest tethering of the GXOGER motifs. Consequently, tethering is counterbalanced through the GLOGER motifs, wherein L stands for leucine, being compensatory for the attachment of the α1-I domain, but having a weak affiliation for α2-I domains [139].
2.1.3.1.5 Electron Irradiation Crosslinking
High-energy electron-induced crosslinking techniques have been revealed to be useful for accurately tuning collagen characteristics for extracellular matrix schemes [140]. Due to the procedure’s minimally invasive nature, collagen remnants stay intact when exposed to high-energy electrons (Figure 4). Specifically, a collagen network 3D pore size analysis as a matter of irradiation dosage indicates an enhancement in density resulting in a reduction in pore size. In addition, mechanical characterization of these scaffolds by rheological techniques reveals increased storage and loss moduli that correspond to an enhancement in crosslink density. Collagen gels can be modified to adapt specific features, such as structural or mechanical cues, to mimic natural and physiological extracellular matrices. These biomimetic scaffold systems need to exhibit a designed and engineered scaffold architecture, distinct mechanical characteristics, distinct thermal stability and display a specific swelling phenotype, precise and non-cytotoxic crosslinking techniques are demanded [141]. Since conventional techniques use compounds such as aldehydes [116,142], tris (2,3-Epoxy propyl) isocyanurate (TEPIC) [143], or enzyme-based cross-linkers [144], which can negatively impact cell performance, reagent-free techniques are of great advantage. Electron irradiation is very efficient among them to network polymeric hydrogels [145]. In this process, macro- and radical ∙OH radicals are generated due to homolytic scission of bonds on the polymer chain and radiolysis of water molecules [146]. The radical ∙OH radicals continue to target the polymer chains, generating additional macroradicals. The macroradicals are extremely reactive and self-recombine to establish covalent bonds that build cross-links inside the polymer matrix. By comparison with chemical crosslinking techniques, electron irradiation at 5–20 kGy holds the prospect of high effectiveness and accurate and rapid crosslinking without causing cytotoxicity of gelatin gels [147,148]. It also instantly sterilizes the media, thereby guaranteeing biomedical usage [149]. Inside the class of ionizing irradiation, electron irradiation is very beneficial for the hydrogel alteration owing to its high penetration depth [150] and high dosage rates [151], which facilitates homogeneous crosslinking. Moreover, it provides accurate global as well as on-site meshing through the utilization of a highly centered electron beam, thereby paving the way for a variety of uses spanning from mechanical texturing to actuators [147,152]. Rather, for future biomedical applications including extracellular matrix models, a characterization of electron beam crosslinked collagen gels with respect to network structure, such as pore size, rheological features, and cytocompatibility, has been performed [140]. Fourier-transform-infrared spectroscopic observations demonstrate that electron beam-assisted crosslinking causes only small alterations, whereas the distinctive polymeric architecture of the collagen is maintained across doses of 50–100 kGy. Electron irradiated collagen possesses high cytocompatibility and can be combined with other isolation techniques of tissue-based scaffolds that comprise a high content of collagen type I, such as decellularized matrices of tissue biopsies or specimen. Consequently, these collagen-rich tissue scaffold samples can be mechanically tailored. Decellularized scaffolds and 3D-printed scaffolds utilizing biomaterials can become nature-like tissue scaffolds.
2.1.3.2 Biological Cross-Linkers
2.1.3.2.1 Genipin
Genipin, a substance derived out of the fruits of Gardenia jasminoides Ellis, has proven to be an alternative biomaterial crosslinking agent in view of the cytotoxicity of crosslinking agents, including glutaraldehyde and formaldehyde [153]. Based on the firmly entrenched food safety of genipin, the crosslinking technique has been proposed for applications in collagen-, gelatin-, and chitosan-based scaffolds and drug-delivery schemes [153]. Genipin has been implicated as a cross-linker in chitosan-based frameworks and is assumed to obey the same two-step mechanistic route in both chitosan and collagen (Figure 4). One of the crosslinking steps has been mapped to incorporate a secondary amide bond of a free amine to the genipin through a nucleophilic SN2 substitution [154,155], and more recently it has been delineated that the second crosslink develops via two additional pathways to accomplish crosslinking to collagen, either through two imide crosslinks or two amide crosslinks [155]. Genipin crosslinks gelatin via lysine and arginine moieties and is anticipated to pursue a resembling mechanistic route in collagen [156]. The cross-links established with genipin provide collagen scaffolds that raise the elastic modulus by almost an order of magnitude. Collagen scaffolds derived from rat tail tendon type I with a porosity of 92% have been shown to be most efficacious when subjected to high crosslinking concentrations (0.7812 wt/wt%) and temperatures (20–37°C), yielding a compressive modulus of elasticity of 30 kPa relative to a noncrosslinked reference control at 5 kPa [157].
However, there seems to be cytotoxicity of genipin at very large concentrations above 5 mM, when crosslinking collagen type I matrices [156]. In addition, neurocompatibility and long-term large animal research trials have proven that genipin, when injected directly into the spine of individuals, relieves their chronic low back pain through enhancing the mechanical characteristics of the annulus [158].
Another examination of the mechanical characteristics of genipin involving bovine type I dermal insoluble collagen indicated that at the highest crosslinking concentrations (1.5624 wt% at room temperature), genipin can function as an alternative for the intermediate crosslinking constraints of EDC-NHS, enhancing both the Young’s modulus and the stress to rupture [159]. Integrin-specific binding has also been seen to be not impaired by genipin crosslinking, leading to high proliferation levels in human dermal fibroblasts and minimal cellular toxicity [159].
2.1.3.2.2 Riboflavin
Riboflavin, synonymously referred to as vitamin B2, has also found to be biocompatible in terms of achieving crosslinking of collagen matrices with blue light. Riboflavin crosslinking of collagen is of specific concern due to the short application times, such as 15 min, needed to obtain pronounced enhancements in mechanical characteristics, such as a 2.5-fold raise in modulus of elasticity [122].
Riboflavin has been used to generate crosslinks in a collagen matrix, such as type I insoluble, bovine Achilles tendon membranes, in specific, through arginine, histidine and lysine amino acids (Figure 4). Even though lack of arginines can lead to impediment of integrin-mediated adhesion, it has been postulated that arginines are not as functional for stabilization of a divalent cation in GFOGER motifs as are glutamines, that are being lost upon EDC-NHS crosslinking [122,160]. However, cell adhesion assays verified that only α2β1-integrin-mediated binding has been impacted, as assessed with HT1080 fibrosarcomas, while integrin binding has been left undisturbed in human dermal fibroblasts expressing a variety of integrins. However, in contrast to EDC-NHS-crosslinked films, riboflavin-crosslinked films failed to induce an enhancement of nonspecific binding while exhibiting similar ultimate tensile strengths compared to EDC-NHS-crosslinked collagen membranes [122,160]. In an investigation of plastically compacted collagen scaffolds, a decline in oxygen diffusivity and viability of human dermal neonatal fibroblasts has been seen after crosslinking of a compacted rat tail collagen type I scaffold [161]. Nevertheless, this cytotoxicity could result from an interplay of compression and crosslinking, as higher levels of plastic compression led to a more pronounced reduction in cell viability compared with riboflavin crosslinking.
2.1.3.2.3 Polyethylene Glycol
The impact of polyethylene glycol polymers with varies molecular weights (Figure 4), degree of branching, and specific terminal groups have been largely analyzed in terms of cross-linking and functionalization of 3D collagen matrices [162–166]. Branched cross-linkers increase the amount of fibers converging at every junction of the scaffold, which is referred to as local connectivity or junction points of the network and additionally enhances the mechanical strength of the hydrogel [167,168].
2.1.3.3 Cell-Derived Cross-Linkers
Cell-derived cross-linkers are undoubtedly the most important class of cross-linkers, and thus greater research efforts need to be devoted to them in the future, as they have the potential to be non-toxic to cells and may also affect the mechanophenotype of the extracellular matrix environment, which in turn may alter the cellular mechanophenotype. In specific, cell-based cross-linkers play a pivotal role in the characterization of organoid and therefore special attention must be paid to them.
2.1.3.3.1 Transglutaminase
Transglutaminases pertain to a family of transferase enzymes that network proteins through the establishment of a bond between an ε-amine (lysine) and γ-carboxyl in glutamines (Figure 5). Many types of transglutaminases exist, among them microbial transglutaminase, factor XII, epidermal, keratinocyte, and tissue transglutaminases, which are frequently encountered as cross-linking reagents in skin, hair, and blood clots in vivo. Within these enzymes, tissue transglutaminase 2 (TG2) is a calcium-dependent enzyme that has exhibited an exceptionally high cellular contribution, encompassing an enhancement in the number of osteoblast adhesions following TG2 crosslinking of freeze-dried type I calfskin collagen scaffolds [169].
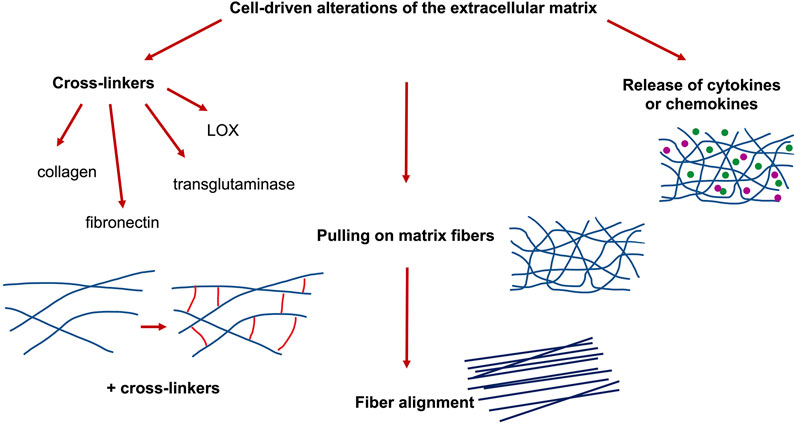
FIGURE 5. Cell-driven alterations of 3D extracellular matrix scaffolds, such as cross-linking of cell-derived molecules, cellular pulling on collagen fibers and cell-released cytokines or chemokines.
Transglutaminases attach to glutamines in the polypeptide chain and engage them for subsequent reactions [170]. In the vicinity of water, this leads to the conversion of the glutamine into a glutamate residue, while in the presence of a suitable amine, an amide bond is produced at the location of the activated glutamine [170]. Therefore, TG2 may serve either as an amide cross-linker that avoids preexisting aspartic or glutamic acids (E and D) in the generation of crosslinks or, conversely, may help to reintegrate glutamates (E) into the substrate, thereby enhancing the number of MIDAS motifs that can be accessed on the substrate for integrin sensing.
The analysis of mechanical properties of TG2 revealed global characteristics, such as the tensile modulus, plasticity and failure strength of TG2-treated films, that are increased compared to non-crosslinked type I bovine dermal insoluble collagen films [122]. TG2 treatment has been not observed to hamper the spreading, attachment, cytotoxicity and proliferation rate of human dermal fibroblasts to their substrate.
2.1.3.3.2 Lysyl Oxidase
Lysyl oxidase (LOX) represents an amine oxidase that is copper-dependent and fulfills a prominent function in the course of connective tissue matrix through crosslinking the extracellular matrix proteins, such as collagen and elastin (Figure 5). LOX promotes the catalysis of the oxidative deamination of specific lysyl and hydroxylysyl residues within collagens and elastin, which represents the initial step of the covalent crosslinking of these extracellular matrix components [171,172]. Therefore, it acts as a key regulator for collagen homeostasis. LOX concentrations rise in numerous fibrotic diseases, in contrast to the reduced expression of the enzyme in specific diseases with disturbed copper metabolism. LOX is produced as a preprotein that is liberated by secretion as a 50 kDa N-glycosylated proenzyme and subsequently undergo proteolytic cutting to the 32 kDa catalytically active mature enzyme. Effectors or conditions that regulate LOX expression comprise transforming growth factor (TGF)-β, platelet-derived growth factor (PDGF), angiotensin II, retinoic acid, fibroblast growth factor (FGF), altered serum conditions, and shear stress. As new LOX-like genes have been discovered, a multigene family may be in question. There is also growing awareness that LOX can have additional important biological roles in parallel to its function in cross-linking elastin and collagen in the extracellular matrix [118].
2.1.4 Collagen and/or Fibronectin Secretion (Altering the Composition of Fibers)
Apart from the cell-derived cross-linkers, the secretion of extracellular matrix proteins by cells and tissues can alter the structural and mechanical properties of the extracellular matrix environment. Currently, 29 types of collagen are known, grouped into types I, II, III, IV, and IX, and are more prevalent in humans [173–175]. In vivo, fibril-forming collagen promotes the creation of fibers, and the fibers are intertwined in a specific manner due to the specific tissue characteristics [176]. The self-assembly of collagen into fibers represents a gradual process [177,178], which is impacted through parameters including initial concentration, ionic strength, temperature and pH [179–181]. The presence of potassium ions causes the formation of the banding pattern, referred to as “D” period patter, of collagen fibers [182]. Moreover, collagen fibers fulfill a largely irreplaceable role in the proper functioning and structure of tissues. On the cellular scale, cells serve as one of the main components of the extracellular matrix, the achievement of multiple functions of cells is highly reliant on the availability of fibers, such as cell adhesion, motility, proliferation and metabolism [183–188]. On the organic scale, collagen fibers provide a fundamental basis for the shape of tissues [189–191], mechanical characteristics [192–194] and tissue repair mechanisms during the process of wound healing [195,196]. In addition, cell-secreted collagen, such as type I or type IV, or fibronectin molecules can crosslink the collagen matrix, both of which enhances the elastic modulus of the collagen matrices (Figure 5).
2.1.5 “Pulling” on Fibers
A scalable technique has been presented to determine how multicellular clusters rely on their capacity to locally pull, push, and even twist the neighboring extracellular matrix. Therefore, they probed their technique by applying biochemical treatments toward cell clusters in order to perturb cell–cell and cell–matrix interferences, which cause subsequently a remodeling of the overall cell cluster and consequently lead to a dissemination with specific mechanical signatures of matrix deformation [197]. These mechano-signatures can alter the mechanophenotype of the microenvironment and specific tractions, encompassing spatially heterogeneous contractile, protrusive, and circumferential types. Multicellular clusters in diverse phases of the epithelial–mesenchymal transition exhibit a successive decrease of protrusive and circumferential tractions, and the generation of localized contractile tractions due to elongated shapes of the cell cluster, all of which has been characterized. Consequently, the mechanical probing of collagen fibers can lead to aligned and hence oriented collagen fiber architectures (Figure 5). Thereby, oriented collagen fiber scaffolds foster the migration of cells [75,198], differentiation [199], wound repair mechanics [200], and the assembly of vascular framework [201]. These results point out to the usefulness of oriented collagen fiber matrices during tissue repair, regeneration, and other functions that deal with the repair and regeneration of bearing tissues with distinct fiber orientation, including discs, cartilage, ligaments, and fibers.
In specific detail, through placement of collagen type I matrices within prestrained (0, 10, 25, 50% strain), poly(dimethylsiloxane) (PDMS)-based microcavities and liberating the mold strain after matrix polymerization, collagen gels have been polymerized with diverse fiber alignment. Endothelial cells incorporated within the various matrices reacted to the elevated collagen fiber orientation with formation of 3D vascular reticulations consisting of thicker, directional branching that promoted collagen IV deposition and lumen creation compared with control conditions. These substrate-dependent variations in microvascular meshwork assembly have been linked to modified cell division and migration modes and have been linked to augmented mechanotransduction pathways [201]. These results suggest that collagen fiber alignment can provide a direct modulation of vascular reticular assembly and that culture systems containing aligned collagen can be employed to explore the underpinning mechanisms, thereby advancing finally the understanding of disease, tissue evolution, and homeostasis.
When subjected to the mechanical force, the buckling sites in the fibers and molecules are initially extended [202], and the buckling sites accumulate a portion of the energy produced due to the stress in the shape of elastic potential energy in a spring-like fashion. Afterwards, when the tensile force progressively grows, the fibers and the molecules slip with respect to one another, and the sliding among the fibers results in the energy continuing to dissipate. In the course of this, the slippage releases energy in a damper-like fashion and maintains a fairly slow rate of variation of the structure under stress [203,204]. The emergence of fiber rearrangement leads to the stress-strain characteristic of the tendon becoming nonlinear, which means that under lower stress, the fiber experiences a large degree of deformation [205–207]. The linear area in the mechanical curve is the action of elongating the fiber. Ultimately, the fiber is extended until it ruptures under loading.
2.1.6 Fiber Alignment Theory
Collagen fibers have been detected in the compartments of fibroblasts when monitoring chicken embryonic corneal cuts [208] and subsequently the same effect has been seen in chicken embryonic tendons [209]. Specifically, it seemed that microfibers are formed in the compartments and that the microfibers increase in density due to side-to-side fusion of the compartments into a dense bundle. Based on experimental findings in embryonic chicken and rat tail tendon, the theory of “fibripositor” has been formulated, that is, the fibers are sequestered into the extracellular matrix through the pores created by the merging of microtubule carriers for the microfiber trafficking and cell protrusion. The alignment of the fibers throughout this process is in accordance to the alignment of fibripositor [177]. The theory has been advanced by the idea that fibripositor are able to apply an inward pulling force on fibers and combine with tissue tension to reorganize the fibers [210]. A schematic model of the fibripositor has been provided in Figure 6. Mechanics has then been determined to have a major impact in the mechanism of aligned fiber generation via the development of a suggested model that accounts for the nature and mode of tendon growth during tensile loads [211]. In this regard, there is also an assumption that the generation of oriented fibers is related to the mechanism of collagen liquid crystal [212], which means that the collagen monomer in highly concentrated liquid solution self-composes into oriented fibers in some restricted environments. Nevertheless, the theory of liquid crystal orientation is not able to fully clarify the generation of mature fibers. Thus, the theory of fibripositor seems to be more suitable to provide an appropriate mechanism of fiber alignment, and the mechanical microenvironment may fulfill an additional task in the alignment process of the fibers.
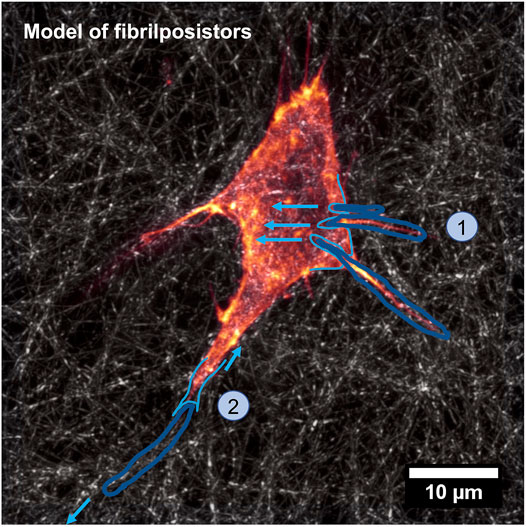
FIGURE 6. Model of the fibripositor. A single fiber is stretched inward due to the tension of the fibripositor (step 1), which is drawn out of the fibripositor because of the tension of the extracellular environment (step 2).
2.1.7 Release of Cytokines or Chemokines
Infiltration of immune cells into solid cancers, their locomotion within the tumor microenvironment, and engagement with other immune cells are governed by their directional movement in the upward direction of chemokine gradients. Deregulated chemokine signal transduction pathways in the tumor microenvironment foster growth of cancers, efflux of effector immune cells, and plethora of immunosuppressive cells. Within physiological settings, the movement of cells within the organ is decisive and governing for the outcomes of the immune system [213,214]. Thus, the inter- and intraorganic locomotion of immune cells is directed through a set of secreted molecules known as chemokines. Immune cells which express the cognate chemokine receptor translocate in response to gradients of the respective cognate ligands in a signaling mechanism referred to as chemotaxis [215].
To date, 50 chemokine ligands and 20 chemokine receptors have been characterized, and all but six chemokine receptors are sensitive to more than one chemokine. Chemokines are classified into four principal classes, according to the position of the first two cysteine (C) residues in their protein sequence: specifically, CC, CXC, C, and CX3C chemokines. The majority of chemokine receptors are cross-membrane heterotrimeric G protein-coupled receptors [214]. Chemokine receptor binding initiates G-protein linkage and consequent activation of subsequent signaling proteins implicated in cell migration, including Rac, Rho, and Cdc42. The overall impact is a motion of the cells in the same direction as the chemotactic slope [214].
Influx of immune cells into the cancer microenvironment remains a pivotal determinant of cancer prognosis, and chemokines serve an integral purpose in guiding the directional migration of both activating and suppressive immune cell types [216–219]. Immune cell movement into cancer tissues is less foreseeable than homeostatic migration of immune cells into lymphoid organs since solid cancers are ectopic and heterogeneous and have no well-defined anatomy. Among even same-type cancers, immune cell migratory profiles differ with time and individual cases. Nevertheless, insight into the chemotactic milieu of solid cancers and recognition of chemokines that govern immune cell entrance into solid cancers is vital for enhancing contemporary immunotherapeutic therapies, encompassing immune checkpoint blockade (Figure 7).
Landmarks that govern this migratory response include soluble signals such as chemotaxis [220] and tethered chemo-attractants/repellents, such as haptotaxis [221]. Moreover, the latter is linked to the durotaxis response of the cells. Chemokines comprise chemotactic cytokines that direct the migration and placement of immune cells within tissues and are crucial for the proper functionality of the innate immune system. Moreover, they play a role in cancer disease, such as malignant progression of cancer. Deregulated chemokine signal transduction in the cancer microenvironment favors growth of cancers, efflux of effector immune cells, and plethora of immunosuppressive cells. Key chemokines that govern immune cell migration into cancer tissues have been pinpointed.
Various investigations have emphasized how chemotactic agent cues, and in specific chemokines, can function as the natural antagonists or act to trigger synergistic actions on selective receptors through the generation of heterocomplexes, thus affecting the migratory immune cell replies. Different chemokines may also mutually interfere with one another and display antagonistic or synergistic behavior at targeted chemokine receptors. They can simultaneously elicit distinct receptors, leading to either arrest or amplification of intracellular cell signals [222,223], or a single receptor can be engaged through a heterocomplex of two chemokines, leading to a more robust cellular answer [223,224]. A number of chemokines have been characterized to generate heterocomplexes both in vitro and in vivo under both inflammatory and regenerative circumstances (G. [223,225,226]), but little is yet appreciated about the existence and pertinence of heterocomplexes in the cancer microenvironment [227]. In this regard, chemokines may also mutually interfere with inflammatory substances liberated in the microenvironment, thus enhancing cellular reactions triggered by chemokine receptors [228,229]. It has been delineated that the alarmin high-mobility group box protein 1 (HMGB1), capable of massive liberation in the microsurroundings of the cancers, creates a complex with the chemokine CXCL12 that augments CXCR4-driven signal transduction, thereby adding to the modulation of the activity of the chemokine network [230]. However, there is still an ongoing debate whether all in vitro heterocomplexes are relevant for in vivo situations.
3 Extracellular Matrix Acts on Cells and Cell Clusters
In the past, the majority of cell culture assays has been performed in a simple 2D environment, where the cells or collections of cells were cultured on a flat surface treated for tissue culture. For this reason, the focus of this review is slightly shifted to the cellular side acting on their microenvironment. However, in more advanced approaches, the surface is modulated by several coatings with commonly employed extracellular matrix proteins, such as fibronectin, collagen, vitronectin or laminin. The motility of cells has been assessed employing these flat surfaces on which mainly the cell adhesion to the substrate is the rate-limiting factor of cell migration and a gradient either of the coating or in the culture liquid can determine the directionality of the movement. Thereby, 2D and 3D environments as well as the in vitro and in vivo cell migration situations need to be taken into account, since these data may point in opposite directions [231].
The architecture of tissues is based on the extracellular matrix that serves as its major contributor. The extracellular matrix assembles a 3D scaffold and is mostly secreted by nearby stromal cells, which contains a broad range of fibrous and non-fibrous elements. Additionally, the extracellular matrix stores non-structural elements, such as polysaccharides [232]. Consequently, multiple in vitro cell culture scaffolds that can imitate the characteristics of the extracellular matrix have been developed to examine tumor biology, such as various 3D cell culture models. Various implementations of 3D culture scaffolds have arisen incorporating prominent hydrogels [233,234], such as collagen [235–237] and Matrigel [238], non-adhesive surfaces [239,240] and artificial frameworks [241–244].
In principle, 3D culture systems are expected to more closely match the physiological extracellular milieu in vivo than conventional 2D cell culture systems. While these cell culture tools assist in resolving some key concerns, they may be coupled with multidisciplinary intricacies. For instance, the non-uniform largeness of spheroids accounts for the low repeatability of cell responsiveness to cytotoxic pharmaceuticals [245,246]. In addition, large spheroids do not have a vasculature in the center, so the potential inability to supply drug to the entire multicellular spheroid is likely to be an influential contributor to reduced chemosensitivity rather than cellular resistance to pharmaceuticals [247]. Cell culture requirements can cause the expression of certain genes to shift, which in turn results in altered cellular behaviors [248,249]. Consequently, to a certain degree, in vitro cell reactions do not actually reproduce the response of cancer cells in situ, but show cell behavior linked to the prevailing experimental circumstances [250–253]. Based on this finding, it is important to cautiously conceive the selected cell culture model in order to explore the specified hypotheses.
3.1 Benefits of 3D Extracellular Matrix Environments Over 2D Substrates
Since drug screenings have been less efficient, there is a need for new oncology drug candidates to be successfully identified for testing in clinical trials. The bad outcome of the predictive capacity of artificial monolayers based on 2D culture assays has contributed to a large amount to this urgent demand. Monolayer assays fail to consider the natural 3D microenvironment of the cells. Consequently, false positives frequently get into clinical investigations, resulting in high attrition rates and a huge amount of wasted time and resources. During the last two decades, a wide range of 3D in vitro cultivation instruments have been created by both tissue engineers and cell biologists to improve the depiction of cell biology in vivo. These instruments maintain the 3D architecture of the cells and can be leveraged to forecast the toxicity of and resistance to antitumor compounds. Advances in tissue engineering further enhance 3D cell models by incorporating the tumor microenvironment, which is essential for metastatic spread progression and the formation of blood vessels. Nevertheless, the pervasive adoption of 3D cell culture in cell-based drug discovery applications has been constrained by multiple factors, among which are their expense and replicability. Moreover, different 3D cell culture methods often yield spheroids of varying size and shape, which can greatly impact drug potency and toxicity. Therefore, it is essential to morphometrically classify multicellular spheroids to circumvent confounding assumptions between distinct spheroid types. Standardized 3D culturing techniques may decrease data variation even further and increase biological pertinence.
3.2 Structural Characteristics of the Matrix Environment: Composition, Such as Structural Elements and Storage of Non-structural Molecules
The components of the extracellular matrix combine to create a structurally robust compound and provide a key input to the mechanical characteristics of the tissue. The extracellular matrix is also a repository of growth factors and bioactive molecules. It is a very dynamic unit that is of crucial importance as it defines and monitors the most basic functions and properties of cells, including proliferation, adhesion, migration, invasion, polarity, differentiation, and apoptosis. The extracellular matrix environment can influence the migratory behavior of cells therein by altering the matrix composition and the storage of non-structural elements, such as growth factors, cytokines, chemokines, enzymes or matrix cross-linkers.
The extracellular matrix environment can be termed “core matrisome” [254] and includes more than 300 proteins. Among them are collagens, elastin, fibronectin, proteoglycans, and cell-binding glycoproteins, each of which possesses specific physical and biochemical characteristics. The two major components are presented briefly in the following.
Collagen is made of three polypeptide α chains that build a triple helical structure. In vertebrates, 46 different collagen chains combine to generate 28 collagen types [174,255] that can be divided into fibril-forming collagens, such as types I, II, III, fibril-associated collagens with interruptions in their triple helices, or FACITs, such as types IX, XII, network-forming collagens, such as the basement membrane collagen type IV and others, including type VI. The fibril-associated collagens comprise uninterrupted triple-helix-forming domains accompanied by amino- and carboxyl-terminal non-collagenous domains. These non-collagenous domains undergo proteolytic excision, and the triple helices produced are laterally attached to produce fibrils. Non-fibrillar supramolecular structures, exemplified by the reticular nets of collagen IV in basement membranes and pearly filaments, are constituted by non-fibrillar collagens. FACITs are not self-assembling into fibrils, but are instead accompanied by collagen fibrils.
Proteoglycans are composed of a core protein with side chains of glycosaminoglycans appended to it. Glycosaminoglycans are linear, anionic polysaccharides consisting of repetitive disaccharide entities. Four groups of glycosaminoglycans exist, such as hyaluronic acid, keratan sulfate, chondroitin/dermatan sulfate, and heparan sulfate with the latter covering heparin. All are sulfated apart from hyaluronic acid. The highly negatively charged GAG chains enable proteoglycans to bind water and divalent cations, giving them room-filling and smearing properties. Secreted proteoglycans comprise large proteoglycans including aggrecan and versican, small leucine-rich proteoglycans such as decorin and lumican, and basement membrane proteoglycans like perlecan. Syndecans have a cell surface connection, whereas serglycine represents an intracellular proteoglycan.
Certain proline residues present in collagens are hydroxylated through prolyl 4-hydroxylase and prolyl 3-hydroxylase. LOX additionally hydroxylates certain selected lysine residues. After processing, the fibrillar procollagens are sequestered into the extracellular compartment, where their propeptides are excised. The evolved collagens subsequently combine to form fibrils through covalent cross-links established between the lysine residues of two collagen chains through a process catalyzed by LOX. The basic collagenous framework determines the architecture, shape, and organizational structure of tissues. Proteoglycans also are known to cross-talk with growth factors and growth factor receptors and are involved in cell signaling [256] and biological functions, including angiogenesis.
The aforementioned components of extracellular matrix networks are just major constituents that pronouncedly impact the mechanical characteristics and non-structural components of the matrix that both serve as signaling reservoir for cells and tissues. Considerable evidence points to the extracellular matrix as an essential niche for stem cells, as ordinary stem cells are reliant on signal transduction across extracellular matrix receptors including the laminin receptor, α6β1 integrin [257], the vitronectin receptor αVβ3 [258], and collagen receptors, such as α1β1, α2β1, α10β1 and α11β1 integrins [259] and new indications that the cancer-associated extracellular matrix is an integral feature of the cancer stem cell niche [260]. Thereby, apart from the composition of the extracellular matrix environment, the architecture and the mechanical cues play a crucial role in creating an environment that supports a niche for cancer stem cells. In specific detail, the structural parameters, such as pore-size, fiber-size, matrix-alignment, fiber length and fiber nodes affect the mechanical characteristics of the matrix scaffold, such as stiffness, viscosity and poroelasticity. Consequently, there are increased efforts to precisely characterize the structural elements of extracellular matrix environments [167,261–263].
3.3 Mechanical Constraints of the Environment
Most of the investigations on the reaction of cells to mechanical pacing, nevertheless, are performed on planar 2D substrates, which are unable to resemble the natural mechanical microsurroundings of 3D cells. There is growing body of evidence that cell performance in 2D and 3D microenvironments is markedly divergent. Within the group of materials employed for engineering the mechanical microenvironment of 3D cells, hydrogels have become progressively more relevant due to their tunable characteristics such as chemical and mechanical attributes.
The relevance of the mechanical constraint in cancer has in fact been emphasized through experiments that change the composition and rigidity of the extracellular matrix [264]. Tumor growth has also been demonstrated to be regulated through mechanical compression induced through the tumor on its own as it grows in a constrained space [265,266]. Pressure-induced patho-physiological outgrowth of this type has also been examined in vitro. The growth of multicellular aggregates is significantly diminished when they are entrapped within soft gels [267–269] or subjected to mild osmotic compression [270,271]. Evidence has been obtained that the cell cytoskeleton is engaged in the reaction to compression and can induce growth inhibition through cell cycle stoppage [269,272]. Moreover, it has recently been suggested that cell volume is a pivotal factor in the mechanosensitive response tract [272]. However, it is not clear how such modest global compression is imparted to the single cells of the assemblage to modify their proliferation.
In this case, the cells are assumed to respond primarily to the mechanical stress transmitted through the extracellular matrix when the aggregate is under pressure [273]. The hypothesis is based on two experimental findings. Firstly, an aggregate is a compound substance comprising cells, extracellular matrix, and interstitial liquid. The existence of hydrated extracellular matrix is attested through the richness of fibronectin within the interstitial cavity (Figure 8). Because the extracellular matrix is 100–1000 times capable of compaction relative to the cells, it accommodates the majority of the deformation, while still imparting the mechanical stress to the cells. Secondly, while an osmotic pressure of a few kPa severely diminishes cell proliferation inside multicellular assemblies, an equivalent pressure has no apparent impact on individual cells cultured on a Petri dish, in the complete absence of extracellular matrix [271]. Moreover, the utilization of pharmaceuticals that influence the architecture of the cytoskeleton exerts a marginal impact on the actual compressibility of multicellular assemblies. This characteristic pattern suggests that the volume loss under compression is primarily attributable to the dehydration of the extracellular matrix [273].
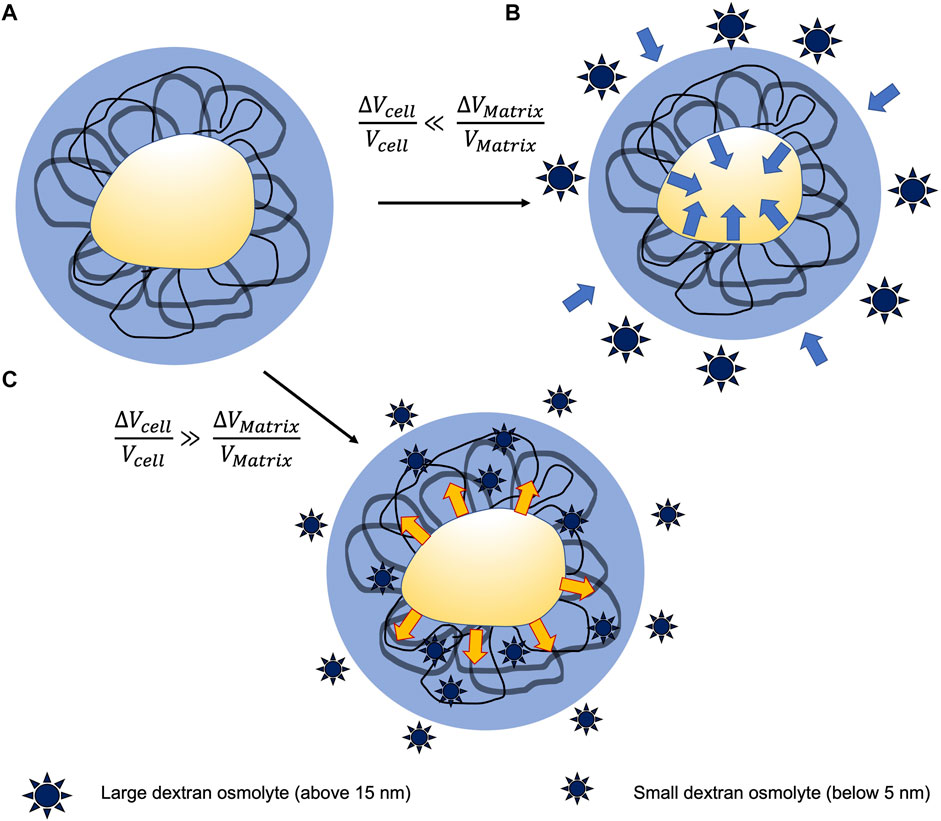
FIGURE 8. Interplay between cell aggregates and their matrix surrounding modeled through a selective compression technique. (A) Schematic drawing of a cell surrounded by the extracellular matrix filaments and permeated through the interstitial liquid. (B) Large osmolytes cannot penetrate the extracellular matrix scaffold and thereby a bulk compression is imposed toward the cell. Since the extracellular matrix is more compressible compared to the incorporated cells, the extracellular matrix effectively soaks up the majority of the deformation and imposes a positive stress on the cell. (C) Small osmolytes can penetrate the extracellular matrix scaffold in the absence of any osmotic pressure. However, they compress the cell that, in turn, transmits tension toward the extracellular matrix scaffold.
The significance of the mechanical environment in cancer has long been emphasized through experiments that change the constitution and rigidity of the extracellular matrix [264]. Tumor growth has also been found to be regulated through mechanical compression imposed directly through the tumor by its own extension in a constrained setting [265,266]. Pressure-induced patho-physiological growth of this kind has also been examined in vitro. However, when multicellular assemblies are entrapped through soft gels [267–269] or undergo mild osmotic compression [271,273,274], their rate of growth is markedly diminished. The presence of the intracellular cytoskeleton has been implicated in the reaction to compression and has been demonstrated to elicit growth arrest due to cell cycle blockage [269,272]. Moreover, it has also been proposed lately that cell volume is a pivotal factor in the mechanosensory response [272,275]. However, it is not clear how such gentle overall compression is imparted to the individual cells of the assembly to modify their proliferation.
3.4 Extracellular Matrix Fiber Reassembly Encourages the Mechanosensing of Distant Cells
Cells perceive the intrinsic mechanical features of the extracellular matrix by exerting tensile forces. The capacity of cells to react to external forces, to sense and translate the mechanical nature of the extracellular matrix, and to both synthesize and restructure it, has an essential part to fill in multiple domains of cellular performance [276]. For instance, extracellular matrix stiffening in the course of advancing disease states, including cancer and fibrosis [277], signal reservoir of non-structural elements [278] or the ongoing cell and tissue aging [279] may have a detrimental effect on cell migration, growth, differentiation and proliferation. In the alternative, abnormalities in intracellular signaling pathways that impair the capacity of cells to recognize and react to extracellular mechanical cues may also account for diseases such as cancer [277]. The capacity of cells to perceive and react to mechanical cues is referred to as mechanotransduction. Mechanotransduction involves sensing of external forces or biomechanical features and relaying this kind of input, which initiates a specified intracellular signaling answer. The cytoskeleton serves a vital purpose in mechanotransduction by connecting cellular compartments such as other cytoskeletal components and the nucleus to the force-sensing machinery [280].
The intricacy of the mechanical characteristics of the extracellular is placed in the focus and it is pointed out that cells sensing the mechanical characteristics of the matrix can in turn be impacted by them. While cells harbor multiple force sensors, including as the force sensitive channels TRPV4 and PIEZO1-2 [281,282] and intercellular adhesions [283], the focus of the current effort has been on mechanotransduction events at integrin-associated complexes. These link the extracellular matrix directly to the actin cytoskeleton and are key contributors to mechanotransduction. Thus, an important research focus is to investigate and discuss the functional role of protein dynamics of integrin-associated complexes in the context of their function in mechanosensing and signaling.
Elasticity has been seen to govern multiple cellular functions, such as the motility of various cells, cell growth, proliferation and differentiation [284,285]. Nevertheless, it is not merely the elastic characteristics that matter in stimulating modulation of cell performance, and in contrast to purely elastic polyacrylamide gels, tissues exhibit stress relaxation properties [286]. In fact, the proliferation of fibroblasts on soft surfaces is improved when the capacity to remodel the extracellular matrix is augmented by imposing stress relaxation in the subjacent surface or raising its viscous characteristics [287]. Moreover, hydrogels with greater or lower stress relaxation can control stem cell fate regardless of other known variables such as elasticity or ligand density [286]. The pairing of elasticity and viscous characteristics, or viscoelasticity, may be especially relevant to “in vivo-like” settings such as collagen nets, which exhibit significant viscous characteristics on time scales pertinent to cells [288].
It gets more complex when looking at the mechanical characteristics of extracellular matrix structures, because they offer more than just a stiffness coefficient: they have a tendency to rigidify when subjected to external forces [289]. This phenomenon is referred to as strain-stiffening of extracellular matrix networks that can also occur when cellular forces are applied, causing the entire network to become stiffer [4,290]. Stiffening by stretch can induce cells to gauge a stiffer ambient than would be expected due to initial polymerization constraints in vitro, and can establish a positive feedback circuit for achieving cellular force production [291]. Therefore, the final stiffness of the surrounding environment can be characterized as the aggregate of multiple determinants including the mechanics of the extracellular matrix meshwork, the cell density, and the capacity of these cells to react and apply tensile forces. In fact, cells that generate low traction, such as neuronal cells, rigidify the extracellular matrix meshwork through this stiffening mechanism considerably inferior to cells that produce high traction force potential, such as fibroblasts.
A major difference to 2D traction force analysis assays, such as those based on conventional polyacrylamide or PDMS substrates, and 3D traction force assays is that forces propagate further in the extracellular matrix scaffold. This characteristic feature of extracellular matrixes is highly similar to the in vivo situation, where the fibrous nature of the connective tissue microenvironment is present [292,293]. Subsequently, the distances over which cells can interact in a mechanical sense with each other are allowed to lengthen [294,295].
3.5 Classical Cancer Hallmarks Heavily Omit the Role of the Extracellular Matrix in Cell Migration
Since the first classic cancer features have been proposed over two decades ago [296] and more of them have been presented more than a decade ago [297], there is a new discussion about whether these features are still appropriate or need to be redefined. More challenging arguments have questioned not only the incorporation of specific characteristics, but also the question: what exactly is it that truly defines a cancer characteristic feature? The term cancer is commonly referred as a malignant tumor [298]. In this sense, a “hallmark” is a distinctive characteristic. However, it needs to be mentioned that five of the six initial hallmarks, but not the hallmark of invasion and metastasis are present in both benign and malignant neoplasms. Therefore it is difficult to distinguish benign from malignant cancers. It may be necessary to supplement that even invasion and spreading are characteristics of certain non-malignant diseases. An instance of this is endometriosis, a relatively frequent disease in women in which endometrial cells migrate to extraanatomical locations and infiltrate new tissue while retaining a benign histologic phenotype [299].
However, it can be argued that the purpose of hallmarks is to establish an organizational setting for cellular features that are exposed throughout the transformation (phenotypically) of normal cells - regardless of whether such transformation ends in a benign growth stage or further develops toward a more advanced and menacing malignancy. Thus, an evolutionary approach to mutation theory can be selected, wherein carcinogenesis represents a dynamic process that can begin (and end) within the lifespan of cells, with cancer characteristics becoming evident during this trajectory [300].
Criticism has also been leveled at the reductionist assumption of somatic mutation theory-the so-called bottom-up perspective on the examination of carcinogenesis that views cancer simply as a disease in which “genes are out-of control,” whereas more organismic, systems- or tissue-disorganizing perspectives have been proposed [301–303]. It is evident that even the original hallmarks list includes tissue-relevant rather than cancer cell-specific moieties, such as angiogenesis [296] and the hallmarks II manuscript contains a part addressing the importance of the tumor microenvironment [297]. Most recently, phenotypically normal cells have been demonstrated to have a high load of nonsilent somatic mutations that are positively targeted in the absence of cancer [304–306]. Consequently, the usefulness of hallmarks of cancer is under question. Additionally, the stromal element of cancers has been identified as an incidental catalyst [307,308] and imperative accessory [305] of the carcinogenic progression.
While the concept of hallmarks brings similarities, it is nevertheless critical to remember that cancer is not a uniform disease and that reliance on common pathways-and the implications of their use-vary across cancer types. For instance, vascularization is an essential hallmark of cancer, but several cancers are poorly vascularized and may rely somewhat less on one such hallmark [309]. Another instance is the tissue-specific usage of branched-chain amino acids in cancer cells that exhibit the identical driving mutation [310]. Consequently, cancer can be considered to be a multitude of diseases, each with a distinct agenda, utilizing features to varying degrees, and consequently demanding customized therapy.
4 Cell Mechanophenotype
The migration of cells is fundamental to basic stages of development and adulthood, encompassing embryogenesis, wound healing, and inflammatory reactions [311]. The migratory capacity results broadly from the active momentum of its intracellular constituents-primarily the cytoskeleton-which produces propulsive forces and defines the anterior-posterior polarity of cells [1,312]. The spatiotemporal dynamics of the cytoskeleton are governed through intricate regulatory reticulations [313], and can be typified through both deterministic and stochastic moieties [314–316]. The temporal incorporation of these intricate intracellular dynamics dictates the large-scale characteristics of cell trajectories, which for their part can be employed as accessible metrics to deduce intracellular characteristics [317–319], as well as cell interactions with the surrounding area [320–322] or with adjacent cells [323,324]. Cells interoperate in vivo with various extracellular settings with a broad range of biochemical and biomechanical characteristics [1]. These exchanges have been demonstrated to be bidirectional: ambient stimuli directly impact cell form, migration, and polarity [325–327], and reciprocally, cells participate in active ways to reshape their surroundings [328,329]. However, to date, both pathways have been characterized separately, and the feedback of cell-induced reshaping of the environment on the large-scale attributes of cell migration has largely eluded investigation [330].
To surmount the intrinsic challenges of assaying cell migration in 3D conditions in vivo, the engineering of micropatterned surfaces has emerged as a high-performance strategy [331–333]. Within these types of in vitro settings, and especially in 1D settings, the decreased dimensionality of the cellular surroundings permits a comprehensive quantitative analysis of the phase space traversed by the traveling cells. Notably, while such 1D assays have unveiled noticeable deterministic characteristics in cell movement profiles, cell pathways in higher dimensions appear to remain arbitrary [315,323,328,334,335]. In addition, a lot of the characteristics of cell migration on a 1D substrate can imitate cell performance in a 3D matrix [328].
4.1 Single Cell Mechanophenotype
Components of the cytoskeleton are readily identified as epithelial-mesenchymal transition (EMT) biomarkers, especially intermediate filaments such as keratin (within epithelial cells) and vimentin (within mesenchymal cells) [336]. Hence, it seems to be likely that these dramatic cytoskeletal scaffold alterations affect the overall cell mechanophenotype of individual cells. It is still a question whether these structural alterations occur first or whether the mechanophenotype of the cells emerges first and secondarily cause the well-known cytoskeletal challenges of cells undergoing a EMT or MET. Intermediary phases may also occur and render this phenomenon even more intricate.
To bridge the gaps in cancer screening and surveillance, one exciting line of research is cellular mechanophenotyping. To persist and prosper, cancer cells face many challenges related to extrinsic forces from adjacent cells, the extracellular matrix, and the vasculature. The process of identifying and gaining an intimate knowledge of their mechanical response to these forces ultimately appears to be advancing the knowledge of cancer. Additionally, mechanophenotyping, alongside the conventional approaches of immunostaining and genetic mapping, has the potential to deliver a complete portrait of a heterogeneous cancer. In future applications and experiments, the focus needs to be on the advancement of techniques and the development of new technologies that provide single-cell mechanophenotyping. Single cell analysis is critical because mechanical cues from the surroundings can mask the intrinsic mechanical characteristics of a cell, which can vary in the course of time. Phenotypic heterogeneity and plasticity are both defining characteristics of cancer cells and continue to be a difficulty when examining bulk end point assessments [337]. EMT seems to take place as a rare event within a small subfraction of cells, which can be overseen when solely conducting bulk measurement. Instead, comprehensive single cell measurements need to be performed. New knowledge of molecular and cellular length scale dynamics can be gained through live cell imaging on the basis of high spatial and temporal resolution in the course of invasion and EMT [338]. In specific, cytoskeletal protrusions are especially essential for directional migration, and cells can noticeably reshape their ambient extracellular matrix [339]. Conversely, cells can experience considerable deformation to pass through the extracellular matrix, a process that can be eased through a pliable (more compliant) cytoskeleton. Moreover, it has been postulated that cancer cells are considerably softer than their non-transformed equivalents, especially in the setting of stem-like states expressing vimentin [340].
This leads to the hypothesis about the universality of both the usage of migratory phenotypes by cells and mechanophenotypes of cells. It seems to be a rather simple view on the migratory phenotype of cells, when hypothesizing that all diverse cell types behave universal and hence exhibit the identical migratory phenotype with a specific and universal mechanophenotype. There are so many differences between the cell types or among the diverse differential stages of cells under physiological conditions that it seems to be not conclusive to state that they all acquire the exact same mechanophenotype when migrating through a dense extracellular matrix environment. Instead, it seems to be more accurate or intuitive to hypothesize that cells can adapt several diverse mechanophenotypes in order to migrate through their extracellular matrix tissue environment [2,341].
However, there is still debate on whether the aggressive cancer cells all employ the same mechanophenotype or whether they can adapt even a more elastic (stiffer cytoskeleton) to migrate and invade dense extracellular matrix confinements [236,341].
Finally, there is increased focus on the subcellular resolution of cell-matrix adhesions [342], and on collective responses conveyed through cell-cell connections [343]. Consequently, bulk studies conceal heterogeneity in the mechanistic characteristics of individual cells, particularly those infrequent subsets that aggressively result in cancer progression or resistance to treatment. The technologies on which the focus should be placed on encompass atomic force microscopy, hydrodynamic and optical stretching, suspended microchannel resonators, and mechano-node pore sensing [344].
Traditionally, EMT has been conceived as a multilayered regimen of phenotypic alterations that result in an epithelial cell gaining mesenchymal characteristics, incorporating changed polarity and cytoskeletal architecture [345]. EMT programs can be triggered through inflammatory cues, which include growth factors such as TGF-β, HGF, EGF, and WNT, hypoxia, and extracellular matrix components such as collagen I, which function via developmental transcription factors such as SNAIL, SLUG, TWIST, and ZEB1/2, as well as E2A proteins, E12/E47, to attenuate E-cadherin expression and trigger mesenchymal gene expression (Figure 9) [346]. Small, non-coding single-stranded RNAs (microRNAs or miRNAs) function collaboratively with transcription factors to modulate the facilitation or attenuation of EMT signaling pathway profiles in a sensitive, contextual fashion [346]. For example, the well-known miR-34 and miR-200 miRNA families serve dual functions as both tumor and EMT suppressors through establishment of double-negative feedback circuits with SNAIL and ZEB1/2, respectively [347]. EMT is capable of being excited to different degrees, resulting in a multifaceted array of intermediate stages referred to as partial EMT ([341,348,349,350,351]), and can be reverse-engaged through mesenchymal-epithelial transitions (MET). Collectively, these dynamic events have been more widely characterized as so-called epithelial-mesenchymal plasticity [352].
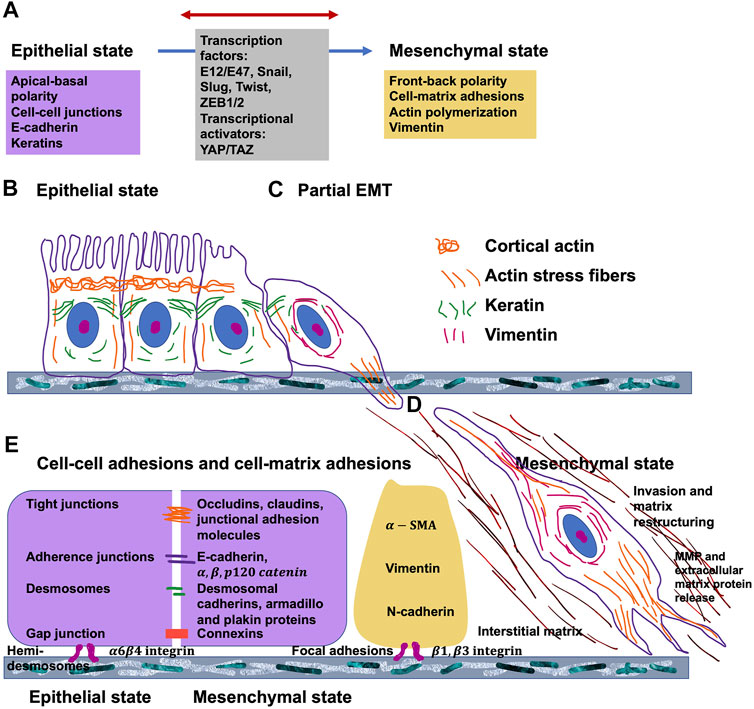
FIGURE 9. Epithelial-to-mesenchymal (EMT) progression. (A) General characteristics of epithelial and mesenchymal phenotypes and transcription factors that govern EMT. Cell shape and cytoskeletal structure of (B) epithelial stage, (C) partial EMT stage and (D) mesenchymal stage. (E) Cell-cell and cell matrix adhesions.
4.2 Bulk Cell Mechanophenotype
Insight into the contribution of the mechanophenotype to the competitive attachment of cells to other cells or to subjacent carriers may provide insight into mechanisms such as tissue evolution, progression of cancer, and injury healing. The purpose of this investigation is to determine how the mechanophenotype, characterized by entire-cell elastic/viscoelastic characteristics for the perinuclear area, and the cellular organization are entangled through the mechanosensing mechanism [353].
The whole cell mechanophenotype impacts a number of crucial features, manifold of which encompass proliferation, differentiation, motility, shaping, and multicellular connectivity [354]. Thus, gaining an appreciation of how the mechanophenotype affects cell performance in terms of the local, mechanical microsurroundings, is crucial to governing and guiding an outcome of achieving successful cellular architecture in regenerating tissues. The capacity of cells to accumulate or expand singly relies not alone on cell type, extracellular matrix ligands, and substrate rigidity, yet more notably on the intrinsic mechanophenotype [63,355].
The cells retained a signature perinuclear, entire-cell mechanophenotype on all supports, irrespective of their stiffness and throughout all cluster forms and assemblies generated by the cells. Non-transfected WI-38 cells achieved a steady mechanical condition 1 day after out-plating and retained this mechanophenotype for the remaining 3 days of the experiment. Mechanically diverse GFP-, dnRhoA-, and β-actin-transfected cells also exhibited intrinsic entire-cell mechanical characteristics that largely seemed to be disconnected to the external microenvironment. Specifically, in the presence of a polyacrylamide (PAA) substrate, the findings of this investigation further imply that cells have the capacity to mechanosensitively grasp their surroundings and electively attach and propagate to any material, PAA substrate or adjacent cells, that is more rigid than their intrinsic mechanophenotype (atomic force microscopy with 5 µm bead). The most compliant cell type displayed the lowest quantity of nodulation across all three gels, indicating that it tends to prefer to attach to the more rigid substrate rather than soft, adjacent cells. Moreover, meaningfully larger nodule development for all cell types has been noted on 0.3 and 0.5 kPa PAA gels compared to 1.4 kPa gels, where cells could expand and evolve a more patterned actin cytoskeleton [353].
The intrinsic mechanophenotype of WI-38 cells differed throughout the initial adhesion to a substrate, although it maintained a broadly stable pattern over time. The various cellular assemblies in place also started to build during the first day, suggesting that the cells are able to respond rapidly to their surrounding microenvironment. Because the mechanical properties of WI-38 cells do not change with time, single time point comparisons between the transfected cell lines have been made. Mechanically diverse transfected WI-38 cell lines also retained their intrinsic entire-cell mechanophenotypes on carriers of different rigidity. These findings are corroborated by other investigations that have also noted a stable, intrinsic mechanophenotype regardless of alterations in the cellular microsurroundings. Embryonic stem cells did not enhance their apical cell rigidity on surfaces of varying rigidity, whereas basal tensile forces at the border of cell-substrate interactions on PAA gels are in the spectrum of 0.35–8 kPa [356].
Oligodendrocyte progenitor cells have been revealed to be more compliant compared to differentiated oligodendrocytes. Moreover, the stiffness of both progenitor and differentiated cells appeared to be unaffected by the elasticity of the PAA gel and varied between 0.1 and 70 kPa [357]. It is relevant to remark that alterations in cell survival, proliferation, migration and other biological determinants emerged which remained autonomous from the cellular mechanophenotype, underlining that this indicator is not merely a secondary marker of normal cellular function [357]. However, other investigations have proposed that the mechanophenotype is a pliable property that adapts to the elasticity of the surface to which the cell is adhered [356–360]. Nevertheless, these experimental designs vary markedly in the mechanical assay strategies employed, such as atomic force microscopy with sharp pyramidal apices over the cytoplasm/cytoskeleton instead of spherical beads over the perinuclear region, the range of PAA gel stiffness examined, encompassing 0.5–40 kPa, which are several times higher than the WI-38 mechanophenotype, and the cell type examined, such as fibroblasts vs glioma cells vs endothelial cells. The focus is placed on whole-cell characteristics to yield an average measurement of a cell’s mechanical behavior, rather than nanometer-scale point measurements, which can differ widely according to what undergirding cellular constituent it is contacted. Gel elasticity has also been narrowly constrained to stiffnesses immediately above and below those of the cells, instead of involving elasticities orders of magnitude higher. In this way, the focus is on the competitive attachment of cells to a soft interface due to their elasticity [353].
4.3 Bulk Cell Spheroid Phenotype
Macroscale tools like tissue surface tensiometry and micropipette aspiration technique are not be employed to reveal local variation of around cells within the center of the spheroid. Instead, they can only provide results from bulk measurement from the outside of the spheroid. Cavitation rheology seems to be a suitable biophysical technique to determine the elastic modulus of spheroids from the inside, since the technique relates the knowledge of bubble formation to the spheroid deformation [361,362]. In particular, a spheroid is soaked partly into a glass capillary, whereafter a micron-sized glass needle, which is accurately controlled by a micromanipulator, is placed within the spheroid. Injection of a cavitation agent (air or water) creates an elastic instability in the shape of a void by applying pressure slowly. The pressure-growth correlation for this forced spherical bubble is based on the spheroid’s modulus of elasticity [207,363]. Specifically, matching the energy involved in bubble generation with the bond energies of the cell surface proteins yields an approximation of the cortical tension of the cells comprising the spheroid [361]. Spheroids are retained in the culture medium throughout the measurements, which presents a technical difficulty as they can swim away from the needle upon insertion. Besides, the elastic modulus values obtained are of validity under the “thick-shell” regime alone, where the forced voids are sufficiently small so as not to interfere with the outer diameter of the spheroid.
The local tissue mechanical characteristics can be assessed through the usage of hydrogels that serve as mechanosensors [270,364]. In specific, mechanically precisely determined elastic polyacrylamide (PAA) microbeads act as internal cell-like sensors, which have been embedded inside the spheroids that are cultured under mechanical stress [364]. These beads are fluorescently labeled for tracking properties and functionalized for serving as an adhesive substrate for the cells that exhibits total elastic characteristics. An advantage of the PAA material is that it is inert and the cell can solely interact through the linkage of the ligand coated to the beads and the cell surface receptor. The local pressure within the spheroid can be determined by detecting the strain of the hydrogels, which is reflected in volume alterations. The bulk modulus of the bead can be revealed by osmotic compression before the experiment start employing higher weight dextran solution. At relatively small compressions, which are observed at low dextran concentrations, the stress/strain relationship is purely linear and the bulk modulus of the beads can be derived from the inclination of the curve. Aside the linear regime, the empirical polynomial Mooney-Rivlin model can be employed. Thereafter, the pressure pattern of the spheroid can be analyzed before and after the osmotic compression of the entire spheroid with randomly distributed microbeads. These PAA gels can be fine-tuned in mechanics through alteration of the ratio between acrylamide and bisacrylamide [365]. To overcome a foreign body reaction, the microbeads can be coated with elevated levels of extracellular matrix proteins [366]. An alternative technique are hydrogels that can be tuned by temperature, such as PNiPAAM beads. These beads stay in a compact state under tissue culture temperatures, but expand when they are chilled down a couple of degrees. They can be envisioned as springs that are prestressed through thermodynamic driving out of water prior to their installation in the tissue. Reducing the temperature relaxes this preload and places the beads back into a new steady-state volume determined on the basis of the stiffness of the ambient tissue. The volume variation pertains to the elasticity of the tissue after the creep process. These samples can be injected in in vivo mouse cancers without causing additional fibrosis or inflammation over a timespan of 3 weeks, which indicates that they possess a suitable biocompatibility [270]. The calibration of the PNiPAAM beads can be performed by the encapsulation in PAA gels with fine-tunable stiffness that display linear elastic characteristics. In specific, through the alteration of the radii of the beads after releasing the pre-strain, the mechanical characteristics can be obtained. Nevertheless, it needs to be pointed out that these hydrogel mechanosensors exhibit at least four specific limitations. Firstly, the analysis of the tissue bulk modulus or elasticity following creep miss a time-dependent part. Secondly, PNiPAAM can be dependent on local surrounding parameters, including pH, which may be not homogeneous within spheroids [367]. Thirdly, the change in temperature required to carry out the analysis may impact the stiffness of the tissue, however, it has been demonstrated that cellular stiffness is not severely altered in the range of 21°C and 37°C [368]. Fourthly, hydrogel sensors can have an impact on cellular functions, as they represent foreign objects and trigger a foreign body reaction [366,369]. However, the functionalization of the hydrogel surface with suitable extracellular matrix molecules can aid to circumvent this effect. A major advantage of these beads is that they can be utilized directly for in situ mechanical probing within spheroids and living tissues.
Another alternative biophysical technique to investigate spheroid mechanics are optical tweezers. They permit the analysis of mechanics at a subcellular length scale inside spheroids that have been embedded within an extracellular matrix scaffold. Optical tweezers have been broadly employed, such as in physics, in soft matter [370] and in biology, including biological physics [371]. Moreover, the optical tweezer technique has been coupled to nano- and microfluids. The technique utilizes a highly strongly focused laser beam to optically trap a refractive object (bead) in the focal point [372], which offers excellent resolution in positioning (±1 nm) of micron-sized beads and in non-contact analysis of forces (±50 fN) [373]. Optical tweezers have recently been employed to carry out active microrheology on migrating cells in the circumference of a spheroid enveloped in a collagen matrix [275]. The mechanical characteristics of the cytoplasm within the peripheral cells have been gauged through the incorporation of tiny latex beads into the gel, which have been subjected to endocytosis. The force-displacement curve obtained when pulling the beads with the optical tweezers reveals the cytoplasmic stiffness. Most importantly, the technique is insensitive to the mechanics of the actin cortex, which lies underneath the membrane and is a major contributor to cell surface tension. Moreover, the interior of a cell is inherently heterogeneous, thus requires carefulness in identifying which cellular moieties govern the reaction. Nonetheless, this technique provides the closest to insights into sub-cellular mechanics within cell spheroids.
5 Matrix Mechanophenotype
What about local inhomogeneities? What about non-linearity of mechanical properties of the extracellular matrix? What about the dynamic nature of the mechanophenotype? There exists large inhomogeneities in hydrogels based on biopolymers, such as collagen matrices [167]. These inhomogeneities rely mainly on the different sources of collagen and may subsequently be based on extraction techniques of collagen type I from tissues of different organisms.
Apart from the capability to restructure the extracellular matrix through proteolytic break-down, cancer cells can employ alternatively cellular force to mechanically remodulate the extracellular matrix environment, when these cells migrate across barriers of tissue [325–327]. Although it has been revealed that the assembly of invadopodia and their activity can be governed through the rigidity of the extracellular matrix [328,329,331,332], it is currently unclear whether invadopodial structures assembled in concert with collagen fibers are imbued with matrix-deforming activity that imparts forces to the ambient extracellular matrix. Moreover, the mechanisms through which invasive cells orchestrate topological and mechanical inputs from the 3D extracellular matrix milieu with the organization and operation of invadopodia throughout matrix break-down and invasion are still mostly unclear. To elucidate this issue, the ultrastructural arrangement, dynamics, and mechanical features of invadopodia arising at the boundary layer between breast cancer cells and fibrillar type I collagen require assessment. Employing a combination of platinum replica electron microscopy, collagen type I fiber tracking and laser-based scission of collagen fibrils, there exists a protease-independent function for MT1-MMP in guiding the polymerization of actin and the generation of force at the leading edge of invadopodia [31]. These results strongly endorse a paradigm shift in invasion in which self-assembling force-generating proteolytic cell-matrix contacts encourage expansion of matrix pores to favor cancer cell invasion.
5.1 Perlecan
Perlecan constitutes a large multiplex multipurpose heparan sulfate proteoglycan abundant in vascularized tissues, however, it is also prevalent in low- and non-vascularized connective tissues including articular cartilage, intervertebral disc, meniscus, ligament, and in tendon in the form of a hybrid in which at least one of its heparan sulfate glycosaminoglycan chains is substituted with a chondroitin sulfate chain [374,375]. Smooth muscle cells (SMCs) produce a chondroitin sulfate/heparan sulfate hybrid version of perlecan, while keratinocytes in epithelial tissues produce a version comprising chondroitin sulfate, heparan sulfate, and keratan sulfate [376], and endothelial cells-perlecan has been found monosubstituted with heparan sulfate. Mast cells generate perlecan specimens with reduced molecular weight nuclear proteins [377] which appear to be caused through alternative splicing and/or protease scission in immunoglobulin-rich domain IV.
Perlecan acts to coordinate tropoelastin and aids in the assembly of elastic microfibrils into translamellar transverse bridges that, along with fibrillin and elastin, work to stabilize the extracellular matrix of the annulus fibrosus of the intervertebral disc. Pericellular perlecan interfaces with collagen VI and XI to delineate and solidify this matrix partition, which assumes a strategic location that facilitates two-way cell-matrix communication linking the cell to its broader extracellular matrix. Evidence from the extracellular matrix is transmitted through this pericellular matrix all the way down to the chondrocyte, so that it can sense and react to subsequent subtle alterations in the microenvironment in an effort to adjust tissue homeostasis. Therefore, perlecan has a crucial modulatory function in chondrocyte metabolism and differentiation. Perlecan functions as a carrier proteoglycan that transports low soluble lipid-modified proteins including the Wnt or Hedgehog family and aids in the formation of tissue morphogen gradients that propel tissue embryogenesis. Cell surface perlecan on endothelial cells or osteocytes serves as a flow detector within the blood [378] and lacunar duct fluid, supplying responses to smooth muscle cells that adjust vascular tone and blood pressure, and the regulation of bone turnover through osteocytes, illustrating the multiple functions of perlecan in load-bearing connective tissues [379]. Cell-assembled extracellular matrix frameworks composed of perlecan-elastin and perlecan-elastin-collagen type VI are both firm and compliant and account for the viscoelastic material characteristics of tension- and load-bearing connective tissues.
5.2 Heparansulfate Proteoglycan
Heparan sulfate/hyaluronan proteoglycans are engaged in numerous tasks spanning from the development and maintenance of microstructure to the organization of the extracellular matrix and basement membrane through attachment to matrix molecules including collagen IV, laminin and fibronectin [380,381]. In specific detail, heparan sulphate alters cell-cell interplay through serving as a co-receptor for various cell surface receptors and impacting cell-extracellular matrix interactions. In addition, heparan sulfate can promote the sequestration of several growth factors, chemokines, cytokines, morphogens, and enzymes that create a sheltered pool that, when liberated, can foster receptor-ligand signaling compounds to impart key regulatory actions in cellular events to sustain tissue homeostasis [382]. Structural alteration of heparan sulfate can result posttranslationally through the function of sulfotransferases, sulfatases, and heparanase. MMPs and other proteolytic enzymes, such as plasminogen, can change the core of heparan sulphate proteoglycans and hence, can govern heparan sulphate proteoglycan-dependent signal transduction processes [383,384]. They play a role in cancer progression [385].
6 Hallmarks of the Mechanophenotype Transition
How solid tumors gain the capacity to metastasize at distant locations is not well comprehended. A driver of metastasis by the enemy microenvironment may be that it provokes the stress reactions itself, which leads to hypervariability of ordinary routines that govern cell migration, nutrient absorption, and the tissue organization [11]. The habit variability may impart survival benefits and permit flexibility to accommodate fairly major program adjustments under stress. Imagining a solid tumor as not just a haphazardly altering tumbling ball of cells, but as an evolving system that is subject to adaptation to survive in the body through kidnapping and merging of intrinsic diversity programs, gives rise to a different way of conceiving of what is behind complicated phenomena such as cancer metastasis. Several cellular processes could be susceptible to such selection and hypervariability induction under stress, though the focus is set on tissue organization, cell migration, and nutrient acquisition through macropinocytosis, that utilizes the cellular migration machinery [11].
Cells can evade primary cancers in either an individual or a collective fashion. Single cells produce protrusions, regulate their adhesion, and exploit their actomyosin cytoskeleton to generate forces, propel themselves through physical restraints and traverse the extracellular matrix. Specifically, cells characteristically institute an anterior-posterior polarization through positive and negative loopback mechanisms that establish master sites of actin assembly, adhesion, or contractile force production. The Rho GTPase family of proteins has a key function in controlling single-cell migration through coordinating the activation and dynamics of a number of actin cytoskeleton-regulating proteins. While the regulatory nature of the interconnected webs is intricate, Rac1 is characteristically assumed to primarily regulate actin-based protrusions [52], RhoA primarily conveys actomyosin contractility and Cdc42 orchestrates the polarity of adhesion, contractility, and cell protrusion [386]. Cell polarity emerges from asymmetry in the allocation and arrangement of cell constituents (Figure 10). The polarity is an essential characteristic of all living organisms, and a lot of energy is spent on breaking the symmetry and creating the polarity. There are parallels between planar cell polarity and apical-basal polarity in epithelial tissues and face-back polarity in motile cells.
6.1 Phase Separation as a Hallmark
The route of signal transduction in the course of innate and adaptive immunity are critical for the defense of pathogen and especially in their identification and the overall functional roles of immune cells. Assemblies of higher order have evolved lately as a core operating mechanism that governs immune signaling and, consequently, cellular intercommunication in more general terms. Primarily, higher-order assemblies exist in two types: on the one hand, ordered, solid-like large supramolecular aggregates generated through stable and rigid protein-protein interactions, and on the other hand, liquid-like phase-separated condensates generated through softer and more dynamic intermolecular interactions (Figure 10) [387].
The structure and dynamics of a variety of higher-order assemblies, among them amyloids, several types of signalosomes, and intracellular granules. It has been hypothesized that the synergy between folded domains, linear motifs, and regions of intrinsic disorganization governs the creation and intrinsic uncertainty of all higher-order assemblies, generating a textural and dynamic continuum [387].
With the acquisition of the initial protein crystal patterns, the structure-function paradigm emerged, where a folded protein structure dictates the proper operation of a protein. Which has been recently further refined by the MeshCODE theory [388]. Growing evidence from different areas of structural biology revealed that protein function also emanates from their intrinsically disordered regions that do not exhibit a coherently well-defined conformation, extending the classical view of how structure dictates function [389,390]. Some sequences in intrinsically disordered regions are repeats and carry little content of information in comparison to folded proteins. Such domains are often termed low complexity domains [391]. Intrinsically disordered regions can wrinkle into a singular conformation when bound to another protein or during oligomerization to carry out their designated purpose. Alternatively, these can convolve into sets of structured conformations or stay extensively disordered and demonstrate a fast swapping of conformations even in the intricate regime, both of those have been termed fuzzy structures [392].
6.2 Fuzzy Structures as a Hallmark of Transition of Cellular Functions
In tandem with growing structural abundance, higher-order intricacies have arisen over a wide array of the biological scene, comprising amyloids and prions [393], several types of signaling complexes commonly referred to as signalosomes [394,395], and nuclear and cytoplasmic granules [396–398].
For instance, signalosomes possess elevated local concentrations of protein-binding sites, and thereby offer a high avidity for low-affinity ligands, which then foster the signal transduction processes [394]. Consequently, signalosomes have serially shifted the manner in which the entire field views cellular assembly and signal transduction in a collective manner [395,399].
Intracellular organelles consist of membrane-bound vesicles or membrane-free compositions made up of proteins and RNA. These organelles perform pivotal biological functions to compartmentalize the cell for spatial and temporal organization of biological actions. Emerging evidence indicates that membrane-free intracellular compartments are multiphase viscous fluid droplets that develop through phase separation. Proteins with an intrinsic propensity to be conformally heterogeneous tend to be the principal carriers of liquid-liquid phase separation inside the cell. These results illustrate the pertinence of traditional ideas from the physics of polymer phase transitions for gaining an in-depth insight into the structure of intracellular membrane-free compartments [399].
These complexes are distinct from the majority of conventional macromolecular complexes in that their constituent proteins frequently polymerize or interconnect, which leads to diverse stoichiometries and heterogeneous conformational features. There is a fundamental question to be answered: are there shared biophysical principles at stake in the various kinds of higher-order structures? Because insights from one type of structure can influence the functional mechanisms of the other, they were therefore surveyed jointly for mechanistic evidence. Folded domains, linear motifs, and intrinsically unordered domains have been found to be synergistic with one another in higher-order assemblies, creating integrally fuzzy structures. These complexes may share a common critical concentration for their assemblage, however, they display distinct structural and dynamic characteristics as a function of the basic underlying assembly machinery and regulatory regime (Figure 10).
6.3 Mechanics-Induced Invadopodia Formation
Live cell imaging revealed that Tks5GFP-positive invadopodia at the ventral cell surface appeared to be highly dynamic, with a mean lifespan of approximately 41 ± 1.7 min, and that they expanded alongside the subjacent collagen fiber at a speed of 0.15 ± 0.02 µm/min, forming characteristic arc-shaped or circular structures [31]. Temporal sequences revealed that invadopodia/collagen fiber assemblages homothetically elongate over time, with an overall mean radial speed of 0.16 ± 0.02 µm/min [31]. Supplementing this type of activity, in many cases there has been seen proteolytic breakage and pushback of the assembly of invadopodia and collagen fibers. The fiber relaxation analysis plotted as a function of time yielded a characteristic viscoelastic performance of the invadopodia/fiber complex that exhibits an initial speed of V0 = 3.1 ± 0.22 µm/min, typifying the relationship of stress to resistance of the fiber [400]. Collectively, these findings indicate a powerful rearrangement ability of collagenolytic invadopodia on the basis of MMP activity and demonstrate that collagen fibers affiliated with invadopodia maintain mechanical tension and bending moment that unwind during proteolytic breakage, revealing that cells at the level of invadopodia generate and transmit force toward the fibers.
7 Migratory Phenotype
Cell migration is a basic mechanism in multicellular organisms and is characterized by a concerted motion of individual or multiple cells in a certain direction and to a specific location. Embryo development, healing of wounds, and proper operation of the immune system all demand cell movement in multicellular organisms, underscoring their essential contribution to life. Routes fueling migratory activity can be switched on or off according to prevailing conditions, nevertheless adult epithelia, the source of numerous solid cancers or carcinomas, exhibit restricted migration and are prone to stick to adjacent cells in an organized fashion.
7.1 Single Cell Migration
Single cell migration is regulated through the Rho GTPase Rac1, which interfaces with the Scar/WAVE complex, leading to activation of Arp2/3 and exploding nucleation of ramified actin filaments [401]. As the earliest de novo actin nucleator to be detected, the Arp2/3 complex is a key actor within protrusive force production models through the dynamic actin meshwork. Actin nucleation centers agglomerate with receptors and associated signaling scaffold proteins owing to multivalent binding interactions, producing domains that are similar to phase separations [402,403]. Transmembrane receptors at the cell membrane surface frequently cluster at nanometer to micrometer scales to engage in signal transduction in reaction to environmental stimuli. Extracellular ligand oligomerization, domain-domain interferences, and binding to multivalent proteins are all factors conducive to cluster development. There are specific mechanisms that foster the assembly of clusters in several prominent receptor systems, encompassing glycosylated receptors, cell adhesion receptors, immune receptors, receptor tyrosine kinases and Wnt receptors. These clusters have been proposed to share characteristics of systems that perform a liquid-liquid phase separation and therefore can be analyzed in this regard (Figure 10) [402]. These clusters facilitate persistent local actin polymerization and can initiate crosstalk with other signaling molecules, permitting cells to outwardly extrude the plasma membrane and generate outgrowths including lamellipodia, filopodia, and invadopodia.
7.1.1 Phase Separation in the Cytoplasm
Biomolecular condensates congregate macromolecules in mounts lacking a circumferential membrane. Multiple multivalent exchanges occur in most condensates, leading to a phase separation of the liquid and the fluid. The liquid-liquid phase separation enhances the intrinsic activity of actin-regulatory proteins for the assembly of actin through the Arp2/3 complex. This elevation has been observed to occur due to the liquid-liquid phase separation of the Nephrin-Nck-N-WASP signal transduction route on lipid bilayers. Thereby, the membrane dwell time of N-WASP and Arp2/3 complex is raised, which subsequently enhances the assembly of actin structures [403]. The residence time depends on the relative stoichiometry of the signaling proteins in the phase-separated clusters, resulting in stoichiometry-dependent N-WASP and Arp2/3 activity. This mechanism for governing protein activity is facilitated due to the stoichiometrically indeterminate character of the biomolecular condensates. Such control should be a common attribute of signaling schemes that are constituted via multivalent exchanges and yield non-equilibrium outcomes [403].
Biomolecular condensates are membrane-free cellular subcompartments that frequently arise from liquid-liquid phase partitioning fueled through intermolecular multivalent cross-talk. Phase separation of liquid from liquid has been identified in processes as divergent as gene regulation, autophagy, and signal transduction at membrane receptors. Precisely how the generation of biomolecular condensates governs these processes is not clear. Two recent investigations revealed that biomolecular 2D condensates of membrane-associated signaling compounds enhance the dwell time of signaling compounds within these complexes at the membrane, which is termed membrane dwell time, and thus control their activity ([403,404]).
Analysis of the local assembly of F-actin at membrane-associated biomolecular 2D condensates revealed that the generation of these condensates is based on specific interactions resembling those of the LAT-GRB2-SOS system, albeit with a different group of proteins participating, including phosphorylated nephrin (membrane receptor), NCK (adaptor protein), and N-WASP (NCK-interacting protein and activator of the actin nucleation factor Arp2/3 complex). Arp2/3-driven actin polymerization has been identified to be increased within condensates compared to levels outside of condensates, which has been inferred to be due to elevated N-WASP activity present within condensates. Resembling SOS, this upregulation of N-WASP activity actually directly covariates with enhanced membrane residence time of N-WASP (and also Arp2/3) inside the condensates. Significantly, the retention time of the N-WASP membrane responded sensitively to NCK concentration: as the NCK concentration increases, the residence time initially lengthened but subsequently declined beyond a saturation point. This saturation point presumably mirrors the depletion of phosphorylated sites on nephrin with consequent loss of multivalent connectivity, leaving N-WASP garnished with free NCK that is not linked to phospho-nephrin. Hence, the relative stoichiometry of the condensate constituents - through mutually influencing multivalent reactions inside the condensate - finely tunes their activity and regulates their dwell time within the signaling compounds. Since multivalent interactions and multistep reaction cascades are prevalent in signal transduction paths, these tenets of signal regulation through biomolecular condensates are probably universally valid. Subsequently, it can be concluded that the mechanophenotype of cells can be altered by phase-separation of cytoplasmic liquid components.
7.1.2 Phase Separation in the Nucleus
Besides the phase separation in the cytoskeleton, there exist a liquid-liquid phase separation in the nucleus [405]. In the majority of previous experimental setups, the nuclear-scale phase separation between chromatin and aqueous phase in the nucleus has not been accounted. Nevertheless, recent super-resolution microscopy indicates a pool of chromatin and aqueous phase with a non-uniform dispersion of chromatin at the submicron length scale [406]. In a different investigation, greater phase separation of chromatin and the aqueous phase has been noticed in early development, with chromatin situated at the nuclear periphery [407]. Theoretically, chromatin can be located near the lamina layer of the nuclear envelope, occupy the nucleus in a traditional fashion, be arranged in the center, or be available as wetting droplets. These transitions are governed through alterations in nuclear volume and the engagement of chromatin with the lamina, which is part of the nuclear envelope at the nuclear periphery. On the basis of a simple polymer model that encompasses the main characteristics of chromatin self-attraction and its attachment to the lamina, it can be theoretically demonstrated that the type of chromatin partitioning is governed by the rivalry of these two actions [408]. Chromatin is an intricate, linear macromolecule composed of DNA and histone proteins that is resident in the nucleus of eukaryotic cells, where it is dissolved by water, salts, and other small molecules [409]. In multiple investigations, the organization of chromatin in interphase cells appears to be homogeneous at nuclear length scale. According to this traditional view, the chromatin and its aqueous diluent fill the nucleus homogeneously as a single phase [410]. In fact, even the context of the conventional screen exhibits a phase separation resembling that of soluble AB-block copolymers [411], with areas of transcriptionally active euchromatin (A-block) divided from areas of relatively inactive heterochromatin (B-block), both of which are abundant in equal amounts.
7.2 Collective Cell Migration
Cells that form a tissue migrate as members of a collective. To coordinate collective multicellular navigation, each individual cell assimilates local input, incorporating chemical cues and mechanical stresses [264]. The border between a component cell and its immediate adjacent cells comprises cell-cell junctions and cryptic lamellipodia [412], however, the condition of local mechanical stress applied at this interface is not amenable to experimentation. It is therefore not evident how collective mechanical events can be orchestrated over length scales that encompass large multicellular assemblies. Within the cell layer, for instance, a cell monolayer, there are unpredictable fluctuations of the mechanical stress, which are strong, occur instantaneously and propagate across the monolayer. These fluctuations clearly delineate a fractured stress scene that grows more heterogeneous, inertial, and cohesive as the density of the whole system grows. Local cell migrations move alongside local alignments of the maximum principal stress inside this sustained fractured stress scene. Therefore, it can be postulated that collective migration is guided through a straightforward but standardizing physiological rationale: neighboring cells converge to transfer a noticeable intercellular normal stress through local cell-cell junctions, while migrating down alignments with minimal intercellular shear stress.
High-throughput genomic approaches have pinpointed molecular actors and depicted their engagement in broad signaling pathways [413]. However, in spite of receiving in-depth signaling and structural insights, the role of intercellular adhesion in collective migration is controversial [414], and a lack of predictive power is inherent in what is known about collective cell migration, leaving it largely descriptive.
A major reason for these constraints is the lack of a physical picture linking cell movement to mechanical stresses occurring within the cell body and at cell-cell interfaces; these stresses have never been gauged. A high-resolution card of these stress constituents inside a moving monolayer has been presented, which acts as a basic experimental model scheme [415]. These stress patterns indicate that the regional cellular trajectory tracks local stress fields that are highly heterogeneous and interact drastically over ranges that span across multiple cell bodies. In combination, these results point to an unexpected but standardizing physiological principle, specifically, that every cell is prone to migrate and rearrange in a manner that minimizes local intercellular shear stress. In-depth familiarity with the biology of the cell-cell junction, the cryptic lamellipodium, or a particular molecular occurrence could certainly not ever anticipate such a standardizing principle, as it is an emergent characteristic of a multicellular collective framework. By comparison with the familiar single-cell guided mechanisms of chemotaxis, durotaxis, and haptotaxis, this disparate but inherently collective mechanism is termed plithotaxis [415].
Monolayer Stress Microscopy has been designed to quantify the local stress level inside a monolayer. Using an inverted optical microscope, it is possible to record the cell-generated shifts of fluorescent markers incorporated near the surface of a collagen-coated PAA gel support to that the cells adhere. Based on these dislocations, a chart of the tensile forces acting on the gel exerted by the monolayer is obtained [343]. Ultimately, on the basis of these tensile forces determined locally at the cell-substrate boundary, a simple equilibrium of forces, as required by Newton’s laws, can be employed to evaluate the distribution of mechanical stresses throughout the cell layer. The slope of these stresses across the cell layer results from the accumulation of tensile forces acting on the undersurface of the cells. The recorded stresses in the single-layer film reflect the average values over the entire cell layer thickness. At any point within the patch, the local coordinate system can be flipped in the cell plane to locate the specific alignments along which the local normal stress is maximum and minimum, respectively, thereby delineating the two principal stress elements, such as maximum and minimum stress items, and the two corresponding principal alignments that are orthogonal to one another. Therefore, the accompanying monolayer stress microscopy output shows every single component of the stress tensor in the layer in high resolution and images them individually.
Since cells spread cryptic lamellipodia [412] and move forward across the monolayer, stresses need to be in mechanical equilibrium at all points and at all times. However, there is not yet a mechanistic setting or physical framework that ties these stresses to cellular reorientation, restructuring, or migration. In contrast, in the instance of an isolated single cell, uniaxial strain typically results in the cell realigning at a certain angle based on the amount of applied stress, ranging from parallel to vertical, based on the time scale of the mechanical perturbation. At this point, in the instance of monolayers, a question is to what extent intercellular tensions are biologically reasonable and beneficial for forecasting. The response to this question is implied from two pieces of experimental proof. Firstly, because phase contrast images and stress maps are unrelated measurements, the correspondence between the alignment of the cell body and the orientation of the maximum principal stress is remarkable. There are more tensile stress and zero shear stresses. Secondly, cells may not merely align themselves with the maximal principal orientation, however, they additionally move alongside that orientation. However, it seems to be not attributable to inhomogeneous stresses, since the stress field is more or less isotropic.
Cooperative movements arise in a natural way in inert particle systems that feature tight packing, structural disorganization, and glassy dynamics, such as colloidal glasses [416]. A key characteristic that identifies these schemes as glassy is the deceleration of internal structural reordering with increasing system density. As the system density grows, each participant is progressively enclosed by its surrounding particles, so that many adjacent particles need to be relocated in a cooperative manner in order to be capable of being relocated at all [417]. Therefore, the size of the cooperative system clusters grows as system density rises. In addition, the number of possible structural rearrangements diminishes with increasing cluster size, so that the time required for cooperative relocations jumps until the system ultimately gets effectively frozen or stalled [417]. Could the monolayer cell layer reveal such evidence of glassy dynamics? Even though a mechanistic relationship between particle-to-particle forces and spatially heterogeneous dynamical behavior in glassy systems continues to be ambiguous [418], the results are in line with the convergence towards a glass transition [415].
A pivotal question in morphogenesis and disease is of how distinct structures arise from uniform populations of cells [285]. Differentiation and pattering in multicellular systems is presently accounted for by the presence of morphogenetic gradients and through local variability in the constitution, topology, and rigidity of the extracellular matrix [419]. Moreover, the spontaneously generated, fractured stress landscape referred to here, when transmitted through the sensory scheme of the individual cell [420], is anticipated to induce uneven release of soluble or insoluble proteins, thereby changing the local cellular microenvironment, resulting in cytoskeletal reinforcement [421] or fluidization of the cytoskeleton [422], and activate stress-dependent genetic programs in a highly nonuniform fashion, yielding differentiated tissues.
8 Conclusion and Future Directions
Although there have been many efforts to analyze mechanotransduction within cells, one was still at the beginning of understanding the entities complex play. Although cellular responses and their mechanophenotype are altered by changes in the mechanophenotype of their microenvironment, it has been found that the matrix mechanophenotype is also altered by the cells. This bidirectional response interaction has just begun to be incorporated into cell and tissue models and, in particular, the effects of cells on the mechanophenotype of extracellular matrices has been increasingly studied. In addition, the initial mechanical analyses were mostly time point dependent in a 2D microenvironment, which is now changing to studies in a 3D microenvironment and is a tremendous advantage. Similarly, time point measurements are rather outdated. In the future, the focus should be on dynamic analysis of mechanical properties, with the addition of another 4-dimensionality, time. Fluctuations in mechanophenotype can thus be translated, and changes in the amplitude of these fluctuations can also provide insights into the overall regulatory scheme. This includes a particular focus on phase transitions in the cytoplasm and nucleus. Moreover, thereby play also hydrogel-based 3D scaffolds a crucial role that can be easily and reproducibly mechanical probed by using chemical, natural or physical techniques. Moreover, the embedment of immune and stromal cells provides an additional crucial element to explore the effect of cell-derived factors, such as matrix-embedded by-stander cells or cells under investigation, such as cancer cells.
A recognized gap in scientific knowledge is the mechanism by which cells, such as non-tumorigenic epithelial cells and cancer cells, respond to and integrate acute mechanical stress. It should be noted that certain mechanical stresses can be more severe and dynamic than others. New biophysical techniques to examine the effects of mechanical stress in normal and malignant epithelial cells may yield new glimpses into the prospective contribution of fast mechano-chemical signaling, which has been shown to be effective in a number of different cell types.
To change the mechanophenotype in a precisely controlled manner, it seems necessary to alter the crosslinking efficiency, which affects multiple hydrogel characteristics, comprising pore size, rigidity, and sensitivity of the hydrogel to enzymatic break-down and matrix deposition. Specifically, more dedicated molecules implicated need to be identified that regulate the process of signal transduction from the outer extracellular matrix environment to the interior of the cell. Thereby, signaling directly or indirectly into the nucleus, where transcription of genes in affected in order to respond to the mechanical constraints evoked by the local surrounding microenvironment. Consequently, the cellular investigation levels need to be on different length scales, such as organoid, cellular, cytoskeletal and nuclear length scales including the alteration of gene transcription.
Frequently, architecture, mechanotransduction, and cellular performance are tightly coupled. The present difficulty is to discriminate the multiple target labels in the process of interplay between microenvironment and cellular functions. In this regard, it is still not clear, in part, how the current components generate and translate the physical force to alter cytoskeletal rearrangement and cellular functionalities. Another major challenge is the lack of available techniques to quantify the specific mechanical pressure in the course of perturbation of the mechanophenotype of the extracellular matrix environment. Moreover, knowledge of the fundamental mechanisms of the mechanical characteristics of the microenvironment contributes to advances in the treatment of injuries, malignancies, and diseases associated with alterations in the mechanophenotype of the extracellular matrix. A recently developed biophysical technique is a method that paves the way for monitoring both extracellular stiffness and dynamic mechanical stress by cladding cells on substrates of varying stiffness in conjunction with transient stretching of the substrate or “tugging” by magnetic manipulations [423].
Beyond the development of biophysical techniques, it needs to be clarified whether there exist universal hallmarks of the migratory behavior of cells that can be defined on the basis of the mechanophenotype. This appears to be the most complicated question to address and is likely to require the greatest future effort.
Another, less important, concern is that most synthetic hydrogel systems employed in this research discipline are nanoporous and lack the fibrillarity and ligand appearance of native extracellular matrices. Integrating collagen fibers into synthetic hydrogels [424,425], or employing synthetic approaches to engineer collagen-like fibers [426], has the potential to aid in overcoming this critical restriction. Integrating advancements in chemical synthesis pathways that enable a more explicit level of control over the constitution, structure, and accurate placement of functional moieties [427,428] may further help to overcome these concerns. In addition, RAFT or DNA origami [429] and noninvasive tailoring of characteristics in real time [430], with adaptive fabrication techniques that are capable of programming material constitution and architectural design over multiple length scales [431] are expected to afford new material frameworks for examining the effects of viscoelasticity and viscoplasticity in both in vitro and in vivo settings. Thereby, also theoretical models need to be addressed, modified and newly developed.
Finally, there are prosperous future directions that can be envisioned for the field of cell migration and invasion, when the microenvironment is taken into account. Overall, the utilization of spheroids has been identified as a feasible vehicle for the assessment of nanomedicines under circumstances that more accurately mirror the in vivo tumor microenvironment than conventional monolayer studies [240]. By customizing conventional cell-based assays, spheroids have the inherent capability to act as a middleman between conventional in vitro and in vivo testing models for high-throughput assessment of therapeutic contenders. These spheroids or organoids also allow the application of biophysical methods.
A future research avenue is that these interactions of extracellular matrix composition, influenced by target cells or neighboring cells, are implicated in the generation of supramolecular assemblies such as collagen fibrils and elastic fibers, in tissue architecture, and in cell-matrix interactions that in turn govern cell growth and behavior. Consequently, the emphasis is on interdisciplinary research, the development of new biophysical techniques, and the discovery of key principles of cellular constituents, the mechanoduction process and the directional interplay between cells and their microenvironment.
Author Contributions
CTM designed and wrote the manuscript and prepared all figures.
Conflict of Interest
The author declares that the research was conducted in the absence of any commercial or financial relationships that could be construed as a potential conflict of interest.
Publisher’s Note
All claims expressed in this article are solely those of the authors and do not necessarily represent those of their affiliated organizations, or those of the publisher, the editors and the reviewers. Any product that may be evaluated in this article, or claim that may be made by its manufacturer, is not guaranteed or endorsed by the publisher.
Acknowledgments
The author acknowledges support from the German Research Foundation (DFG) and Universität Leipzig within the program of Open Access Publishing, and Thomas M. L. Mierke for critical proof reading of the manuscript.
Abbreviations
3D, three-dimensional; ADAMs, A Disintegrin And Metalloproteinases; CT, cytoplasmic tail; DHT, dehydrothermal treatment; EDC-NHS, 1-ethyl-3-(3-dimethylaminopropyl) carbodiimide-N-hydroxysuccinimide; EMT, Epithelial-to-mesenchymal; FAK, focal adhesion kinase; FGF, fibroblast growth factor; GFP, Green fluorescent protein; GPI, glycosylphosphatidylinositol; LOX, lysyl oxidase; MIDAS, metal ion dependent adhesion site; MMPs, matrix metallo-proteinases; NMHC II, non-muscle myosin heavy chain II; PAA, polyacrylamide; PDGF, platelet-derived growth factor; PDMS, polydimethyalsiloxane; PSGL-1, P-selectin glycoprotein ligand-1; TEPIC, tris (2,3-Epoxy propyl) isocyanurate; TG2, transglutaminase 2; TGF-β, transforming growth factor-β; TNF, tumor necrosis factor; UV, ultraviolet radiation; VCAM-1, vascular cell adhesion molecule-1.
References
1. Charras G, Sahai E. Physical Influences of the Extracellular Environment on Cell Migration. Nat Rev Mol Cel Biol (2014) 15(12):813–24. doi:10.1038/nrm3897
2. Mierke CT. The Matrix Environmental and Cell Mechanical Properties Regulate Cell Migration and Contribute to the Invasive Phenotype of Cancer Cells. Rep Prog Phys (2019) 82(6):064602. doi:10.1088/1361-6633/ab1628
3. Mierke CT. The Fundamental Role of Mechanical Properties in the Progression of Cancer Disease and Inflammation. Rep Prog Phys (2014) 77(7):076602. doi:10.1088/0034-4885/77/7/076602
4. Mierke CT, Kollmannsberger P, Zitterbart DP, Diez G, Koch TM, Marg S, et al. Vinculin Facilitates Cell Invasion into Three-Dimensional Collagen Matrices. J Biol Chem (2010) 285(17):13121–30. doi:10.1074/jbc.M109.087171
5. Lämmermann T, Sixt M. Mechanical Modes of 'amoeboid' Cell Migration. Curr Opin Cel Biol (2009) 21(5):636–44. doi:10.1016/j.ceb.2009.05.003
6. Reversat A, Gaertner F, Merrin J, Stopp J, Tasciyan S, Aguilera J, et al. Cellular Locomotion Using Environmental Topography. Nature (2020) 582(7813):582–5. doi:10.1038/s41586-020-2283-z
7. Lu D, Kassab GS. Role of Shear Stress and Stretch in Vascular Mechanobiology. J R Soc Interf (2011) 8(63):1379–85. doi:10.1098/rsif.2011.0177
8. Barriga EH, Franze K, Charras G, Mayor R. Tissue Stiffening Coordinates Morphogenesis by Triggering Collective Cell Migration In Vivo. Nature (2018) 554(7693):523–7. doi:10.1038/nature25742
9. Kim BG, Gao M-Q, Kang S, Choi YP, Lee JH, Kim JE, et al. Mechanical Compression Induces VEGFA Overexpression in Breast Cancer via DNMT3A-dependent miR-9 Downregulation. Cell Death Dis (2017) 8(3):e2646. doi:10.1038/cddis.2017.73
10. Chaudhuri O, Cooper-White J, Janmey PA, Mooney DJ, Shenoy VB. Effects of Extracellular Matrix Viscoelasticity on Cellular Behaviour. Nature (2020) 584(7822):535–46. doi:10.1038/s41586-020-2612-2
11. Nikolaou S, Machesky LM. The Stressful Tumour Environment Drives Plasticity of Cell Migration Programmes, Contributing to Metastasis. J Pathol (2020) 250(5):612–23. doi:10.1002/path.5395
12.JC Gerhart, and J Norton, editors. The Plausibility of Life: Resolving Darwin’s Dilemma. London, England: Yale University Press (2008).
13. Friedl P, Wolf K. Proteolytic Interstitial Cell Migration: A Five-step Process. Cancer Metastasis Rev (2009) 28(1–2):129–35. doi:10.1007/s10555-008-9174-3
14. Pedersen NM, Wenzel EM, Wang L, Antoine S, Chavrier P, Stenmark H, et al. Protrudin-mediated ER-Endosome Contact Sites Promote MT1-MMP Exocytosis and Cell Invasion. J Cel Biol (2020) 219(8):e202003063. doi:10.1083/jcb.202003063
15. Naegeli KM, Hastie E, Garde A, Wang Z, Keeley DP, Gordon KL, et al. Cell Invasion In Vivo via Rapid Exocytosis of a Transient Lysosome-Derived Membrane Domain. Develop Cel (2017) 43(4):403–17.e10. doi:10.1016/j.devcel.2017.10.024
16. Castro-Castro A, Marchesin V, Monteiro P, Lodillinsky C, Rossé C, Chavrier P. Cellular and Molecular Mechanisms of MT1-MMP-Dependent Cancer Cell Invasion. Annu Rev Cel Dev. Biol. (2016) 32(1):555–76. doi:10.1146/annurev-cellbio-111315-125227
17. Eddy RJ, Weidmann MD, Sharma VP, Condeelis JS. Tumor Cell Invadopodia: Invasive Protrusions that Orchestrate Metastasis. Trends Cel Biol (2017) 27(8):595–607. doi:10.1016/j.tcb.2017.03.003
18. Linder S, Wiesner C, Himmel M. Degrading Devices: Invadosomes in Proteolytic Cell Invasion. Annu Rev Cel Dev. Biol. (2011) 27(1):185–211. doi:10.1146/annurev-cellbio-092910-154216
19. Murphy DA, Courtneidge SA. The 'ins' and 'outs' of Podosomes and Invadopodia: Characteristics, Formation and Function. Nat Rev Mol Cel Biol (2011) 12(7):413–26. doi:10.1038/nrm3141
20. Feinberg TY, Zheng H, Liu R, Wicha MS, Yu SM, Weiss SJ. Divergent Matrix-Remodeling Strategies Distinguish Developmental from Neoplastic Mammary Epithelial Cell Invasion Programs. Develop Cel (2018) 47(2):145–60.e6. doi:10.1016/j.devcel.2018.08.025
21. Hotary K, Allen E, Punturieri A, Yana I, Weiss SJ. Regulation of Cell Invasion and Morphogenesis in a Three-Dimensional Type I Collagen Matrix by Membrane-type Matrix Metalloproteinases 1, 2, and 3. J Cel Biol (2000) 149(6):1309–23. doi:10.1083/jcb.149.6.1309
22. Lodillinsky C, Infante E, Guichard A, Chaligné R, Fuhrmann L, Cyrta J, et al. P63/MT1-MMP axis Is Required for In Situ to Invasive Transition in Basal-like Breast Cancer. Oncogene (2016) 35(3):344–57. doi:10.1038/onc.2015.87
23. Sabeh F, Shimizu-Hirota R, Weiss SJ. Protease-dependent versus -independent Cancer Cell Invasion Programs: Three-Dimensional Amoeboid Movement Revisited. J Cel Biol (2009) 185(1):11–9. doi:10.1083/jcb.200807195
24. Wolf K, Wu YI, Liu Y, Geiger J, Tam E, Overall C, et al. Multi-step Pericellular Proteolysis Controls the Transition from Individual to Collective Cancer Cell Invasion. Nat Cel Biol (2007) 9(8):893–904. doi:10.1038/ncb1616
25. Artym VV, Swatkoski S, Matsumoto K, Campbell CB, Petrie RJ, Dimitriadis EK, et al. Dense Fibrillar Collagen Is a Potent Inducer of Invadopodia via a Specific Signaling Network. J Cel Biol (2015) 208(3):331–50. doi:10.1083/jcb.201405099
26. Juin A, Billottet C, Moreau V, Destaing O, Albiges-Rizo C, Rosenbaum J, et al. Physiological Type I Collagen Organization Induces the Formation of a Novel Class of Linear Invadosomes. Mol Biol Cel (2012) 23(2):297–309. doi:10.1091/mbc.e11-07-0594
27. Parekh A, Ruppender NS, Branch KM, Sewell-Loftin MK, Lin J, Boyer PD, et al. Sensing and Modulation of Invadopodia across a Wide Range of Rigidities. Biophysical J (2011) 100(3):573–82. doi:10.1016/j.bpj.2010.12.3733
28. Wolf K, te Lindert M, Krause M, Alexander S, te Riet J, Willis AL, et al. Physical Limits of Cell Migration: Control by ECM Space and Nuclear Deformation and Tuning by Proteolysis and Traction Force. J Cel Biol (2013) 201(7):1069–84. doi:10.1083/jcb.201210152
29. Castagnino A, Castro-Castro A, Irondelle M, Guichard A, Lodillinsky C, Fuhrmann L, et al. Coronin 1C Promotes Triple-Negative Breast Cancer Invasiveness through Regulation of MT1-MMP Traffic and Invadopodia Function. Oncogene (2018) 37(50):6425–41. doi:10.1038/s41388-018-0422-x
30. Monteiro P, Rossé C, Castro-Castro A, Irondelle M, Lagoutte E, Paul-Gilloteaux P, et al. Endosomal WASH and Exocyst Complexes Control Exocytosis of MT1-MMP at Invadopodia. J Cel Biol (2013) 203(6):1063–79. doi:10.1083/jcb.201306162
31. Ferrari R, Martin G, Tagit O, Guichard A, Cambi A, Voituriez R, et al. MT1-MMP Directs Force-Producing Proteolytic Contacts that Drive Tumor Cell Invasion. Nat Commun (2019) 10(1):4886. doi:10.1038/s41467-019-12930-y
32. Petersen NO, McConnaughey WB, Elson EL. Dependence of Locally Measured Cellular Deformability on Position on the Cell, Temperature, and Cytochalasin B. Proc Natl Acad Sci (1982) 79(17):5327–31. doi:10.1073/pnas.79.17.5327
33. Infante E, Castagnino A, Ferrari R, Monteiro P, Agüera-González S, Paul-Gilloteaux P, et al. LINC Complex-Lis1 Interplay Controls MT1-MMP Matrix Digest-On-Demand Response for Confined Tumor Cell Migration. Nat Commun (2018) 9(1):2443. doi:10.1038/s41467-018-04865-7
34. Abercrombie M, Heaysman JEM, Pegrum SM. The Locomotion of Fibroblasts in Culture I. Movements of the Leading Edge. Exp Cel Res (1970) 59(3):393–8. doi:10.1016/0014-4827(70)90646-4
35. Abercrombie M, Heaysman JE, Pegrum SM. The Locomotion of Fibroblasts in Culture. I. Movements of the Leading Edge. Exp Cel Res (1970) 59(2–3):393–8. doi:10.1016/0014-4827(70)90646-4
36. Abercrombie M, Joan E, Heaysman M, Pegrum SM. The Locomotion of Fibroblasts in Culture. Exp Cel Res (1970) 60(3):437–44. doi:10.1016/0014-4827(70)90537-9
37. Liotta LA. Tumor Invasion and Metastases-Rrole of the Extracellular Matrix: Rhoads Memorial Award Lecture. Cancer Res (1986) 46(1):1–7.
38. Friedl P, Wolf K. Plasticity of Cell Migration: A Multiscale Tuning Model. J Cel Biol (2010) 188(1):11–9. doi:10.1083/jcb.200909003
39. Page-McCaw A, Ewald AJ, Werb Z. Matrix Metalloproteinases and the Regulation of Tissue Remodelling. Nat Rev Mol Cel Biol (2007) 8(3):221–33. doi:10.1038/nrm2125
40. Sato H, Takino T, Miyamori H. Roles of Membrane-type Matrix Metalloproteinase-1 in Tumor Invasion and Metastasis. Cancer Sci (2005) 96(4):212–7. doi:10.1111/j.1349-7006.2005.00039.x
41. Humphrey JD, Dufresne ER, Schwartz MA. Mechanotransduction and Extracellular Matrix Homeostasis. Nat Rev Mol Cel Biol (2014) 15(12):802–12. doi:10.1038/nrm3896
42. Vogel V. Unraveling the Mechanobiology of Extracellular Matrix. Annu Rev Physiol (2018) 80(1):353–87. doi:10.1146/annurev-physiol-021317-121312
43. Segel M, Neumann B, Hill MFE, Weber IP, Viscomi C, Zhao C, et al. Niche Stiffness Underlies the Ageing of central Nervous System Progenitor Cells. Nature (2019) 573(7772):130–4. doi:10.1038/s41586-019-1484-9
44. Paszek MJ, Zahir N, Johnson KR, Lakins JN, Rozenberg GI, Gefen A, et al. Tensional Homeostasis and the Malignant Phenotype. Cancer Cell (2005) 8(3):241–54. doi:10.1016/j.ccr.2005.08.010
45. Tanner K, Mori H, Mroue R, Bruni-Cardoso A, Bissell MJ. Coherent Angular Motion in the Establishment of Multicellular Architecture of Glandular Tissues. Proc Natl Acad Sci (2012) 109(6):1973–8. doi:10.1073/pnas.1119578109
46. Meshel AS, Wei Q, Adelstein RS, Sheetz MP. Basic Mechanism of Three-Dimensional Collagen Fibre Transport by Fibroblasts. Nat Cel Biol (2005) 7(2):157–64. doi:10.1038/ncb1216
47. Cukierman E. Taking Cell-Matrix Adhesions to the Third Dimension. Science (2001) 294(5547):1708–12. doi:10.1126/science.1064829
48. Maaser K, Wolf K, Klein CE, Niggemann B, Zänker KS, Bröcker E-B, et al. Functional Hierarchy of Simultaneously Expressed Adhesion Receptors: Integrin α2β1 but Not CD44 Mediates MV3 Melanoma Cell Migration and Matrix Reorganization within Three-Dimensional Hyaluronan-Containing Collagen Matrices. Mol Biol Cel (1999) 10(10):3067–79. doi:10.1091/mbc.10.10.3067
49. Friedl P. Prespecification and Plasticity: Shifting Mechanisms of Cell Migration. Curr Opin Cel Biol (2004) 16(1):14–23. doi:10.1016/j.ceb.2003.11.001
50. Grinnell F, Ho C-H, Tamariz E, Lee DJ, Skuta G. Dendritic Fibroblasts in Three-Dimensional Collagen Matrices. MBoC (2003) 14(2):384–95. doi:10.1091/mbc.e02-08-0493
51. Friedl P, Bröcker E-B. The Biology of Cell Locomotion within Three-Dimensional Extracellular Matrix. Cell Mol Life Sci (Cmls) (2000) 57(1):41–64. doi:10.1007/s000180050498
52. Kunschmann T, Puder S, Fischer T, Steffen A, Rottner K, Mierke CT. The Small GTPase Rac1 Increases Cell Surface Stiffness and Enhances 3D Migration into Extracellular Matrices. Sci Rep (2019) 9(1):7675. doi:10.1038/s41598-019-43975-0
53. Giannone G, Dubin-Thaler BJ, Döbereiner H-G, Kieffer N, Bresnick AR, Sheetz MP. Periodic Lamellipodial Contractions Correlate with Rearward Actin Waves. Cell (2004) 116(3):431–43. doi:10.1016/S0092-8674(04)00058-3
54. Mitchison TJ, Cramer LP. Actin-Based Cell Motility and Cell Locomotion. Cell (1996) 84(3):371–9. doi:10.1016/S0092-8674(00)81281-7
55. Ridley AJ. Cell Migration: Integrating Signals from Front to Back. Science (2003) 302(5651):1704–9. doi:10.1126/science.1092053
56. Defilippi P, Olivo C, Venturino M, Dolce L, Silengo L, Tarone G. Actin Cytoskeleton Organization in Response to Integrin-Mediated Adhesion. Microsc Res Tech (1999) 47(1):67–78. doi:10.1002/(SICI)1097-0029(19991001)47:1<67::AID-JEMT7>3.0.CO;2-P
57. Yoshigi M, Hoffman LM, Jensen CC, Yost HJ, Beckerle MC. Mechanical Force Mobilizes Zyxin from Focal Adhesions to Actin Filaments and Regulates Cytoskeletal Reinforcement. J Cel Biol (2005) 171(2):209–15. doi:10.1083/jcb.200505018
58. Wang R, Wang Z, Millet L, Gillette MU, Levine AJ, Popescu G. Dispersion-relation Phase Spectroscopy of Intracellular Transport. Opt Express (2011) 19(21):20571. doi:10.1364/OE.19.020571
59. Ghosh S, Kollar B, Nahar T, Suresh Babu S, Wojtowicz A, Sticht C, et al. Loss of the Mechanotransducer Zyxin Promotes a Synthetic Phenotype of Vascular Smooth Muscle Cells. J Am Heart Assoc (2015) 4(6):e001712. doi:10.1161/JAHA.114.001712
60. Hirata H, Tatsumi H, Sokabe M. Zyxin Emerges as a Key Player in the Mechanotransduction at Cell Adhesive Structures. Communicative Integr Biol (2008) 1(2):192–5. doi:10.4161/cib.1.2.7001
61. Mierke CT, Fischer T, Puder S, Kunschmann T, Soetje B, Ziegler WH. Focal Adhesion Kinase Activity Is Required for Actomyosin Contractility-Based Invasion of Cells into Dense 3D Matrices. Sci Rep (2017) 7(1):42780. doi:10.1038/srep42780
62. Jay PY, Pham PA, Wong SA, Elson EL. A Mechanical Function of Myosin II in Cell Motility. J Cel Sci (1995) 108(Pt 1):387–93. doi:10.1242/jcs.108.1.387
63. Yeung T, Georges PC, Flanagan LA, Marg B, Ortiz M, Funaki M, et al. Effects of Substrate Stiffness on Cell Morphology, Cytoskeletal Structure, and Adhesion. Cell Motil. Cytoskeleton (2005) 60(1):24–34. doi:10.1002/cm.20041
64. Eastwood M, McGrouther D, Brown R. A Culture Force Monitor for Measurement of Contraction Forces Generated in Human Dermal Fibroblast Cultures: Evidence for Cell-Matrix Mechanical Signalling. Biochim Biophys Acta (1994) 1201(2):186–92. doi:10.1016/0304-4165(94)90040-x
65. Lee GM, Loeser RF. Cell Surface Receptors Transmit Sufficient Force to bend Collagen Fibrils. Exp Cel Res (1999) 248(1):294–305. doi:10.1006/excr.1999.4418
66. Golomb E, Ma X, Jana SS, Preston YA, Kawamoto S, Shoham NG, et al. Identification and Characterization of Nonmuscle Myosin II-C, a New Member of the Myosin II Family. J Biol Chem (2004) 279(4):2800–8. doi:10.1074/jbc.M309981200
67. Shohet RV, Conti MA, Kawamoto S, Preston YA, Brill DA, Adelstein RS. Cloning of the cDNA Encoding the Myosin Heavy Chain of a Vertebrate Cellular Myosin. Proc Natl Acad Sci (1989) 86(20):7726–30. doi:10.1073/pnas.86.20.7726
68. Simons M, Wang M, McBride OW, Kawamoto S, Yamakawa K, Gdula D, et al. Human Nonmuscle Myosin Heavy Chains Are Encoded by Two Genes Located on Different Chromosomes. Circ Res (1991) 69(2):530–9. doi:10.1161/01.RES.69.2.530
69. Bresnick AR. Molecular Mechanisms of Nonmuscle Myosin-II Regulation. Curr Opin Cel Biol (1999) 11(1):26–33. doi:10.1016/s0955-0674(99)80004-0
70. Kovács M, Wang F, Hu A, Zhang Y, Sellers JR. Functional Divergence of Human Cytoplasmic Myosin II. J Biol Chem (2003) 278(40):38132–40. doi:10.1074/jbc.M305453200
71. Wang F, Kovács M, Hu A, Limouze J, Harvey EV, Sellers JR. Kinetic Mechanism of Non-muscle Myosin IIB. J Biol Chem (2003) 278(30):27439–48. doi:10.1074/jbc.M302510200
72. Tullio AN, Accili D, Ferrans VJ, Yu Z-X, Takeda K, Grinberg A, et al. Nonmuscle Myosin II-B Is Required for normal Development of the Mouse Heart. Proc Natl Acad Sci (1997) 94(23):12407–12. doi:10.1073/pnas.94.23.12407
73. Üren D, Hwang H-K, Hara Y, Takeda K, Kawamoto S, Tullio AN, et al. Gene Dosage Affects the Cardiac and Brain Phenotype in Nonmuscle Myosin II-B-Depleted Mice. J Clin Invest (2000) 105(5):663–71. doi:10.1172/JCI8199
74. Kuragano M, Murakami Y, Takahashi M. Nonmuscle Myosin IIA and IIB Differentially Contribute to Intrinsic and Directed Migration of Human Embryonic Lung Fibroblasts. Biochem Biophysical Res Commun (2018) 498(1):25–31. doi:10.1016/j.bbrc.2018.02.171
75. Lee P, Lin R, Moon J, Lee LP. Microfluidic Alignment of Collagen Fibers for In Vitro Cell Culture. Biomed Microdevices (2006) 8(1):35–41. doi:10.1007/s10544-006-6380-z
76. Olazabal IM, Caron E, May RC, Schilling K, Knecht DA, Machesky LM. Rho-Kinase and Myosin-II Control Phagocytic Cup Formation during CR, but Not FcγR, Phagocytosis. Curr Biol (2002) 12(16):1413–8. doi:10.1016/S0960-9822(02)01069-2
77. Isik N, Brzostowski JA, Jin T. An Elmo-like Protein Associated with Myosin II Restricts Spurious F-Actin Events to Coordinate Phagocytosis and Chemotaxis. Develop Cel (2008) 15(4):590–602. doi:10.1016/j.devcel.2008.08.006
78. Haviv L, Gillo D, Backouche F, Bernheim-Groswasser A. A Cytoskeletal Demolition Worker: Myosin II Acts as an Actin Depolymerization Agent. J Mol Biol (2008) 375(2):325–30. doi:10.1016/j.jmb.2007.09.066
79. Humphrey D, Duggan C, Saha D, Smith D, Käs J. Active Fluidization of Polymer Networks through Molecular Motors. Nature (2002) 416(6879):413–6. doi:10.1038/416413a
80. Arora PD, Conti MA, Ravid S, Sacks DB, Kapus A, Adelstein RS, et al. Rap1 Activation in Collagen Phagocytosis Is Dependent on Nonmuscle Myosin II-A. MBoC (2008) 19(12):5032–46. doi:10.1091/mbc.e08-04-0430
81. Arora PD, Wang Y, Janmey PA, Bresnick A, Yin HL, McCulloch CA. Gelsolin and Non-muscle Myosin IIA Interact to Mediate Calcium-Regulated Collagen Phagocytosis. J Biol Chem (2011) 286(39):34184–98. doi:10.1074/jbc.M111.247783
82. Bos JL, de Bruyn K, Enserink J, Kuiperij B, Rangarajan S, Rehmann H, et al. The Role of Rap1 in Integrin-Mediated Cell Adhesion. Biochem Soc Trans (2003) 31(1):83–6. doi:10.1042/bst0310083
83. Fischer T, Wilharm N, Hayn A, Mierke CT. Matrix and Cellular Mechanical Properties Are the Driving Factors for Facilitating Human Cancer Cell Motility into 3D Engineered Matrices. Converg Sci Phys Oncol (2017) 3(4):044003. doi:10.1088/2057-1739/aa8bbb
84. Wei Q, Adelstein RS. Conditional Expression of a Truncated Fragment of Nonmuscle Myosin II-A Alters Cell Shape but Not Cytokinesis in HeLa Cells. Mol Biol Cel (2000) 11(10):3617–27. doi:10.1091/mbc.11.10.3617
85. Schiro JA, Chan BMC, Roswit WT, Kassner PD, Pentland AP, Hemler ME, et al. Integrin α2β1 (VLA-2) Mediates Reorganization and Contraction of Collagen Matrices by Human Cells. Cell (1991) 67(2):403–10. doi:10.1016/0092-8674(91)90191-Z
86. Nishizaka T, Shi Q, Sheetz MP. Position-dependent Linkages of Fibronectin- Integrin-Cytoskeleton. Proc Natl Acad Sci (2000) 97(2):692–7. doi:10.1073/pnas.97.2.692
87. Kelley CA, Sellers JR, Gard DL, Bui D, Adelstein RS, Baines IC. Xenopus Nonmuscle Myosin Heavy Chain Isoforms Have Different Subcellular Localizations and Enzymatic Activities. J Cel Biol (1996) 134(3):675–87. doi:10.1083/jcb.134.3.675
88. Kolega J. Asymmetric Distribution of Myosin IIB in Migrating Endothelial Cells Is Regulated by a Rho-dependent Kinase and Contributes to Tail Retraction. Mol Biol Cel (2003) 14(12):4745–57. doi:10.1091/mbc.e03-04-0205
89. Saitoh T, Takemura S, Ueda K, Hosoya H, Nagayama M, Haga H, et al. Differential Localization of Non-muscle Myosin II Isoforms and Phosphorylated Regulatory Light Chains in Human MRC-5 Fibroblasts. FEBS Lett (2001) 509(3):365–9. doi:10.1016/S0014-5793(01)03186-6
90. Brown ME, Bridgman PC. Retrograde Flow Rate Is Increased in Growth Cones from Myosin IIB Knockout Mice. J Cel Sci (2003) 116(6):1087–94. doi:10.1242/jcs.00335
91. Lo C-M, Buxton DB, Chua GCH, Dembo M, Adelstein RS, Wang Y-L. Nonmuscle Myosin IIB Is Involved in the Guidance of Fibroblast Migration. Mol Biol Cel (2004) 15(3):982–9. doi:10.1091/mbc.e03-06-0359
92. Dreymueller D, Pruessmeyer J, Groth E, Ludwig A. The Role of ADAM-Mediated Shedding in Vascular Biology. Eur J Cel Biol (2012) 91(6–7):472–85. doi:10.1016/j.ejcb.2011.09.003
93. van der Vorst EPC, Jeurissen M, Wolfs IMJ, Keijbeck A, Theodorou K, Wijnands E, et al. Myeloid A Disintegrin and Metalloproteinase Domain 10 Deficiency Modulates Atherosclerotic Plaque Composition by Shifting the Balance from Inflammation toward Fibrosis. Am J Pathol (2015) 185(4):1145–55. doi:10.1016/j.ajpath.2014.11.028
94. Sun C, Wu MH, Lee ES, Yuan SY. A Disintegrin and Metalloproteinase 15 Contributes to Atherosclerosis by Mediating Endothelial Barrier Dysfunction via Src Family Kinase Activity. Arterioscler Thromb Vasc Biol (2012) 32(10):2444–51. doi:10.1161/ATVBAHA.112.252205
95. Holdt LM, Thiery J, Breslow JL, Teupser D. Increased ADAM17 mRNA Expression and Activity Is Associated with Atherosclerosis Resistance in LDL-Receptor Deficient Mice. Arterioscler Thromb Vasc Biol (2008) 28(6):1097–103. doi:10.1161/ATVBAHA.108.165654
96. Nicolaou A, Zhao Z, Northoff BH, Sass K, Herbst A, Kohlmaier A, et al. Adam17 Deficiency Promotes Atherosclerosis by Enhanced TNFR2 Signaling in Mice. Arterioscler Thromb Vasc Biol (2017) 37(2):247–57. doi:10.1161/ATVBAHA.116.308682
97. Schlomann U, Rathke-Hartlieb S, Yamamoto S, Jockusch H, Bartsch JW. Tumor Necrosis Factor α Induces a Metalloprotease-Disintegrin, ADAM8 (CD 156): Implications for Neuron-Glia Interactions during Neurodegeneration. J Neurosci (2000) 20(21):7964–71. doi:10.1523/JNEUROSCI.20-21-07964.2000
98. van der Vorst EPC, Keijbeck AA, de Winther MPJ, Donners MMPC. A Disintegrin and Metalloproteases: Molecular Scissors in Angiogenesis, Inflammation and Atherosclerosis. Atherosclerosis (2012) 224(2):302–8. doi:10.1016/j.atherosclerosis.2012.04.023
99. Ainola M, Li T-F, Mandelin J, Hukkanen M, Choi SJ, Salo J, et al. Involvement of a Disintegrin and a Metalloproteinase 8 (ADAM8) in Osteoclastogenesis and Pathological Bone Destruction. Ann Rheum Dis (2009) 68(3):427–34. doi:10.1136/ard.2008.088260
100. Foley SC, Mogas AK, Olivenstein R, Fiset PO, Chakir J, Bourbeau J, et al. Increased Expression of ADAM33 and ADAM8 with Disease Progression in Asthma. J Allergy Clin Immunol (2007) 119(4):863–71. doi:10.1016/j.jaci.2006.12.665
101. Gossens K, Naus S, Holländer GA, Ziltener HJ. Deficiency of the Metalloproteinase-Disintegrin ADAM8 Is Associated with Thymic Hyper-Cellularity. PLoS ONE (2010) 5(9):e12766. doi:10.1371/journal.pone.0012766
102. Johansson MW, Lye MH, Barthel SR, Duffy AK, Annis DS, Mosher DF. Eosinophils Adhere to Vascular Cell Adhesion Molecule-1 via Podosomes. Am J Respir Cel Mol Biol (2004) 31(4):413–22. doi:10.1165/rcmb.2004-0099OC
103. Kelly K, Hutchinson G, Nebenius-Oosthuizen D, Smith AJH, Bartsch Jr. W, Horiuchi K, et al. Metalloprotease-disintegrin ADAM8: Expression Analysis and Targeted Deletion in Mice. Dev Dyn (2005) 232(1):221–31. doi:10.1002/dvdy.20221
104. Richens J, Fairclough L, Ghaemmaghami A, Mahdavi J, Shakib F, Sewell H. The Detection of ADAM8 Protein on Cells of the Human Immune System and the Demonstration of its Expression on Peripheral Blood B Cells, Dendritic Cells and Monocyte Subsets. Immunobiology (2007) 212(1):29–38. doi:10.1016/j.imbio.2006.06.012
105. Oreo KM, Gibson PG, Simpson JL, Wood LG, McDonald VM, Baines KJ. Sputum ADAM8 Expression Is Increased in Severe Asthma and COPD. Clin Exp Allergy (2014) 44(3):342–52. doi:10.1111/cea.12223
106. Valkovskaya N, Kayed H, Felix K, Hartmann D, Giese NA, Osinsky SP, et al. ADAM8 Expression Is Associated with Increased Invasiveness and Reduced Patient Survival in Pancreatic Cancer. J Cell. Mol Med (2007) 11(5):1162–74. doi:10.1111/j.1582-4934.2007.00082.x
107. Gifford V, Itoh Y. MT1-MMP-dependent Cell Migration: Proteolytic and Non-proteolytic Mechanisms. Biochem Soc Trans (2019) 47(3):811–26. doi:10.1042/BST20180363
108. Nagase H, Visse R, Murphy G. Structure and Function of Matrix Metalloproteinases and TIMPs. Cardiovasc Res (2006) 69(3):562–73. doi:10.1016/j.cardiores.2005.12.002
109. Itoh Y. Membrane-type Matrix Metalloproteinases: Their Functions and Regulations. Matrix Biol (2015) 44-46(46):207–23. doi:10.1016/j.matbio.2015.03.004
110. Pap T, Shigeyama Y, Kuchen S, Fernihough JK, Simmen B, Gay RE, et al. Differential Expression Pattern of Membrane‐type Matrix Metalloproteinases in Rheumatoid Arthritis. Arthritis Rheum (2000) 43(6):1226–32. doi:10.1002/1529-0131(200006)43:6<1226::AID-ANR5>3.0.CO;2-4
111. Ohkawara H, Ikeda K, Ogawa K, Takeishi Y. Membrane Type 1-matrix Metalloproteinase (Mt1-mmp) Identified as a Multifunctional Regulator of Vascular Responses. Fukushima J Med Sci (2015) 61(2):91–100. doi:10.5387/fms.2015-15
112. Miller M-C, Manning HB, Jain A, Troeberg L, Dudhia J, Essex D, et al. Membrane Type 1 Matrix Metalloproteinase Is a Crucial Promoter of Synovial Invasion in Human Rheumatoid Arthritis. Arthritis Rheum (2009) 60(3):686–97. doi:10.1002/art.24331
113. Seiki M. Membrane-type 1 Matrix Metalloproteinase: A Key Enzyme for Tumor Invasion. Cancer Lett (2003) 194(1):1–11. doi:10.1016/S0304-3835(02)00699-7
114. Fortunati D, Chau DYS, Wang Z, Collighan RJ, Griffin M. Cross-linking of Collagen I by Tissue Transglutaminase Provides a Promising Biomaterial for Promoting Bone Healing. Amino Acids (2014) 46(7):1751–61. doi:10.1007/s00726-014-1732-0
115. Mekhail M, Wong KKH, Padavan DT, Wu Y, O'Gorman DB, Wan W. Genipin-Cross-linked Electrospun Collagen Fibers. J Biomater Sci Polym Edition (2011) 22(17):2241–59. doi:10.1163/092050610X538209
116. Olde Damink LHH, Dijkstra PJ, Van Luyn MJA, Van Wachem PB, Nieuwenhuis P, Feijen J. Glutaraldehyde as a Crosslinking Agent for Collagen-Based Biomaterials. J Mater Sci Mater Med (1995) 6(8):460–72. doi:10.1007/BF00123371
117. Olde Damink LHH, Dijkstra PJ, van Luyn MJA, van Wachem PB, Nieuwenhuis P, Feijen J. Cross-linking of Dermal Sheep Collagen Using a Water-Soluble Carbodiimide. Biomaterials (1996) 17(8):765–73. doi:10.1016/0142-9612(96)81413-X
118. Smith-Mungo LI, Kagan HM. Lysyl Oxidase: Properties, Regulation and Multiple Functions in Biology. Matrix Biol (1998) 16(7):387–98. doi:10.1016/S0945-053X(98)90012-9
119. Mouw JK, Ou G, Weaver VM. Extracellular Matrix Assembly: A Multiscale Deconstruction. Nat Rev Mol Cel Biol (2014) 15(12):771–85. doi:10.1038/nrm3902
120. Bax DV, Davidenko N, Gullberg D, Hamaia SW, Farndale RW, Best SM, et al. Fundamental Insight into the Effect of Carbodiimide Crosslinking on Cellular Recognition of Collagen-Based Scaffolds. Acta Biomater (2017) 49:218–34. doi:10.1016/j.actbio.2016.11.059
121. Takigawa T, Endo Y. Effects of Glutaraldehyde Exposure on Human Health. J Occup Health (2006) 48(2):75–87. doi:10.1539/joh.48.75
122. Nair M, Best SM, Cameron RE. Crosslinking Collagen Constructs: Achieving Cellular Selectivity through Modifications of Physical and Chemical Properties. Appl Sci (2020) 10(19):6911. doi:10.3390/app10196911
123. Schmidt CE, Baier JM. Acellular Vascular Tissues: Natural Biomaterials for Tissue Repair and Tissue Engineering. Biomaterials (2000) 21(22):2215–31. doi:10.1016/S0142-9612(00)00148-4
124. Sheu M-T, Huang J-C, Yeh G-C, Ho H-O. Characterization of Collagen Gel Solutions and Collagen Matrices for Cell Culture. Biomaterials (2001) 22(13):1713–9. doi:10.1016/S0142-9612(00)00315-X
125. Chen D-C, Lai Y-L, Lee S-Y, Hung S-L, Chen H-L. Osteoblastic Response to Collagen Scaffolds Varied in Freezing Temperature and Glutaraldehyde Crosslinking. J Biomed Mater Res (2007) 80A(2):399–409. doi:10.1002/jbm.a.30932
126. Shepherd JH, Ghose S, Kew SJ, Moavenian A, Best SM, Cameron RE. Effect of Fiber Crosslinking on Collagen-Fiber Reinforced Collagen-Chondroitin-6-Sulfate Materials for Regenerating Load-Bearing Soft Tissues. J Biomed Mater Res (2013) 101A(1):176–84. doi:10.1002/jbm.a.34317
127. Davidenko N, Schuster CF, Bax DV, Raynal N, Farndale RW, Best SM, et al. Control of Crosslinking for Tailoring Collagen-Based Scaffolds Stability and Mechanics. Acta Biomater (2015) 25:131–42. doi:10.1016/j.actbio.2015.07.034
128. Nair M, Calahorra Y, Kar-Narayan S, Best SM, Cameron RE. Self-assembly of Collagen Bundles and Enhanced Piezoelectricity Induced by Chemical Crosslinking. Nanoscale (2019) 11(32):15120–30. doi:10.1039/C9NR04750F
129. Ahmad Z, Shepherd JH, Shepherd DV, Ghose S, Kew SJ, Cameron RE, et al. Effect of 1-Ethyl-3-(3-Dimethylaminopropyl) Carbodiimide and N-Hydroxysuccinimide Concentrations on the Mechanical and Biological Characteristics of Cross-Linked Collagen Fibres for Tendon Repair. Regenerative Biomater (2015) 2(2):77–85. doi:10.1093/rb/rbv005
130. Grover CN, Gwynne JH, Pugh N, Hamaia S, Farndale RW, Best SM, et al. Crosslinking and Composition Influence the Surface Properties, Mechanical Stiffness and Cell Reactivity of Collagen-Based Films. Acta Biomater (2012) 8(8):3080–90. doi:10.1016/j.actbio.2012.05.006
131. Haugh MG, Murphy CM, McKiernan RC, Altenbuchner C, O'Brien FJ. Crosslinking and Mechanical Properties Significantly Influence Cell Attachment, Proliferation, and Migration within Collagen Glycosaminoglycan Scaffolds. Tissue Eng A (2011) 17(9–10):1201–8. doi:10.1089/ten.tea.2010.0590
132. Shepherd DV, Shepherd JH, Ghose S, Kew SJ, Cameron RE, Best SM. The Process of EDC-NHS Cross-Linking of Reconstituted Collagen Fibres Increases Collagen Fibrillar Order and Alignment. APL Mater (2015) 3(1):014902. doi:10.1063/1.4900887
133. Madaghiele M, Calò E, Salvatore L, Bonfrate V, Pedone D, Frigione M, et al. Assessment of Collagen Crosslinking and Denaturation for the Design of Regenerative Scaffolds. J Biomed Mater Res (2016) 104(1):186–94. doi:10.1002/jbm.a.35554
134. Chen X, Zhou L, Xu H, Yamamoto M, Shinoda M, Kishimoto M, et al. Effect of the Application of a Dehydrothermal Treatment on the Structure and the Mechanical Properties of Collagen Film. Materials (2020) 13(2):377. doi:10.3390/ma13020377
135. Soller EC, Tzeranis DS, Miu K, So PTC, Yannas IV. Common Features of Optimal Collagen Scaffolds that Disrupt Wound Contraction and Enhance Regeneration Both in Peripheral Nerves and in Skin. Biomaterials (2012) 33(19):4783–91. doi:10.1016/j.biomaterials.2012.03.068
136. Kato Y, Nishikawa T, Kawakishi S. Formation of Protein-Bound 3,4-dihydroxyphenylalanine in Collagen Types I and IV Exposed to Ultraviolet Light. Photochem Photobiol (1995) 61(4):367–72. doi:10.1111/j.1751-1097.1995.tb08624.x
137. Sionkowska A. Modification of Collagen Films by Ultraviolet Irradiation. Polym Degrad Stab (2000) 68(2):147–51. doi:10.1016/S0141-3910(99)00176-7
138. Davidenko N, Bax DV, Schuster CF, Farndale RW, Hamaia SW, Best SM, et al. Optimisation of UV Irradiation as a Binding Site Conserving Method for Crosslinking Collagen-Based Scaffolds. J Mater Sci Mater Med (2016) 27(1):14. doi:10.1007/s10856-015-5627-8
139. Bax DV, Davidenko N, Hamaia SW, Farndale RW, Best SM, Cameron RE. Impact of UV- and Carbodiimide-Based Crosslinking on the Integrin-Binding Properties of Collagen-Based Materials. Acta Biomater (2019) 100:280–91. doi:10.1016/j.actbio.2019.09.046
140. Riedel S, Hietschold P, Krömmelbein C, Kunschmann T, Konieczny R, Knolle W, et al. Design of Biomimetic Collagen Matrices by Reagent-free Electron Beam Induced Crosslinking: Structure-Property Relationships and Cellular Response. Mater Des (2019) 168:107606. doi:10.1016/j.matdes.2019.107606
141. Weadock K, Olson RM, Silver FH. Evaluation of Collagen Crosslinking Techniques. Biomater Med Devices, Artif Organs (1983) 11(4):293–318. doi:10.3109/10731198309118815
142. Ruijgrok JM, de Wijn JR, Boon ME. Glutaraldehyde Crosslinking of Collagen: Effects of Time, Temperature, Concentration and Presoaking as Measured by Shrinkage Temperature. Clin Mater (1994) 17(1):23–7. doi:10.1016/0267-6605(94)90044-2
143. Di Y, Heath RJ. Collagen Stabilization and Modification Using a Polyepoxide, Triglycidyl Isocyanurate. Polym Degrad Stab (2009) 94(10):1684–92. doi:10.1016/j.polymdegradstab.2009.06.019
144. Reiser K, McCormick RJ, Rucker RB. Enzymatic and Nonenzymatic Cross‐linking of Collagen and Elastin. FASEB j. (1992) 6(7):2439–49. doi:10.1096/fasebj.6.7.1348714
145.E Reichmanis, CW Frank, and JH O’Donnell, editors. Irradiation of Polymeric Materials: Processes, Mechanisms, and Applications, Vol. 527. Washington, United States: American Chemical Society (1993). doi:10.1021/bk-1993-0527
146. Hennink WE, van Nostrum CF. Novel Crosslinking Methods to Design Hydrogels. Adv Drug Deliv Rev (2012) 64:223–36. doi:10.1016/j.addr.2012.09.009
147. Riedel S, Bela K, Wisotzki EI, Suckfüll C, Zajadacz J, Mayr SG. Reagent-free Mechanical Patterning of Gelatin Surfaces by Two-step Electron Irradiation Treatment. Mater Des (2018) 153:80–5. doi:10.1016/j.matdes.2018.04.076
148. Wisotzki EI, Friedrich RP, Weidt A, Alexiou C, Mayr SG, Zink M. Cellular Response to Reagent-free Electron-Irradiated Gelatin Hydrogels. Macromol Biosci (2016) 16(6):914–24. doi:10.1002/mabi.201500408
149. Monaco G, Cholas R, Salvatore L, Madaghiele M, Sannino A. Sterilization of Collagen Scaffolds Designed for Peripheral Nerve Regeneration: Effect on Microstructure, Degradation and Cellular Colonization. Mater Sci Eng C (2017) 71:335–44. doi:10.1016/j.msec.2016.10.030
150. Hara M. Various Cross-Linking Methods for Collagens: Merit and Demerit of Methods by Radiation. Jpn Assoc Regenerative Dentistry (2006). doi:10.11223/jarde.3.118
151. Vieira FF, Del Mastro NL. Comparison of γ-radiation and Electron Beam Irradiation Effects on Gelatin. Radiat Phys Chem (2002) 63(3–6):331–2. doi:10.1016/S0969-806X(01)00522-9
152. Riedel S, Heyart B, Apel KS, Mayr SG. Programing Stimuli-Responsiveness of Gelatin with Electron Beams: Basic Effects and Development of a Hydration-Controlled Biocompatible Demonstrator. Sci Rep (2017) 7(1):17436. doi:10.1038/s41598-017-17734-y
153. Manickam B, Sreedharan R, Elumalai M. ‘Genipin’ - the Natural Water Soluble Cross-Linking Agent and its Importance in the Modified Drug Delivery Systems: An Overview. Curr Drug Deliv (2014) 11(1):139–45. doi:10.2174/15672018113106660059
154. Butler MF, Ng Y-F, Pudney PDA. Mechanism and Kinetics of the Crosslinking Reaction between Biopolymers Containing Primary Amine Groups and Genipin. J Polym Sci A Polym Chem (2003) 41(24):3941–53. doi:10.1002/pola.10960
155. Tambe N, Di J, Zhang Z, Bernacki S, El-Shafei A, King MW. Novel Genipin-Collagen Immobilization of Polylactic Acid (PLA) Fibers for Use as Tissue Engineering Scaffolds. J Biomed Mater Res (2015) 103(6):1188–97. doi:10.1002/jbm.b.33285
156. Sundararaghavan HG, Monteiro GA, Lapin NA, Chabal YJ, Miksan JR, Shreiber DI. Genipin-induced Changes in Collagen Gels: Correlation of Mechanical Properties to Fluorescence. J Biomed Mater Res (2008) 87A(2):308–20. doi:10.1002/jbm.a.31715
157. Zhang X, Chen X, Yang T, Zhang N, Dong L, Ma S, et al. The Effects of Different Crossing-Linking Conditions of Genipin on Type I Collagen Scaffolds: An In Vitro Evaluation. Cell Tissue Bank (2014) 15(4):531–41. doi:10.1007/s10561-014-9423-3
158. Singh H, Racadio J, Brown M, Hedman T. Six-Month Safety and Efficacy of Genipin Crosslinking Treatment for Chronic Low Back Pain. Spine J (2017) 17(10):S206. doi:10.1016/j.spinee.2017.08.069
159. Nair M, Johal RK, Hamaia SW, Best SM, Cameron RE. Tunable Bioactivity and Mechanics of Collagen-Based Tissue Engineering Constructs: A Comparison of EDC-NHS, Genipin and TG2 Cross-linkers. Biomaterials (2020) 254:120109. doi:10.1016/j.biomaterials.2020.120109
160. Tirella A, Liberto T, Ahluwalia A. Riboflavin and Collagen: New Crosslinking Methods to Tailor the Stiffness of Hydrogels. Mater Lett (2012) 74:58–61. doi:10.1016/j.matlet.2012.01.036
161. Cheema U, Rong Z, Kirresh O, MacRobert AJ, Vadgama P, Brown RA. Oxygen Diffusion through Collagen Scaffolds at Defined Densities: Implications for Cell Survival in Tissue Models. J Tissue Eng Regen Med (2012) 6(1):77–84. doi:10.1002/term.402
162. Collin EC, Grad S, Zeugolis DI, Vinatier CS, Clouet JR, Guicheux JJ, et al. An Injectable Vehicle for Nucleus Pulposus Cell-Based Therapy. Biomaterials (2011) 32(11):2862–70. doi:10.1016/j.biomaterials.2011.01.018
163. Cosgriff-Hernandez E, Hahn MS, Russell B, Wilems T, Munoz-Pinto D, Browning MB, et al. Bioactive Hydrogels Based on Designer Collagens. Acta Biomater (2010) 6(10):3969–77. doi:10.1016/j.actbio.2010.05.002
164. Sarrigiannidis SO, Rey JM, Dobre O, González-García C, Dalby MJ, Salmeron-Sanchez M. A Tough Act to Follow: Collagen Hydrogel Modifications to Improve Mechanical and Growth Factor Loading Capabilities. Mater Today Bio (2021) 10:100098. doi:10.1016/j.mtbio.2021.100098
165. Taguchi T, Xu L, Kobayashi H, Taniguchi A, Kataoka K, Tanaka J. Encapsulation of Chondrocytes in Injectable Alkali-Treated Collagen Gels Prepared Using Poly(ethylene Glycol)-Based 4-armed star Polymer. Biomaterials (2005) 26(11):1247–52. doi:10.1016/j.biomaterials.2004.04.029
166. Ward J, Kelly J, Wang W, Zeugolis DI, Pandit A. Amine Functionalization of Collagen Matrices with Multifunctional Polyethylene Glycol Systems. Biomacromolecules (2010) 11(11):3093–101. doi:10.1021/bm100898p
167. Hayn A, Fischer T, Mierke CT. Inhomogeneities in 3D Collagen Matrices Impact Matrix Mechanics and Cancer Cell Migration. Front Cel Dev. Biol. (2020) 8:593879. doi:10.3389/fcell.2020.593879
168. Jansen KA, Licup AJ, Sharma A, Rens R, MacKintosh FC, Koenderink GH. The Role of Network Architecture in Collagen Mechanics. Biophysical J (2018) 114(11):2665–78. doi:10.1016/j.bpj.2018.04.043
169. Chau DYS, Collighan RJ, Verderio EAM, Addy VL, Griffin M. The Cellular Response to Transglutaminase-Cross-Linked Collagen. Biomaterials (2005) 26(33):6518–29. doi:10.1016/j.biomaterials.2005.04.017
170. Keillor JW, Clouthier CM, Apperley KYP, Akbar A, Mulani A. Acyl Transfer Mechanisms of Tissue Transglutaminase. Bioorg Chem (2014) 57:186–97. doi:10.1016/j.bioorg.2014.06.003
171. Mäki JM. Lysyl Oxidases in Mammalian Development and Certain Pathological Conditions. Histol Histopathol (2009) 24:651–60. doi:10.14670/HH-24.651
172. Trackman PC. Enzymatic and Non-enzymatic Functions of the Lysyl Oxidase Family in Bone. Matrix Biol (2016) 52-54(54):7–18. doi:10.1016/j.matbio.2016.01.001
173. Gelse K. Collagens-structure, Function, and Biosynthesis. Adv Drug Deliv Rev (2003) 55(12):1531–46. doi:10.1016/j.addr.2003.08.002
174. Ricard-Blum S. The Collagen Family. Cold Spring Harbor Perspect Biol (2011) 3(1):a004978. doi:10.1101/cshperspect.a004978
175. Söderhäll C, Marenholz I, Kerscher T, Rüschendorf F, Esparza-Gordillo J, Worm M, et al. Variants in a Novel Epidermal Collagen Gene (COL29A1) Are Associated with Atopic Dermatitis. Plos Biol (2007) 5(9):e242. doi:10.1371/journal.pbio.0050242
176. Lin J, Shi Y, Men Y, Wang X, Ye J, Zhang C. Mechanical Roles in Formation of Oriented Collagen Fibers. Tissue Eng B: Rev (2020) 26(2):116–28. doi:10.1089/ten.teb.2019.0243
177. Canty EG, Lu Y, Meadows RS, Shaw MK, Holmes DF, Kadler KE. Coalignment of Plasma Membrane Channels and Protrusions (Fibripositors) Specifies the Parallelism of Tendon. J Cel Biol (2004) 165(4):553–63. doi:10.1083/jcb.200312071
178. Kadler KE, Holmes DF, Trotter JA, Chapman JA. Collagen Fibril Formation. Biochem J (1996) 316(1):1–11. doi:10.1042/bj3160001
179. Noitup P, Morrissey MT, Garnjanagoonchorn W. In Vitro self-assembly of Silver-Line Grunt Type I Collagen: Effects of Collagen Concentrations, pH and Temperatures on Collagen Self-Assembly. J Food Biochem (2006) 30(5):547–55. doi:10.1111/j.1745-4514.2006.00081.x
180. Wood GC, Keech MK. The Formation of Fibrils from Collagen Solutions 1. The Effect of Experimental Conditions: Kinetic and Electron-Microscope Studies*. Biochem J (1960) 75(3):588–98. doi:10.1042/bj0750588
181. Yan M, Li B, Zhao X, Qin S. Effect of Concentration, pH and Ionic Strength on the Kinetic Self-Assembly of Acid-Soluble Collagen from Walleye pollock (Theragra chalcogramma) Skin. Food Hydrocolloids (2012) 29(1):199–204. doi:10.1016/j.foodhyd.2012.02.014
182. Cisneros DA, Friedrichs J, Taubenberger A, Franz CM, Muller DJ. Creating Ultrathin Nanoscopic Collagen Matrices for Biological and Biotechnological Applications. Small (2007) 3(6):956–63. doi:10.1002/smll.200600598
183. Hauschka SD, Konigsberg IR. The Influence of Collagen on the Development of Muscle Clones. Proc Natl Acad Sci (1966) 55(1):119–26. doi:10.1073/pnas.55.1.119
184. Kleinman HK, Luckenbill-Edds L, Cannon FW, Sephel GC. Use of Extracellular Matrix Components for Cell Culture. Anal Biochem (1987) 166(1):1–13. doi:10.1016/0003-2697(87)90538-0
185. Martin GR, Kleinman HK. Extracellular Matrix Proteins Give New Life to Cell Culture. Hepatology (1981) 1(3):264–6. doi:10.1002/hep.1840010312
186. Mogilner A, Oster G. Cell Motility Driven by Actin Polymerization. Biophysical J (1996) 71(6):3030–45. doi:10.1016/S0006-3495(96)79496-1
187. Vicker MG. F-actin Assembly in Dictyostelium Cell Locomotion and Shape Oscillations Propagates as a Self-Organized Reaction-Diffusion Wave. FEBS Lett (2002) 510(1–2):5–9. doi:10.1016/S0014-5793(01)03207-0
188. Vicker MG. Eukaryotic Cell Locomotion Depends on the Propagation of Self-Organized Reaction-Diffusion Waves and Oscillations of Actin Filament Assembly☆. Exp Cel Res (2002) 275(1):54–66. doi:10.1006/excr.2001.5466
189. Cowin SC. How Is a Tissue Built?1. J Biomechanical Eng (2000) 122(6):553–69. doi:10.1115/1.1324665
190. Cowin SC. Tissue Growth and Remodeling. Annu Rev Biomed Eng (2004) 6(1):77–107. doi:10.1146/annurev.bioeng.6.040803.140250
191. Silver FH, Freeman JW, Seehra GP. Collagen Self-Assembly and the Development of Tendon Mechanical Properties. J Biomech (2003) 36(10):1529–53. doi:10.1016/S0021-9290(03)00135-0
192. Amiel D, Ishizue KK, Harwood FL, Kitabayashi L, Akeson WH. Injury of the Anterior Cruciate Ligament: The Role of Collagenase in Ligament Degeneration. J Orthop Res (1989) 7(4):486–93. doi:10.1002/jor.1100070405
193. Elliott DH. Structure and Function of Mammalian Tendon. Biol Rev (1965) 40(3):392–421. doi:10.1111/j.1469-185X.1965.tb00808.x
194. Kjær M. Role of Extracellular Matrix in Adaptation of Tendon and Skeletal Muscle to Mechanical Loading. Physiol Rev (2004) 84(2):649–98. doi:10.1152/physrev.00031.2003
195. Mosesson MW, Siebenlist KR, Meh DA. The Structure and Biological Features of Fibrinogen and Fibrin. Ann N Y Acad Sci (2006) 936(1):11–30. doi:10.1111/j.1749-6632.2001.tb03491.x
196. Welch MP, Odland GF, Clark RA. Temporal Relationships of F-Actin Bundle Formation, Collagen and Fibronectin Matrix Assembly, and Fibronectin Receptor Expression to Wound Contraction. J Cel Biol (1990) 110(1):133–45. doi:10.1083/jcb.110.1.133
197. Leggett SE, Patel M, Valentin TM, Gamboa L, Khoo AS, Williams EK, et al. Mechanophenotyping of 3D Multicellular Clusters Using Displacement Arrays of Rendered Tractions. Proc Natl Acad Sci USA (2020) 117(11):5655–63. doi:10.1073/pnas.1918296117
198. Torbet J, Malbouyres M, Builles N, Justin V, Roulet M, Damour O, et al. Orthogonal Scaffold of Magnetically Aligned Collagen Lamellae for Corneal Stroma Reconstruction. Biomaterials (2007) 28(29):4268–76. doi:10.1016/j.biomaterials.2007.05.024
199. Yang S, Shi X, Li X, Wang J, Wang Y, Luo Y. Oriented Collagen Fiber Membranes Formed through Counter-rotating Extrusion and Their Application in Tendon Regeneration. Biomaterials (2019) 207:61–75. doi:10.1016/j.biomaterials.2019.03.041
200. Chen J, Gao K, Liu S, Wang S, Elango J, Bao B, et al. Fish Collagen Surgical Compress Repairing Characteristics on Wound Healing Process In Vivo. Mar Drugs (2019) 17(1):33. doi:10.3390/md17010033
201. McCoy MG, Wei JM, Choi S, Goerger JP, Zipfel W, Fischbach C. Collagen Fiber Orientation Regulates 3D Vascular Network Formation and Alignment. ACS Biomater Sci Eng (2018) 4(8):2967–76. doi:10.1021/acsbiomaterials.8b00384
202. Fratzl P, Misof K, Zizak I, Rapp G, Amenitsch H, Bernstorff S. Fibrillar Structure and Mechanical Properties of Collagen. J Struct Biol (1998) 122(1–2):119–22. doi:10.1006/jsbi.1998.3966
203. Lidén M, Movin T, Ejerhed L, Papadogiannakis N, Blomén E, Hultenby K, et al. A Histological and Ultrastructural Evaluation of the Patellar Tendon 10 Years after Reharvesting its Central Third. Am J Sports Med (2008) 36(4):781–8. doi:10.1177/0363546507311092
204. Screen HRC, Berk DE, Kadler KE, Ramirez F, Young MF. Tendon Functional Extracellular Matrix. J Orthop Res (2015) 33(6):793–9. doi:10.1002/jor.22818
205. Fang F, Lake SP. Experimental Evaluation of Multiscale Tendon Mechanics. J Orthop Res (2017) 35(7):1353–65. doi:10.1002/jor.23488
206. Lake SP, Miller KS, Elliott DM, Soslowsky LJ. Effect of Fiber Distribution and Realignment on the Nonlinear and Inhomogeneous Mechanical Properties of Human Supraspinatus Tendon under Longitudinal Tensile Loading. J Orthop Res (2009) 27(12):1596–602. doi:10.1002/jor.20938
207. Lake SP, Miller KS, Elliott DM, Soslowsky LJ. Tensile Properties and Fiber Alignment of Human Supraspinatus Tendon in the Transverse Direction Demonstrate Inhomogeneity, Nonlinearity, and Regional Isotropy. J Biomech (2010) 43(4):727–32. doi:10.1016/j.jbiomech.2009.10.017
208. Birk DE, Trelstad RL. Extracellular Compartments in Matrix Morphogenesis: Collagen Fibril, Bundle, and Lamellar Formation by Corneal Fibroblasts. J Cel Biol (1984) 99(6):2024–33. doi:10.1083/jcb.99.6.2024
209. Birk DE, Trelstad RL. Extracellular Compartments in Tendon Morphogenesis: Collagen Fibril, Bundle, and Macroaggregate Formation. J Cel Biol (1986) 103(1):231–40. doi:10.1083/jcb.103.1.231
210. Kalson NS, Starborg T, Lu Y, Mironov A, Humphries SM, Holmes DF, et al. Nonmuscle Myosin II Powered Transport of Newly Formed Collagen Fibrils at the Plasma Membrane. Proc Natl Acad Sci (2013) 110(49):E4743–E4752. doi:10.1073/pnas.1314348110
211. Paten JA, Siadat SM, Susilo ME, Ismail EN, Stoner JL, Rothstein JP, et al. Flow-Induced Crystallization of Collagen: A Potentially Critical Mechanism in Early Tissue Formation. ACS Nano (2016) 10(5):5027–40. doi:10.1021/acsnano.5b07756
212. Giraud-Guille MM, Mosser G, Belamie E. Liquid Crystallinity in Collagen Systems In Vitro and In Vivo. Curr Opin Colloid Interf Sci (2008) 13(4):303–13. doi:10.1016/j.cocis.2008.03.002
213. Griffith JW, Sokol CL, Luster AD. Chemokines and Chemokine Receptors: Positioning Cells for Host Defense and Immunity. Annu Rev Immunol (2014) 32(1):659–702. doi:10.1146/annurev-immunol-032713-120145
214. Schulz O, Hammerschmidt SI, Moschovakis GL, Förster R. Chemokines and Chemokine Receptors in Lymphoid Tissue Dynamics. Annu Rev Immunol (2016) 34(1):203–42. doi:10.1146/annurev-immunol-041015-055649
215. Kohli K, Pillarisetty VG, Kim TS. Key Chemokines Direct Migration of Immune Cells in Solid Tumors. Cancer Gene Ther (2021). doi:10.1038/s41417-021-00303-x
216. Barnes TA, Amir E. HYPE or HOPE: The Prognostic Value of Infiltrating Immune Cells in Cancer. Br J Cancer (2017) 117(4):451–60. doi:10.1038/bjc.2017.220
217. Burugu S, Asleh-Aburaya K, Nielsen TO. Immune Infiltrates in the Breast Cancer Microenvironment: Detection, Characterization and Clinical Implication. Breast Cancer (2017) 24(1):3–15. doi:10.1007/s12282-016-0698-z
218. Di Caro G, Marchesi F, Laghi L, Grizzi F. Immune Cells: Plastic Players along Colorectal Cancer Progression. J Cel Mol. Med. (2013) 17(9):1088–95. doi:10.1111/jcmm.12117
219. Ladányi A. Prognostic and Predictive Significance of Immune Cells Infiltrating Cutaneous Melanoma. Pigment Cel Melanoma Res (2015) 28(5):490–500. doi:10.1111/pcmr.12371
220. Roussos ET, Condeelis JS, Patsialou A. Chemotaxis in Cancer. Nat Rev Cancer (2011) 11(8):573–87. doi:10.1038/nrc3078
221. Oudin MJ, Weaver VM. Physical and Chemical Gradients in the Tumor Microenvironment Regulate Tumor Cell Invasion, Migration, and Metastasis. Cold Spring Harb Symp Quant Biol (2016) 81:189–205. doi:10.1101/sqb.2016.81.030817
222. De Buck M, Gouwy M, Berghmans N, Opdenakker G, Proost P, Struyf S, et al. COOH-terminal SAA1 Peptides Fail to Induce Chemokines but Synergize with CXCL8 and CCL3 to Recruit Leukocytes via FPR2. Blood (2017) 131:439–49. doi:10.1182/blood-2017-06-788554
223. Proudfoot AEI, Uguccioni M. Modulation of Chemokine Responses: Synergy and Cooperativity. Front Immunol (2016) 7. doi:10.3389/fimmu.2016.00183
224. Gouwy M, Schiraldi M, Struyf S, Van Damme J, Uguccioni M. Possible Mechanisms Involved in Chemokine Synergy fine Tuning the Inflammatory Response. Immunol Lett (2012) 145(1–2):10–4. doi:10.1016/j.imlet.2012.04.005
225. Lee G, Espirito Santo AI, Zwingenberger S, Cai L, Vogl T, Feldmann M, et al. Fully Reduced HMGB1 Accelerates the Regeneration of Multiple Tissues by Transitioning Stem Cells to GAlert. Proc Natl Acad Sci USA (2018) 115(19):E4463–E4472. doi:10.1073/pnas.1802893115
226. Tirone M, Tran NL, Ceriotti C, Gorzanelli A, Canepari M, Bottinelli R, et al. High Mobility Group Box 1 Orchestrates Tissue Regeneration via CXCR4. J Exp Med (2018) 215(1):303–18. doi:10.1084/jem.20160217
227. Venetz D, Ponzoni M, Schiraldi M, Ferreri AJM, Bertoni F, Doglioni C, et al. Perivascular Expression of CXCL9 and CXCL12 in Primary central Nervous System Lymphoma: T-Cell Infiltration and Positioning of Malignant B Cells. Int J Cancer (2010) 127(10):2300–12. doi:10.1002/ijc.25236
228. Cecchinato V, D’Agostino G, Raeli L, Uguccioni M. Chemokine Interaction with Synergy-Inducing Molecules: Fine Tuning Modulation of Cell Trafficking. J Leukoc Biol (2016) 99(6):851–5. doi:10.1189/jlb.1MR1015-457R
229. Schiraldi M, Raucci A, Muñoz LM, Livoti E, Celona B, Venereau E, et al. HMGB1 Promotes Recruitment of Inflammatory Cells to Damaged Tissues by Forming a Complex with CXCL12 and Signaling via CXCR4. J Exp Med (2012) 209(3):551–63. doi:10.1084/jem.20111739
230. D'Agostino G, Cecchinato V, Uguccioni M. Chemokine Heterocomplexes and Cancer: A Novel Chapter to Be Written in Tumor Immunity. Front Immunol (2018) 9:2185. doi:10.3389/fimmu.2018.02185
231. Mierke CT, Zitterbart DP, Kollmannsberger P, Raupach C, Schlötzer-Schrehardt U, Goecke TW, et al. Breakdown of the Endothelial Barrier Function in Tumor Cell Transmigration. Biophysical J (2008) 94(7):2832–46. doi:10.1529/biophysj.107.113613
232. Sarwar M, Evans JJ. Bioimprinting: Bringing Together 2D and 3D in Dissecting Cancer Biology. BioTechniques (2021). doi:10.2144/btn-2021-0058
233. Dhandayuthapani B, Yoshida Y, Maekawa T, Kumar DS. Polymeric Scaffolds in Tissue Engineering Application: A Review. Int J Polym Sci (2011) 2011:1–19. doi:10.1155/2011/290602
234. Huang G, Wang L, Wang S, Han Y, Wu J, Zhang Q, et al. Engineering Three-Dimensional Cell Mechanical Microenvironment with Hydrogels. Biofabrication (2012) 4(4):042001. doi:10.1088/1758-5082/4/4/042001
235. Gille J, Kunow J, Boisch L, Behrens P, Bos I, Hoffmann C, et al. Cell-Laden and Cell-free Matrix-Induced Chondrogenesis versus Microfracture for the Treatment of Articular Cartilage Defects. CARTILAGE (2010) 1(1):29–42. doi:10.1177/1947603509358721
236. Mierke CT, Frey B, Fellner M, Herrmann M, Fabry B. Integrin α5β1 Facilitates Cancer Cell Invasion through Enhanced Contractile Forces. J Cel Sci (2011) 124(3):369–83. doi:10.1242/jcs.071985
237. Patil VA, Masters KS. Engineered Collagen Matrices. Bioengineering (2020) 7(4):163. doi:10.3390/bioengineering7040163
238. Vinci M, Gowan S, Boxall F, Patterson L, Zimmermann M, Court W, et al. Advances in Establishment and Analysis of Three-Dimensional Tumor Spheroid-Based Functional Assays for Target Validation and Drug Evaluation. BMC Biol (2012) 10(1):29. doi:10.1186/1741-7007-10-29
239. Breslin S, O’Driscoll L. The Relevance of Using 3D Cell Cultures, in Addition to 2D Monolayer Cultures, when Evaluating Breast Cancer Drug Sensitivity and Resistance. Oncotarget (2016) 7(29):45745–56. doi:10.18632/oncotarget.9935
240. Mikhail AS, Eetezadi S, Allen C. Multicellular Tumor Spheroids for Evaluation of Cytotoxicity and Tumor Growth Inhibitory Effects of Nanomedicines In Vitro: A Comparison of Docetaxel-Loaded Block Copolymer Micelles and Taxotere. PLoS ONE (2013) 8(4):e62630. doi:10.1371/journal.pone.0062630
241. Carrow JK, Di Luca A, Dolatshahi-Pirouz A, Moroni L, Gaharwar AK. 3D-printed Bioactive Scaffolds from Nanosilicates and PEOT/PBT for Bone Tissue Engineering. Regenerative Biomater (2019) 6(1):29–37. doi:10.1093/rb/rby024
242. Grant R, Hay D, Callanan A. From Scaffold to Structure: The Synthetic Production of Cell Derived Extracellular Matrix for Liver Tissue Engineering. Biomed Phys Eng Express (2018) 4(6):065015. doi:10.1088/2057-1976/aacbe1
243. Hippler M, Lemma ED, Bertels S, Blasco E, Barner‐Kowollik C, Wegener M, et al. 3D Scaffolds to Study Basic Cell Biology. Adv Mater (2019) 31(26):1808110. doi:10.1002/adma.201808110
244. Langer EM, Allen-Petersen BL, King SM, Kendsersky ND, Turnidge MA, Kuziel GM, et al. Modeling Tumor Phenotypes In Vitro with Three-Dimensional Bioprinting. Cel Rep (2019) 26(3):608–23.e6. doi:10.1016/j.celrep.2018.12.090
245. Eguchi H, Akizuki R, Maruhashi R, Tsukimoto M, Furuta T, Matsunaga T, et al. Increase in Resistance to Anticancer Drugs Involves Occludin in Spheroid Culture Model of Lung Adenocarcinoma A549 Cells. Sci Rep (2018) 8(1):15157. doi:10.1038/s41598-018-33566-w
246. Gong X, Lin C, Cheng J, Su J, Zhao H, Liu T, et al. Generation of Multicellular Tumor Spheroids with Microwell-Based Agarose Scaffolds for Drug Testing. PLOS ONE (2015) 10(6):e0130348. doi:10.1371/journal.pone.0130348
247. Mehta G, Hsiao AY, Ingram M, Luker GD, Takayama S. Opportunities and Challenges for Use of Tumor Spheroids as Models to Test Drug Delivery and Efficacy. J Controlled Release (2012) 164(2):192–204. doi:10.1016/j.jconrel.2012.04.045
248. Ghosh S, Spagnoli GC, Martin I, Ploegert S, Demougin P, Heberer M, et al. Three-dimensional Culture of Melanoma Cells Profoundly Affects Gene Expression Profile: A High Density Oligonucleotide Array Study. J Cel Physiol. (2005) 204(2):522–31. doi:10.1002/jcp.20320
249. Zietarska M, Maugard CM, Filali-Mouhim A, Alam-Fahmy M, Tonin PN, Provencher DM, et al. Molecular Description of a 3D In Vitro Model for the Study of Epithelial Ovarian Cancer (EOC). Mol Carcinog (2007) 46(10):872–85. doi:10.1002/mc.20315
250. Belanger MH, Dolman L, Arcand SL, Shen Z, Chong G, Mes-Masson A-M, et al. A Targeted Analysis Identifies a High Frequency of BRCA1 and BRCA2 Mutation Carriers in Women with Ovarian Cancer from a Founder Population. J Ovarian Res (2015) 8(1):1. doi:10.1186/s13048-015-0124-8
251. Kapałczyńska M, Kolenda T, Przybyła W, Zajączkowska M, Teresiak A, Filas V, et al. 2D and 3D Cell Cultures - a Comparison of Different Types of Cancer Cell Cultures. Arch Med Sci (2016) 14:910. doi:10.5114/aoms.2016.63743
252. Karlsson H, Fryknäs M, Larsson R, Nygren P. Loss of Cancer Drug Activity in colon Cancer HCT-116 Cells during Spheroid Formation in a New 3-D Spheroid Cell Culture System. Exp Cel Res (2012) 318(13):1577–85. doi:10.1016/j.yexcr.2012.03.026
253. Sieh S, Taubenberger AV, Rizzi SC, Sadowski M, Lehman ML, Rockstroh A, et al. Phenotypic Characterization of Prostate Cancer LNCaP Cells Cultured within a Bioengineered Microenvironment. PLoS ONE (2012) 7(9):e40217. doi:10.1371/journal.pone.0040217
254. Hynes RO, Naba A. Overview of the Matrisome--An Inventory of Extracellular Matrix Constituents and Functions. Cold Spring Harbor Perspect Biol (2012) 4(1):a004903. doi:10.1101/cshperspect.a004903
255. Mecham RP. Overview of Extracellular Matrix. Curr Protoc Cel Biol (2012) 57(1). doi:10.1002/0471143030.cb1001s57
256.A Varki, RD Cummings, JD Esko, P Stanley, GW Hart, M Aebiet al. editors. Essentials of Glycobiology. 3rd ed. Cold Spring Harbor, New York: Cold Spring Harbor Laboratory Press (2015). Available from: http://www.ncbi.nlm.nih.gov/books/NBK310274/ (Accessed July 20, 2021).
257. Villa-Diaz LG, Kim JK, Laperle A, Palecek SP, Krebsbach PH. Inhibition of Focal Adhesion Kinase Signaling by Integrin α6β1 Supports Human Pluripotent Stem Cell Self-Renewal. Stem Cells (2016) 34(7):1753–64. doi:10.1002/stem.2349
258. Cattavarayane S, Palovuori R, Tanjore Ramanathan J, Manninen A. α6β1- and αV-integrins Are Required for Long-Term Self-Renewal of Murine Embryonic Stem Cells in the Absence of LIF. BMC Cel Biol (2015) 16(1):3. doi:10.1186/s12860-015-0051-y
259. Popov C, Radic T, Haasters F, Prall WC, Aszodi A, Gullberg D, et al. Integrins α2β1 and α11β1 Regulate the Survival of Mesenchymal Stem Cells on Collagen I. Cel Death Dis (2011) 2(7):e186. doi:10.1038/cddis.2011.71
260. Plaks V, Kong N, Werb Z. The Cancer Stem Cell Niche: How Essential Is the Niche in Regulating Stemness of Tumor Cells?. Cell Stem Cell (2015) 16(3):225–38. doi:10.1016/j.stem.2015.02.015
261. Fischer T, Hayn A, Mierke CT. Fast and Reliable Advanced Two-step Pore-Size Analysis of Biomimetic 3D Extracellular Matrix Scaffolds. Sci Rep (2019) 9(1):8352. doi:10.1038/s41598-019-44764-5
262. Park S, Lim S, Siriviriyakul P, Jeon JS. Three-dimensional Pore Network Characterization of Reconstructed Extracellular Matrix. Phys Rev E (2020) 101(5):052414. doi:10.1103/PhysRevE.101.052414
263. Shimshoni E, Adir I, Afik R, Solomonov I, Shenoy A, Adler M, et al. Distinct Extracellular-Matrix Remodeling Events Precede Symptoms of Inflammation. Matrix Biol (2019) 96:47. doi:10.1016/j.matbio.2020.11.001
264. Levental KR, Yu H, Kass L, Lakins JN, Egeblad M, Erler JT, et al. Matrix Crosslinking Forces Tumor Progression by Enhancing Integrin Signaling. Cell (2009) 139(5):891–906. doi:10.1016/j.cell.2009.10.027
265. Fernández-Sánchez ME, Barbier S, Whitehead J, Béalle G, Michel A, Latorre-Ossa H, et al. Mechanical Induction of the Tumorigenic β-catenin Pathway by Tumour Growth Pressure. Nature (2015) 523(7558):92–5. doi:10.1038/nature14329
266. Nia HT, Liu H, Seano G, Datta M, Jones D, Rahbari N, et al. Solid Stress and Elastic Energy as Measures of Tumour Mechanopathology. Nat Biomed Eng (2017) 1(1):0004. doi:10.1038/s41551-016-0004
267. Alessandri K, Sarangi BR, Gurchenkov VV, Sinha B, Kiessling TR, Fetler L, et al. Cellular Capsules as a Tool for Multicellular Spheroid Production and for Investigating the Mechanics of Tumor Progression In Vitro. Proc Natl Acad Sci (2013) 110(37):14843–8. doi:10.1073/pnas.1309482110
268. Helmlinger G, Netti PA, Lichtenbeld HC, Melder RJ, Jain RK. Solid Stress Inhibits the Growth of Multicellular Tumor Spheroids. Nat Biotechnol (1997) 15(8):778–83. doi:10.1038/nbt0897-778
269. Taubenberger AV, Girardo S, Träber N, Fischer‐Friedrich E, Kräter M, Wagner K, et al. 3D Microenvironment Stiffness Regulates Tumor Spheroid Growth and Mechanics via P21 and ROCK. Adv Biosys (2019) 3(9):1900128. doi:10.1002/adbi.201900128
270. Dolega ME, Delarue M, Ingremeau F, Prost J, Delon A, Cappello G. Cell-like Pressure Sensors Reveal Increase of Mechanical Stress towards the Core of Multicellular Spheroids under Compression. Nat Commun (2017) 8(1):14056. doi:10.1038/ncomms14056
271. Montel F, Delarue M, Elgeti J, Malaquin L, Basan M, Risler T, et al. Stress Clamp Experiments on Multicellular Tumor Spheroids. Phys Rev Lett (2011) 107(18):188102. doi:10.1103/PhysRevLett.107.188102
272. Delarue M, Montel F, Vignjevic D, Prost J, Joanny J-F, Cappello G. Compressive Stress Inhibits Proliferation in Tumor Spheroids through a Volume Limitation. Biophysical J (2014) 107(8):1821–8. doi:10.1016/j.bpj.2014.08.031
273. Dolega ME, Monnier S, Brunel B, Joanny J-F, Recho P, Cappello G. Extracellular Matrix in Multicellular Aggregates Acts as a Pressure Sensor Controlling Cell Proliferation and Motility. ELife (2021) 10:e63258. doi:10.7554/eLife.63258
274. Dolega M, Zurlo G, Goff ML, Greda M, Verdier C, Joanny J-F, et al. Mechanical Behavior of Multi-Cellular Spheroids under Osmotic Compression. J Mech Phys Sol (2021) 147:104205. doi:10.1016/j.jmps.2020.104205
275. Han YL, Pegoraro AF, Li H, Li K, Yuan Y, Xu G, et al. Cell Swelling, Softening and Invasion in a Three-Dimensional Breast Cancer Model. Nat Phys (2020) 16(1):101–8. doi:10.1038/s41567-019-0680-8
276. Jansen KA, Atherton P, Ballestrem C. Mechanotransduction at the Cell-Matrix Interface. Semin Cel Develop Biol (2017) 71:75–83. doi:10.1016/j.semcdb.2017.07.027
277. Jaalouk DE, Lammerding J. Mechanotransduction Gone Awry. Nat Rev Mol Cel Biol (2009) 10(1):63–73. doi:10.1038/nrm2597
278. Lu P, Weaver VM, Werb Z. The Extracellular Matrix: A Dynamic Niche in Cancer Progression. J Cel Biol (2012) 196(4):395–406. doi:10.1083/jcb.201102147
279. Snedeker JG, Gautieri A. The Role of Collagen Crosslinks in Ageing and Diabetes - the Good, the Bad, and the Ugly. Muscles Ligaments Tendons J (2014) 4(3):303–8. doi:10.11138/mltj/2014.4.3.303
280. Cho S, Irianto J, Discher DE. Mechanosensing by the Nucleus: From Pathways to Scaling Relationships. J Cel Biol (2017) 216(2):305–15. doi:10.1083/jcb.201610042
281. Moroni M, Servin-Vences MR, Fleischer R, Sánchez-Carranza O, Lewin GR. Voltage Gating of Mechanosensitive PIEZO Channels. Nat Commun (2018) 9(1):1096. doi:10.1038/s41467-018-03502-7
282. Rocio Servin-Vences M, Moroni M, Lewin GR, Poole K. Direct Measurement of TRPV4 and PIEZO1 Activity Reveals Multiple Mechanotransduction Pathways in Chondrocytes. ELife (2017) 6:e21074. doi:10.7554/eLife.21074
283. Pontani L-L, Jorjadze I, Viasnoff V, Brujic J. Biomimetic Emulsions Reveal the Effect of Mechanical Forces on Cell-Cell Adhesion. Proc Natl Acad Sci (2012) 109(25):9839–44. doi:10.1073/pnas.1201499109
284. Engler AJ, Sen S, Sweeney HL, Discher DE. Matrix Elasticity Directs Stem Cell Lineage Specification. Cell (2006) 126(4):677–89. doi:10.1016/j.cell.2006.06.044
285. Engler AJ, Humbert PO, Wehrle-Haller B, Weaver VM. Multiscale Modeling of Form and Function. Science (2009) 324(5924):208–12. doi:10.1126/science.1170107
286. Chaudhuri O, Gu L, Klumpers D, Darnell M, Bencherif SA, Weaver JC, et al. Hydrogels with Tunable Stress Relaxation Regulate Stem Cell Fate and Activity. Nat Mater (2016) 15(3):326–34. doi:10.1038/nmat4489
287. Chaudhuri O, Gu L, Darnell M, Klumpers D, Bencherif SA, Weaver JC, et al. Substrate Stress Relaxation Regulates Cell Spreading. Nat Commun (2015) 6(1):6365. doi:10.1038/ncomms7365
288. Mohammadi H, McCulloch CA. Impact of Elastic and Inelastic Substrate Behaviors on Mechanosensation. Soft Matter (2014) 10(3):408–20. doi:10.1039/C3SM52729H
289. Storm C, Pastore JJ, MacKintosh FC, Lubensky TC, Janmey PA. Nonlinear Elasticity in Biological Gels. Nature (2005) 435(7039):191–4. doi:10.1038/nature03521
290. Jansen KA, Bacabac RG, Piechocka IK, Koenderink GH. Cells Actively Stiffen Fibrin Networks by Generating Contractile Stress. Biophysical J (2013) 105(10):2240–51. doi:10.1016/j.bpj.2013.10.008
291. Hall MS, Alisafaei F, Ban E, Feng X, Hui C-Y, Shenoy VB, et al. Fibrous Nonlinear Elasticity Enables Positive Mechanical Feedback between Cells and ECMs. Proc Natl Acad Sci USA (2016) 113(49):14043–8. doi:10.1073/pnas.1613058113
292. Ma X, Schickel ME, Stevenson MD, Sarang-Sieminski AL, Gooch KJ, Ghadiali SN, et al. Fibers in the Extracellular Matrix Enable Long-Range Stress Transmission between Cells. Biophysical J (2013) 104(7):1410–8. doi:10.1016/j.bpj.2013.02.017
293. Rudnicki MS, Cirka HA, Aghvami M, Sander EA, Wen Q, Billiar KL. Nonlinear Strain Stiffening Is Not Sufficient to Explain How Far Cells Can Feel on Fibrous Protein Gels. Biophysical J (2013) 105(1):11–20. doi:10.1016/j.bpj.2013.05.032
294. Reinhart-King CA, Dembo M, Hammer DA. Cell-Cell Mechanical Communication through Compliant Substrates. Biophysical J (2008) 95(12):6044–51. doi:10.1529/biophysj.107.127662
295. Sapir L, Tzlil S. Talking over the Extracellular Matrix: How Do Cells Communicate Mechanically? Semin Cel Develop Biol (2017) 71:99–105. doi:10.1016/j.semcdb.2017.06.010
296. Hanahan D, Weinberg RA. The Hallmarks of Cancer. Cell (2000) 100(1):57–70. doi:10.1016/S0092-8674(00)81683-9
297. Hanahan D, Weinberg RA. Hallmarks of Cancer: The Next Generation. Cell (2011) 144(5):646–74. doi:10.1016/j.cell.2011.02.013
298. Lazebnik Y. What Are the Hallmarks of Cancer?. Nat Rev Cancer (2010) 10(4):232–3. doi:10.1038/nrc2827
299. Wilbur M, Shih I-M, Segars J, Fader A. Cancer Implications for Patients with Endometriosis. Semin Reprod Med (2017) 35(01):110–6. doi:10.1055/s-0036-1597120
300. Horne SD, Pollick SA, Heng HHQ. Evolutionary Mechanism Unifies the Hallmarks of Cancer. Int J Cancer (2015) 136(9):2012–21. doi:10.1002/ijc.29031
301. Bizzarri M, Cucina A, Conti F, D’Anselmi F. Beyond the Oncogene Paradigm: Understanding Complexity in Cancerogenesis. Acta Biotheor (2008) 56(3):173–96. doi:10.1007/s10441-008-9047-8
302. Sonnenschein C, Soto AM, Rangarajan A, Kulkarni P. Competing Views on Cancer. J Biosci (2014) 39(2):281–302. doi:10.1007/s12038-013-9403-y
303. Sonnenschein C, Soto AM. The Aging of the 2000 and 2011 Hallmarks of Cancer Reviews: A Critique. J Biosci (2013) 38(3):651–63. doi:10.1007/s12038-013-9335-6
304. Busque L, Patel JP, Figueroa ME, Vasanthakumar A, Provost S, Hamilou Z, et al. Recurrent Somatic TET2 Mutations in normal Elderly Individuals with Clonal Hematopoiesis. Nat Genet (2012) 44(11):1179–81. doi:10.1038/ng.2413
305. Martincorena I, Roshan A, Gerstung M, Ellis P, Van Loo P, McLaren S, et al. High burden and Pervasive Positive Selection of Somatic Mutations in normal Human Skin. Science (2015) 348(6237):880–6. doi:10.1126/science.aaa6806
306. Xie M, Lu C, Wang J, McLellan MD, Johnson KJ, Wendl MC, et al. Age-related Mutations Associated with Clonal Hematopoietic Expansion and Malignancies. Nat Med (2014) 20(12):1472–8. doi:10.1038/nm.3733
307. Kode A, Manavalan JS, Mosialou I, Bhagat G, Rathinam CV, Luo N, et al. Leukaemogenesis Induced by an Activating β-catenin Mutation in Osteoblasts. Nature (2014) 506(7487):240–4. doi:10.1038/nature12883
308. Raaijmakers MHGP. Myelodysplastic Syndromes: Revisiting the Role of the Bone Marrow Microenvironment in Disease Pathogenesis. Int J Hematol (2012) 95(1):17–25. doi:10.1007/s12185-011-1001-x
309. Carmeliet P, Jain RK. Angiogenesis in Cancer and Other Diseases. Nature (2000) 407(6801):249–57. doi:10.1038/35025220
310. Mayers JR, Torrence ME, Danai LV, Papagiannakopoulos T, Davidson SM, Bauer MR, et al. Tissue of Origin Dictates Branched-Chain Amino Acid Metabolism in Mutant Kras-Driven Cancers. Science (2016) 353(6304):1161–5. doi:10.1126/science.aaf5171
311. Ladoux B, Mège R-M. Mechanobiology of Collective Cell Behaviours. Nat Rev Mol Cel Biol (2017) 18(12):743–57. doi:10.1038/nrm.2017.98
312. Ladoux B, Mège R-M, Trepat X. Front-Rear Polarization by Mechanical Cues: From Single Cells to Tissues. Trends Cel Biol (2016) 26(6):420–33. doi:10.1016/j.tcb.2016.02.002
313. Parsons JT, Horwitz AR, Schwartz MA. Cell Adhesion: Integrating Cytoskeletal Dynamics and Cellular Tension. Nat Rev Mol Cel Biol (2010) 11(9):633–43. doi:10.1038/nrm2957
314. Bödeker HU, Beta C, Frank TD, Bodenschatz E. Quantitative Analysis of Random Ameboid Motion. EPL (Europhysics Letters) (2010) 90(2):28005. doi:10.1209/0295-5075/90/28005
315. Brückner DB, Fink A, Schreiber C, Röttgermann PJF, Rädler JO, Broedersz CP. Stochastic Nonlinear Dynamics of Confined Cell Migration in Two-State Systems. Nat Phys (2019) 15(6):595–601. doi:10.1038/s41567-019-0445-4
316. Selmeczi D, Mosler S, Hagedorn PH, Larsen NB, Flyvbjerg H. Cell Motility as Persistent Random Motion: Theories from Experiments. Biophysical J (2005) 89(2):912–31. doi:10.1529/biophysj.105.061150
317. Bosgraaf L, Van Haastert PJM. The Ordered Extension of Pseudopodia by Amoeboid Cells in the Absence of External Cues. PLoS ONE (2009) 4(4):e5253. doi:10.1371/journal.pone.0005253
318. Callan-Jones AC, Voituriez R. Actin Flows in Cell Migration: From Locomotion and Polarity to Trajectories. Curr Opin Cel Biol (2016) 38:12–7. doi:10.1016/j.ceb.2016.01.003
319. Maiuri P, Rupprecht J-F, Wieser S, Ruprecht V, Bénichou O, Carpi N, et al. Actin Flows Mediate a Universal Coupling between Cell Speed and Cell Persistence. Cell (2015) 161(2):374–86. doi:10.1016/j.cell.2015.01.056
320. Bagorda A, Parent CA. Eukaryotic Chemotaxis at a Glance. J Cel Sci (2008) 121(16):2621–4. doi:10.1242/jcs.018077
321. Metzner C, Mark C, Steinwachs J, Lautscham L, Stadler F, Fabry B. Superstatistical Analysis and Modelling of Heterogeneous Random Walks. Nat Commun (2015) 6(1):7516. doi:10.1038/ncomms8516
322. Nakajima A, Ishihara S, Imoto D, Sawai S. Rectified Directional Sensing in Long-Range Cell Migration. Nat Commun (2014) 5(1):5367. doi:10.1038/ncomms6367
323. Brückner DB, Arlt N, Fink A, Ronceray P, Rädler JO, Broedersz CP. Learning the Dynamics of Cell-Cell Interactions in Confined Cell Migration. Proc Natl Acad Sci USA (2021) 118(7):e2016602118. doi:10.1073/pnas.2016602118
324. Dumortier JG, Martin S, Meyer D, Rosa FM, David NB. Collective Mesendoderm Migration Relies on an Intrinsic Directionality Signal Transmitted through Cell Contacts. Proc Natl Acad Sci (2012) 109(42):16945–50. doi:10.1073/pnas.1205870109
325. Gupta M, Sarangi BR, Deschamps J, Nematbakhsh Y, Callan-Jones A, Margadant F, et al. Adaptive Rheology and Ordering of Cell Cytoskeleton Govern Matrix Rigidity Sensing. Nat Commun (2015) 6(1):7525. doi:10.1038/ncomms8525
326. Liu Y-J, Berre ML, Lautenschlaeger F, Maiuri P, Callan-Jones A, Heuzé M, et al. Confinement and Low Adhesion Induce Fast Amoeboid Migration of Slow Mesenchymal Cells. Cell (2015) 160(4):659–72. doi:10.1016/j.cell.2015.01.007
327. Petrie RJ, Yamada KM. Multiple Mechanisms of 3D Migration: The Origins of Plasticity. Curr Opin Cel Biol (2016) 42:7–12. doi:10.1016/j.ceb.2016.03.025
328. Petrie RJ, Gavara N, Chadwick RS, Yamada KM. Nonpolarized Signaling Reveals Two Distinct Modes of 3D Cell Migration. J Cel Biol (2012) 197(3):439–55. doi:10.1083/jcb.201201124
329. Yamada KM, Collins JW, Cruz Walma DA, Doyle AD, Morales SG, Lu J, et al. Extracellular Matrix Dynamics in Cell Migration, Invasion and Tissue Morphogenesis. Int J Exp Path (2019) 100(3):144–52. doi:10.1111/iep.12329
330. d’Alessandro J, Barbier--Chebbah A, Cellerin V, Benichou O, Mège RM, Voituriez R, et al. Cell Migration Guided by Long-Lived Spatial Memory. Nat Commun (2021) 12(1):4118. doi:10.1038/s41467-021-24249-8
331. Caballero D, Voituriez R, Riveline D. Protrusion Fluctuations Direct Cell Motion. Biophysical J (2014) 107(1):34–42. doi:10.1016/j.bpj.2014.05.002
332. Jain S, Cachoux VML, Narayana GHNS, de Beco S, D’Alessandro J, Cellerin V, et al. The Role of Single-Cell Mechanical Behaviour and Polarity in Driving Collective Cell Migration. Nat Phys (2020) 16(7):802–9. doi:10.1038/s41567-020-0875-z
333. Mohammed D, Charras G, Vercruysse E, Versaevel M, Lantoine J, Alaimo L, et al. Substrate Area Confinement Is a Key Determinant of Cell Velocity in Collective Migration. Nat Phys (2019) 15(8):858–66. doi:10.1038/s41567-019-0543-3
334. Hennig K, Wang I, Moreau P, Valon L, DeBeco S, Coppey M, et al. Stick-slip Dynamics of Cell Adhesion Triggers Spontaneous Symmetry Breaking and Directional Migration of Mesenchymal Cells on One-Dimensional Lines. Sci Adv (2020) 6(1):eaau5670. doi:10.1126/sciadv.aau5670
335. Lavi I, Piel M, Lennon-Duménil A-M, Voituriez R, Gov NS. Deterministic Patterns in Cell Motility. Nat Phys (2016) 12(12):1146–52. doi:10.1038/nphys3836
336. Eriksson JE, Dechat T, Grin B, Helfand B, Mendez M, Pallari H-M, et al. Introducing Intermediate Filaments: From Discovery to Disease. J Clin Invest (2009) 119(7):1763–71. doi:10.1172/JCI38339
337. Marusyk A, Janiszewska M, Polyak K. Intratumor Heterogeneity: The Rosetta Stone of Therapy Resistance. Cancer Cell (2020) 37(4):471–84. doi:10.1016/j.ccell.2020.03.007
338. Skylaki S, Hilsenbeck O, Schroeder T. Challenges in Long-Term Imaging and Quantification of Single-Cell Dynamics. Nat Biotechnol (2016) 34(11):1137–44. doi:10.1038/nbt.3713
339. Fletcher DA, Mullins RD. Cell Mechanics and the Cytoskeleton. Nature (2010) 463(7280):485–92. doi:10.1038/nature08908
340. Alibert C, Goud B, Manneville J-B. Are Cancer Cells Really Softer Than normal Cells?. Biol Cel (2017) 109(5):167–89. doi:10.1111/boc.201600078
341. Mierke CT. Mechanical Cues Affect Migration and Invasion of Cells from Three Different Directions. Front Cel Dev. Biol. (2020) 8:583226. doi:10.3389/fcell.2020.583226
342. Polacheck WJ, Chen CS. Measuring Cell-Generated Forces: A Guide to the Available Tools. Nat Methods (2016) 13(5):415–23. doi:10.1038/nmeth.3834
343. Trepat X, Wasserman MR, Angelini TE, Millet E, Weitz DA, Butler JP, et al. Physical Forces during Collective Cell Migration. Nat Phys (2009) 5(6):426–30. doi:10.1038/nphys1269
344. Kozminsky M, Sohn LL. The Promise of Single-Cell Mechanophenotyping for Clinical Applications. Biomicrofluidics (2020) 14(3):031301. doi:10.1063/5.0010800
345. Lamouille S, Xu J, Derynck R. Molecular Mechanisms of Epithelial-Mesenchymal Transition. Nat Rev Mol Cel Biol (2014) 15(3):178–96. doi:10.1038/nrm3758
346. Nieto MA, Huang RY-J, Jackson RA, Thiery JP. EMT: 2016. Cell (2016) 166(1):21–45. doi:10.1016/j.cell.2016.06.028
347. Zaravinos A. The Regulatory Role of MicroRNAs in EMT and Cancer. J Oncol (2015) 2015:1–13. doi:10.1155/2015/865816
348. Kariya Y, Oyama M, Suzuki T, Kariya Y. αvβ3 Integrin Induces Partial EMT Independent of TGF-β Signaling. Commun Biol (2021) 4(1):490. doi:10.1038/s42003-021-02003-6
349. Pal A, Barrett TF, Paolini R, Parikh A, Puram SV. Partial EMT in Head and Neck Cancer Biology: A Spectrum Instead of a Switch. Oncogene (2021) 40:5049–65. doi:10.1038/s41388-021-01868-5
350. Saitoh M. Involvement of Partial EMT in Cancer Progression. J Biochem (2018) 164(4):257–64. doi:10.1093/jb/mvy047
351. Saxena N, Mogha P, Dash S, Majumder A, Jadhav S, Sen S. Matrix Elasticity Regulates Mesenchymal Stem Cell Chemotaxis. J Cel Sci (2018) 131:jcs211391. doi:10.1242/jcs.211391
352. Yang J, Antin P, Antin P, Berx G, Blanpain C, Brabletz T, et al. Guidelines and Definitions for Research on Epithelial-Mesenchymal Transition. Nat Rev Mol Cel Biol (2020) 21(6):341–52. doi:10.1038/s41580-020-0237-9
353. Shah MK, Garcia-Pak IH, Darling EM. Influence of Inherent Mechanophenotype on Competitive Cellular Adherence. Ann Biomed Eng (2017) 45(8):2036–47. doi:10.1007/s10439-017-1841-5
354. Di Carlo D. A Mechanical Biomarker of Cell State in Medicine. J Lab Autom (2012) 17(1):32–42. doi:10.1177/2211068211431630
355. Gilchrist CL, Darling EM, Chen J, Setton LA. Extracellular Matrix Ligand and Stiffness Modulate Immature Nucleus Pulposus Cell-Cell Interactions. PLoS ONE (2011) 6(11):e27170. doi:10.1371/journal.pone.0027170
356. Poh Y-C, Chen J, Hong Y, Yi H, Zhang S, Chen J, et al. Generation of Organized Germ Layers from a Single Mouse Embryonic Stem Cell. Nat Commun (2014) 5(1):4000. doi:10.1038/ncomms5000
357. Jagielska A, Norman AL, Whyte G, Vliet KJV, Guck J, Franklin RJM. Mechanical Environment Modulates Biological Properties of Oligodendrocyte Progenitor Cells. Stem Cell Develop (2012) 21(16):2905–14. doi:10.1089/scd.2012.0189
358. Sen S, Dong M, Kumar S. Isoform-Specific Contributions of α-Actinin to Glioma Cell Mechanobiology. PLoS ONE (2009) 4(12):e8427. doi:10.1371/journal.pone.0008427
359. Sen S, Ng WP, Kumar S. Contributions of Talin-1 to Glioma Cell-Matrix Tensional Homeostasis. J R Soc Interf (2012) 9(71):1311–7. doi:10.1098/rsif.2011.0567
360. Solon J, Levental I, Sengupta K, Georges PC, Janmey PA. Fibroblast Adaptation and Stiffness Matching to Soft Elastic Substrates. Biophysical J (2007) 93(12):4453–61. doi:10.1529/biophysj.106.101386
361. Blumlein A, Williams N, McManus JJ. The Mechanical Properties of Individual Cell Spheroids. Sci Rep (2017) 7(1):7346. doi:10.1038/s41598-017-07813-5
362. Zimberlin JA, McManus JJ, Crosby AJ. Cavitation Rheology of the Vitreous: Mechanical Properties of Biological Tissue. Soft Matter (2010) 6(15):3632. doi:10.1039/b925407b
363. Zimberlin JA, Sanabria-DeLong N, Tew GN, Crosby AJ. Cavitation Rheology for Soft Materials. Soft Matter (2007) 3(6):763. doi:10.1039/b617050a
364. Mok S, Al Habyan S, Ledoux C, Lee W, MacDonald KN, McCaffrey L, et al. Mapping Cellular-Scale Internal Mechanics in 3D Tissues with Thermally Responsive Hydrogel Probes. Nat Commun (2020) 11(1):4757. doi:10.1038/s41467-020-18469-7
365. Tse JR, Engler AJ. Preparation of Hydrogel Substrates with Tunable Mechanical Properties. Curr Protoc Cel Biol (2010) 47(1). doi:10.1002/0471143030.cb1016s47
366. Morais JM, Papadimitrakopoulos F, Burgess DJ. Biomaterials/Tissue Interactions: Possible Solutions to Overcome Foreign Body Response. Aaps J (2010) 12(2):188–96. doi:10.1208/s12248-010-9175-3
367. Pei Y, Chen J, Yang L, Shi L, Tao Q, Hui B, et al. The Effect of pH on the LCST of poly(N-Isopropylacrylamide) and poly(N-Isopropylacrylamide-Co-Acrylic Acid). J Biomater Sci Polym Edition (2004) 15(5):585–94. doi:10.1163/156856204323046852
368. Sunyer R, Conte V, Escribano J, Elosegui-Artola A, Labernadie A, Valon L, et al. Collective Cell Durotaxis Emerges from Long-Range Intercellular Force Transmission. Science (2016) 353(6304):1157–61. doi:10.1126/science.aaf7119
369. Mierke CT. Phagocytized Beads Reduce the α5β1 Integrin Facilitated Invasiveness of Cancer Cells by Regulating Cellular Stiffness. Cell Biochem Biophys (2013) 66(3):599–622. doi:10.1007/s12013-012-9506-3
370. Valentine MT, Dewalt LE, Ou-Yang HD. Forces on a Colloidal Particle in a Polymer Solution: A Study Using Optical Tweezers. J Phys Condens Matter (1996) 8(47):9477–82. doi:10.1088/0953-8984/8/47/048
371. Bustamante C, Bryant Z, Smith SB. Ten Years of Tension: Single-Molecule DNA Mechanics. Nature (2003) 421(6921):423–7. doi:10.1038/nature01405
372. Ashkin A. Optical Trapping and Manipulation of Neutral Particles Using Lasers. Proc Natl Acad Sci (1997) 94(10):4853–60. doi:10.1073/pnas.94.10.4853
373. Gutsche C, Elmahdy MM, Kegler K, Semenov I, Stangner T, Otto O, et al. Micro-rheology on (Polymer-grafted) Colloids Using Optical Tweezers. J Phys Condens Matter (2011) 23(18):184114. doi:10.1088/0953-8984/23/18/184114
374. Knox SM, Whitelock JM. Perlecan: How Does One Molecule Do So many Things?. Cell. Mol. Life Sci. (2006) 63(21):2435–45. doi:10.1007/s00018-006-6162-z
375. Melrose J, Hayes AJ, Whitelock JM, Little CB. Perlecan, the "jack of All Trades" Proteoglycan of Cartilaginous Weight-Bearing Connective Tissues. BioEssays (2008) 30(5):457–69. doi:10.1002/bies.20748
376. Knox S, Fosang AJ, Last K, Melrose J, Whitelock J. Perlecan from Human Epithelial Cells Is a Hybrid Heparan/chondroitin/keratan Sulfate Proteoglycan. FEBS Lett (2005) 579(22):5019–23. doi:10.1016/j.febslet.2005.07.090
377. Jung M, Lord MS, Cheng B, Lyons JG, Alkhouri H, Hughes JM, et al. Mast Cells Produce Novel Shorter Forms of Perlecan that Contain Functional Endorepellin. J Biol Chem (2013) 288(5):3289–304. doi:10.1074/jbc.M112.387811
378. Siegel G, Malmsten M, Ermilov E. Anionic Biopolyelectrolytes of the Syndecan/perlecan Superfamily: Physicochemical Properties and Medical Significance. Adv Colloid Interf Sci (2014) 205:275–318. doi:10.1016/j.cis.2014.01.009
379. Guilak F, Hayes AJ, Melrose J. Perlecan in Pericellular Mechanosensory Cell-Matrix Communication, Extracellular Matrix Stabilisation and Mechanoregulation of Load-Bearing Connective Tissues. Int J Mol Sci (2021) 22(5):2716. doi:10.3390/ijms22052716
380. Iozzo RV. Basement Membrane Proteoglycans: From Cellar to Ceiling. Nat Rev Mol Cel Biol (2005) 6(8):646–56. doi:10.1038/nrm1702
381. Whitelock JM, Iozzo RV. Heparan Sulfate: A Complex Polymer Charged with Biological Activity. Chem Rev (2005) 105(7):2745–64. doi:10.1021/cr010213m
382. Sarrazin S, Lamanna WC, Esko JD. Heparan Sulfate Proteoglycans. Cold Spring Harbor Perspect Biol (2011) 3(7):a004952. doi:10.1101/cshperspect.a004952
383. Theocharis AD, Skandalis SS, Gialeli C, Karamanos NK. Extracellular Matrix Structure. Adv Drug Deliv Rev (2016) 97:4–27. doi:10.1016/j.addr.2015.11.001
384. Whitelock JM, Murdoch AD, Iozzo RV, Underwood PA. The Degradation of Human Endothelial Cell-Derived Perlecan and Release of Bound Basic Fibroblast Growth Factor by Stromelysin, Collagenase, Plasmin, and Heparanases. J Biol Chem (1996) 271(17):10079–86. doi:10.1074/jbc.271.17.10079
385. Elgundi Z, Papanicolaou M, Major G, Cox TR, Melrose J, Whitelock JM, et al. Cancer Metastasis: The Role of the Extracellular Matrix and the Heparan Sulfate Proteoglycan Perlecan. Front Oncol (2020) 9:1482. doi:10.3389/fonc.2019.01482
386. Woodham EF, Machesky LM. Polarised Cell Migration: Intrinsic and Extrinsic Drivers. Curr Opin Cel Biol (2014) 30:25–32. doi:10.1016/j.ceb.2014.05.006
387. Xia S, Chen Z, Shen C, Fu T-M. Higher-order Assemblies in Immune Signaling: Supramolecular Complexes and Phase Separation. Protein Cell (2021) 12:680–94. doi:10.1007/s13238-021-00839-6
388. Goult BT. The Mechanical Basis of Memory - the MeshCODE Theory. Front Mol Neurosci (2021) 14:592951. doi:10.3389/fnmol.2021.592951
389. Oldfield CJ, Dunker AK. Intrinsically Disordered Proteins and Intrinsically Disordered Protein Regions. Annu Rev Biochem (2014) 83(1):553–84. doi:10.1146/annurev-biochem-072711-164947
390. Wright PE, Dyson HJ. Intrinsically Disordered Proteins in Cellular Signalling and Regulation. Nat Rev Mol Cel Biol (2015) 16(1):18–29. doi:10.1038/nrm3920
391. Huntley MA, Golding GB. Simple Sequences Are Rare in the Protein Data Bank. Proteins (2002) 48(1):134–40. doi:10.1002/prot.10150
392. Tompa P, Fuxreiter M. Fuzzy Complexes: Polymorphism and Structural Disorder in Protein-Protein Interactions. Trends Biochem Sci (2008) 33(1):2–8. doi:10.1016/j.tibs.2007.10.003
393. Eisenberg D, Jucker M. The Amyloid State of Proteins in Human Diseases. Cell (2012) 148(6):1188–203. doi:10.1016/j.cell.2012.02.022
394. Bienz M. Signalosome Assembly by Domains Undergoing Dynamic Head-To-Tail Polymerization. Trends Biochem Sci (2014) 39(10):487–95. doi:10.1016/j.tibs.2014.08.006
395. Wu J, Lewis AH, Grandl J. Touch, Tension, and Transduction - the Function and Regulation of Piezo Ion Channels. Trends Biochem Sci (2017) 42(1):57–71. doi:10.1016/j.tibs.2016.09.004
396. Anderson P, Kedersha N, Ivanov P. Stress Granules, P-Bodies and Cancer. Biochim Biophys Acta (2015) 1849(7):861–70. doi:10.1016/j.bbagrm.2014.11.009
397. Hyman AA, Weber CA, Jülicher F. Liquid-Liquid Phase Separation in Biology. Annu Rev Cel Dev. Biol. (2014) 30(1):39–58. doi:10.1146/annurev-cellbio-100913-013325
398. Mitchell SF, Parker R. Principles and Properties of Eukaryotic mRNPs. Mol Cel (2014) 54(4):547–58. doi:10.1016/j.molcel.2014.04.033
399. Brangwynne CP, Tompa P, Pappu RV. Polymer Physics of Intracellular Phase Transitions. Nat Phys (2015) 11(11):899–904. doi:10.1038/nphys3532
400. Liang X, Michael M, Gomez G. Measurement of Mechanical Tension at Cell-Cell Junctions Using Two-Photon Laser Ablation. Bio Protoc (2016) 6(24):e2068. doi:10.21769/BioProtoc.2068
401. Swaney KF, Li R. Function and Regulation of the Arp2/3 Complex during Cell Migration in Diverse Environments. Curr Opin Cel Biol (2016) 42:63–72. doi:10.1016/j.ceb.2016.04.005
402. Case LB, Ditlev JA, Rosen MK. Regulation of Transmembrane Signaling by Phase Separation. Annu Rev Biophys (2019) 48(1):465–94. doi:10.1146/annurev-biophys-052118-115534
403. Case LB, Zhang X, Ditlev JA, Rosen MK. Stoichiometry Controls Activity of Phase-Separated Clusters of Actin Signaling Proteins. Science (2019) 363(6431):1093–7. doi:10.1126/science.aau6313 (
404. Huang WYC, Alvarez S, Kondo Y, Lee YK, Chung JK, Lam HYM, et al. A Molecular Assembly Phase Transition and Kinetic Proofreading Modulate Ras Activation by SOS. Science (2019) 363(6431):1098–103. doi:10.1126/science.aau5721
405. Cai D, Feliciano D, Dong P, Flores E, Gruebele M, Porat-Shliom N, et al. Phase Separation of YAP Reorganizes Genome Topology for Long-Term YAP Target Gene Expression. Nat Cel Biol (2019) 21(12):1578–89. doi:10.1038/s41556-019-0433-z
406. Cremer T, Cremer M, Hübner B, Strickfaden H, Smeets D, Popken J, et al. The 4D Nucleome: Evidence for a Dynamic Nuclear Landscape Based on Co-aligned Active and Inactive Nuclear Compartments. FEBS Lett (2015) 589(20 Pt A):2931–43. doi:10.1016/j.febslet.2015.05.037
407. Popken J, Brero A, Koehler D, Schmid VJ, Strauss A, Wuensch A, et al. Reprogramming of Fibroblast Nuclei in Cloned Bovine Embryos Involves Major Structural Remodeling with Both Striking Similarities and Differences to Nuclear Phenotypes Ofin Vitrofertilized Embryos. Nucleus (2014) 5(6):555–89. doi:10.4161/19491034.2014.979712
408. Bajpai G, Amiad Pavlov D, Lorber D, Volk T, Safran S. Mesoscale Phase Separation of Chromatin in the Nucleus. ELife (2021) 10:e63976. doi:10.7554/eLife.63976
409. Bundschuh R. Physical Biology of the Cell Physical Biology of the Cell , Rob Phillips , Jané Kondev and Julie Theriot Garland Science, New York, 2009. $125.00 (807 pp). ISBN 978-0-8153-4163-5. Phys Today (2009) 62(8):44. doi:10.1063/1.3206095
410. Rosa S, Shaw P. Insights into Chromatin Structure and Dynamics in Plants. Biology (2013) 2(4):1378–410. doi:10.3390/biology2041378
411. Meier DJ. Theory of Block Copolymers. I. Domain Formation in A-B Block Copolymers. J Polym sci., C Polym Symp (1969) 26(1):81–98. doi:10.1002/polc.5070260106
412. Farooqui R, Fenteany G. Multiple Rows of Cells behind an Epithelial Wound Edge Extend Cryptic Lamellipodia to Collectively Drive Cell-Sheet Movement. J Cel Sci (2005) 118(1):51–63. doi:10.1242/jcs.01577
413. Simpson KJ, Selfors LM, Bui J, Reynolds A, Leake D, Khvorova A, et al. Identification of Genes that Regulate Epithelial Cell Migration Using an siRNA Screening Approach. Nat Cel Biol (2008) 10(9):1027–38. doi:10.1038/ncb1762
414. Bindschadler M, McGrath JL. Sheet Migration by Wounded Monolayers as an Emergent Property of Single-Cell Dynamics. J Cel Sci (2007) 120(5):876–84. doi:10.1242/jcs.03395
415. Tambe DT, Corey Hardin C, Angelini TE, Rajendran K, Park CY, Serra-Picamal X, et al. Collective Cell Guidance by Cooperative Intercellular Forces. Nat Mater (2011) 10(6):469–75. doi:10.1038/nmat3025
416. Parisi G, Zamponi F. Mean-field Theory of Hard Sphere Glasses and Jamming. Rev Mod Phys (2010) 82(1):789–845. doi:10.1103/RevModPhys.82.789
417. Weeks ER. Three-Dimensional Direct Imaging of Structural Relaxation Near the Colloidal Glass Transition. Science (2000) 287(5453):627–31. doi:10.1126/science.287.5453.627
418. Hall RW, Wolynes PG. Intermolecular Forces and the Glass Transition. J Phys Chem B (2008) 112(2):301–12. doi:10.1021/jp075017j
419. Nelson CM, VanDuijn MM, Inman JL, Fletcher DA, Bissell MJ. Tissue Geometry Determines Sites of Mammary Branching Morphogenesis in Organotypic Cultures. Science (2006) 314(5797):298–300. doi:10.1126/science.1131000
420. Geiger B, Spatz JP, Bershadsky AD. Environmental Sensing through Focal Adhesions. Nat Rev Mol Cel Biol (2009) 10(1):21–33. doi:10.1038/nrm2593
421. Roca-Cusachs P, Gauthier NC, del Rio A, Sheetz MP. Clustering of 5 1 Integrins Determines Adhesion Strength whereas V 3 and Talin Enable Mechanotransduction. Proc Natl Acad Sci (2009) 106(38):16245–50. doi:10.1073/pnas.0902818106
422. Krishnan R, Park CY, Lin Y-C, Mead J, Jaspers RT, Trepat X, et al. Reinforcement versus Fluidization in Cytoskeletal Mechanoresponsiveness. PLoS ONE (2009) 4(5):e5486. doi:10.1371/journal.pone.0005486
423. Indra I, Gasparski AN, Beningo KA. An In Vitro Correlation of Metastatic Capacity and Dual Mechanostimulation. PLOS ONE (2018) 13(11):e0207490. doi:10.1371/journal.pone.0207490
424. Lou J, Stowers R, Nam S, Xia Y, Chaudhuri O. Stress Relaxing Hyaluronic Acid-Collagen Hydrogels Promote Cell Spreading, Fiber Remodeling, and Focal Adhesion Formation in 3D Cell Culture. Biomaterials (2018) 154:213–22. doi:10.1016/j.biomaterials.2017.11.004
425. Vining KH, Stafford A, Mooney DJ. Sequential Modes of Crosslinking Tune Viscoelasticity of Cell-Instructive Hydrogels. Biomaterials (2019) 188:187–97. doi:10.1016/j.biomaterials.2018.10.013
426. Baker BM, Trappmann B, Wang WY, Sakar MS, Kim IL, Shenoy VB, et al. Cell-mediated Fibre Recruitment Drives Extracellular Matrix Mechanosensing in Engineered Fibrillar Microenvironments. Nat Mater (2015) 14(12):1262–8. doi:10.1038/nmat4444
427. Braunecker WA, Matyjaszewski K. Controlled/living Radical Polymerization: Features, Developments, and Perspectives. Prog Polym Sci (2007) 32(1):93–146. doi:10.1016/j.progpolymsci.2006.11.002
428. Ong LL, Hanikel N, Yaghi OK, Grun C, Strauss MT, Bron P, et al. Programmable Self-Assembly of Three-Dimensional Nanostructures from 10,000 Unique Components. Nature (2017) 552(7683):72–7. doi:10.1038/nature24648
429. Händler T, Tutmarc C, Glaser M, Freitag JS, Smith DM, Schnauß J. Measuring Structural Parameters of Crosslinked and Entangled Semiflexible Polymer Networks with Single-Filament Tracing. Phys Rev E (2021) 103(6):062501. doi:10.1103/PhysRevE.103.062501
430. Rosales AM, Anseth KS. The Design of Reversible Hydrogels to Capture Extracellular Matrix Dynamics. Nat Rev Mater (2016) 1(2):15012. doi:10.1038/natrevmats.2015.12
Keywords: cytoskeletal mechanics, confinement, extracellular matrix, homogeneities, cancer cell, fibroblast, matrix degradation, forces
Citation: Mierke CT (2021) Bidirectional Mechanical Response Between Cells and Their Microenvironment. Front. Phys. 9:749830. doi: 10.3389/fphy.2021.749830
Received: 30 July 2021; Accepted: 30 September 2021;
Published: 20 October 2021.
Edited by:
Yuan Lin, The University of Hong Kong, Hong Kong, SAR ChinaReviewed by:
Youhua Tan, Hong Kong Polytechnic University, Hong Kong, SAR ChinaAndrey Cherstvy, University of Potsdam, Germany
Copyright © 2021 Mierke. This is an open-access article distributed under the terms of the Creative Commons Attribution License (CC BY). The use, distribution or reproduction in other forums is permitted, provided the original author(s) and the copyright owner(s) are credited and that the original publication in this journal is cited, in accordance with accepted academic practice. No use, distribution or reproduction is permitted which does not comply with these terms.
*Correspondence: Claudia Tanja Mierke, Y2xhdWRpYS5taWVya2VAdW5pLWxlaXB6aWcuZGU=