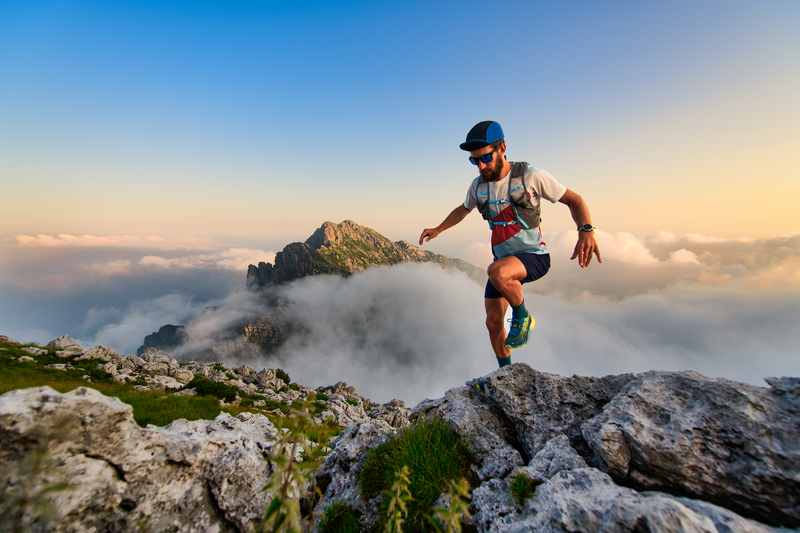
94% of researchers rate our articles as excellent or good
Learn more about the work of our research integrity team to safeguard the quality of each article we publish.
Find out more
BRIEF RESEARCH REPORT article
Front. Phys. , 16 August 2021
Sec. Optics and Photonics
Volume 9 - 2021 | https://doi.org/10.3389/fphy.2021.727109
This article is part of the Research Topic Nonlinear Optics With Structured Light View all 10 articles
A 7.8-GHz linewidth ytterbium-doped fiber (YDF) laser with an output power of 75 W at 1,018 nm is demonstrated based on narrow-bandwidth fiber Bragg gratings. Effective suppression of spectral broadening and amplified spontaneous emission is achieved by optimizing the resonator structure and active fiber parameters. An 1,178-nm diamond Raman output pumped by this narrow-linewidth 1,018 nm source is addressed in this study, which shows a promising application of generating the sodium guide star laser at 589 nm. A single-longitudinal-mode Stokes with an output power of 0.6 W is obtained using this multimode 1,018 nm laser at the pump power of 13 W. The impact of pump spectral linewidth on the effective Raman gain coefficient is analyzed, and the laser threshold of the diamond Stokes resonator increases with the broadening of the pump linewidth.
High-power 1,018 nm ytterbium-doped fiber (YDF) lasers [1–7] are attractive pump sources. In tandem-pumping configurations, 1,018 nm fiber lasers have been widely harnessed to pump YDF amplifiers and random fiber lasers, due to their advantages of low quantum defect, high beam brightness, and high efficiency. Besides, narrow spectral-linewidth 1,018 nm lasers are of intense interest for many applications in beam combining laser subsystem [8], frequency converted to 509 and 254 nm for laser spectroscopy [9] and atom trapping [10].
A promising application using high-power narrow-linewidth 1,018 nm fiber lasers is as the pump for a diamond Raman laser to generate 1,178 nm output through the first-order diamond Raman shift (39.99 THz). Frequency-doubled 1,178 nm lasers [11, 12] provide a crucial application for the adaptive optics system, acting as sodium laser beacon. The Raman gain profile of the diamond pumped by a single frequency source is a Lorentzian shape with a full-width at half-maximum (FWHM) linewidth of 45 GHz [13]. Pumped with a narrow-linewidth laser, a single-longitudinal-mode (SLM) Stokes output was directly available in a diamond Raman resonator due to the homogeneous Raman gain profile and the absence of spatial hole burning effect [14]. The combination of diamond’s ability to rapidly dissipate heat [15] and its gain nature of spatial hole burning free provides a pathway toward high-power SLM lasers. Our recently reported work, where SLM powers of 11.8 W at 1,240 nm and 38 W at 620 nm were achieved in a simple standing-wave diamond Raman frequency-doubling cavity pumped by a 1,064-nm laser with 3.3 GHz linewidth [16], brings forth a promising approach to demonstrate a high-power SLM 589 nm laser by exploiting a narrow-linewidth 1,018 nm pump.
For optical fiber lasers, spectral linewidth broadening, induced by fiber nonlinearity and dispersion [17–20], is the main challenge of generating narrow-linewidth lasers with a high output power. The output spectral linewidth of 1,018 nm emitting with an output power of around 100 W has been still more than 75 GHz [21, 22]. Another main challenge is the amplified spontaneous emission (ASE) at around 1,030 nm due to its higher gain in YDF than that at 1,018 nm. This is understood as follows. In a homogeneously broadened gain medium such as Yb-doped silica fiber, the gain at one wavelength is uniquely determined by the gain at two other wavelengths [23, 24]. Assuming pumping at 976 nm, the ASE gain at 1,030 nm is calculated by,
In this article, a linewidth of 7.8 GHz linearly polarized 1,018 nm YDF laser is demonstrated. At a pump power of 118 W, output power of 75 W was achieved corresponding to an optical to optical conversion efficiency of 64%. To the best of our knowledge, this is the narrowest linewidth reported of 1,018 nm fiber laser at this output power level. The 1,018 nm fiber laser was successfully used to generate the first-order Stokes laser at 1,178 nm in a standing-wave diamond Raman resonator. The 1,178 nm output characteristics were also experimentally investigated.
The schematic of experimental setup is shown in Figure 1. The 1,018 nm YDF laser is formed with a fiber resonator and a one-stage of YDF amplifier. A 27-W 976-nm laser diode (LD) was coupled into the fiber resonator as the pump through a combiner. The linear fiber resonator consisted of a pair of fiber Bragg gratings (FBGs) and 1.5 m long active fiber. The reflectivities of the high reflecting (HR) and output coupling (OC) FBGs were 98.5 and 17%, respectively. The center wavelengths of two FBGs were both located at about 1,017.9 nm with bandwidths of 0.4 and 0.08 nm, respectively, shown in the inset of Figure 1. The active fiber was a large core-to-cladding ratio Yb-doped double-cladding fiber with core and cladding diameters of 15 and 130 μm (LMA-YDF-15/130), and an absorption of 5.40 dB/m at 976 nm. A length of 50 m passive fiber (LMA-GDF-15/130) was fused between the active fiber and OC-FBG in order to increase the optical length of the oscillator. A fast-axis blocked polarization-maintaining (PM) optical isolator was inserted between the resonator and amplifier to enable only slow-axis polarized light passing and prevent the backward feedback into the resonator. In the fiber amplifier, two 60 W 976 nm LDs and a length of 0.9 m with core/cladding diameters of 20/130 μm PM LMA Yb-doped double cladding fiber (10 dB/m at 976 nm) were used to provide the pump and gain, respectively. Two pump strippers were used to remove the residual pump of the resonator and amplifier.
FIGURE 1. Diagram of 1,018 nm fiber laser and 1,178 nm diamond Raman laser (dotted box). LD: laser diode; PM: polarization maintaining; PLMA: polarized large mode area; YDF: Yb3+-doped fiber; GDF: germanium-doped fiber; HR FBG: highly reflective fiber Bragg grating; OC FBG: output-coupling fiber Bragg grating; 15/130 and 20/130: fiber core diameter of 15 or 20 μm, and a fiber-cladding diameter of 130 μm. The inset is the transmission spectra of HR (black) and OC (blue) FBGs.
• The 1,018 nm resonator was an all non-PM fiber connected linear structure. The length of active fiber was optimized to 1.5 m. The fast-axis blocked isolator acting as a polarizer enabled a linearly polarized output. The output power of the 1,018 nm seed laser was measured after the PM isolator. The threshold was about 1.5 W, and the output power increased to 2.6 W at the pump power of 11.8 W. Figure 2A depicts the output power of the amplifier as a function of pump power. When the pump power increased up to 118 W, the output power of 75 W was achieved corresponding to an optical-to-optical conversion efficiency of 64%. The maximum output power was limited by the available pump power. The output polarization extinction ratio was only about 12 dB at the output power of 75 W due to the insufficient polarization extinction ratio of the PM isolator.
• The output spectra of the 1,018 nm laser were analyzed using a spectrometer (HF-8997-2, LightMachinery; resolution of 0.8 GHz) equipped with an InGaAs camera. As is shown in Figure 3A, the spectrum of the seed laser was centered at 1,017.90 nm and had a FWHM linewidth of 6.6 GHz. The longitudinal mode spacing of the seed laser spectrum was about 1.9 MHz, corresponding to an oscillator length of about 53 m. The narrow spectral linewidth was generated by exciting partial longitudinal modes within the FBG reflection bandwidth due to a low-power pumping. In order to suppress spectral broadening and ASE, the length of the large core-cladding ratio gain fiber (PLMA 20/130) in the amplifier was shortened to 0.9 m. The spectral FWHM linewidth was slightly broadened from 6.6 to 7.8 GHz as the output power was increased from 2.3 to 75 W, as shown in Figure 3A. The slight redshift of central wavelength was observed due to the thermal induced refractive index decrease of the silica fiber. A broad spectrum at output power of 75 W was measured using an optical spectrum analyzer (YOKOGAWA, AQ6370B) with a resolution of 0.02 nm. The inset in Figure 3A depicts that the signal-to-noise ratio (SNR) between 1,018 and 1,030 nm was 49 dB, indicating excellent suppression of the ASE.
• A FBG resonator-based fiber laser usually has a temporal profile with high intensity fluctuation due to longitudinal modes beating. The intensity fluctuations of the pump laser is transferred to the stimulated Raman scattering process due to the short response time [25]. The resonator consists of a spool of 50 m passive fiber to decrease the longitudinal mode spacing and thus increase the number of longitudinal modes. Due to the longitudinal modes with random phases, the intensity fluctuations caused by modes beating decreased with the increase of the number of modes. The amplified 1,018 nm laser was collimated by a commercial pigtailed collimator which delivered an output beam diameter of 1.3 mm.
• The collimated continuous-wave (CW) 1,018 nm laser was injected into a standing-wave diamond Raman cavity after passing through a free-space isolator and a plano-convex focusing lens (f = 50 mm), shown in the dotted box in Figure 1. The output power evolution of the pump laser, measured after the free-space isolator, in about 1 h is shown in Figure 4. The starting power was 67.4 W, corresponding to a total loss of 10% that resulted from the collimator and isolator. The output power decreased slowly from 67.4 to 65.4 W in the first half hour, during which the laser diode and the gain fiber of the amplifier reached thermal stability. In the next half hour, the average output power stabilized at about 65.4 W. Note that there were periodic power fluctuations, due to the slow variation of the polarization state of the non-PM fiber seed oscillator. In a non-PM fiber, the state of the polarization is sensitive to the fiber birefringence which varies with temperature, pressure, and mechanical disturbances [26, 27]. The periodic variation (∼1.8 min) of the polarization state is likely to result from the periodic temperature fluctuation of the water chiller. One solution to the power fluctuations is to substitute the seed laser with an all-PM fiber oscillator.
• The diamond Raman resonator consisted of two plano-concave mirrors as the input coupler and output coupler, respectively. The input coupler with 50 mm radius curvature was highly transmissive (>98%) at 1,018 nm and highly reflective (>99.9%) at 1,178 nm. The OC had a 100-mm radius of curvature, was highly reflective (>99.9%) at 1,018 nm, and provided approximately 0.1% transmission at 1,178 nm. The diamond (Element Six Ltd., low-birefringence, low-nitrogen, CVD-grown single crystal) with dimensions of 8 mm × 4 mm × 1.2 mm was inserted at the waist of the near-concentric resonator.
FIGURE 2. (A) The output power of the fiber amplifier as a function of pump power. (B) Measured 1,178 nm Stokes power as a function of the incident pump power; inset: beam profiles for Stokes (top) and pump (bottom) at the maximum power.
FIGURE 3. (A) Output spectra of the 1,018 nm fiber laser at the output power of 2.3, 44.5, and 75 W; inset: a broad output spectrum at the output power of 75 W. (B) An SLM Stokes spectrum at the output powers of 0.6 W. (C) The Stokes spectrum at the output powers of 6.1 W; The Lorentzian curve of the Stokes spectrum (dotted red).
FIGURE 4. Output power stability evolution of the 1,018 nm fiber laser after the free-space isolator.
The output power of 1,178 nm Stokes is plotted in Figure 2B as a function of the incident 1,018 nm pump power. The threshold was 9.3 W, beyond which the 1,178 nm output only attained power of 6.1 W at the incident pump power of 63 W since the transmittance of the OC at 1,178 nm was only 0.1%. The effective Raman gain coefficient
• The beam profiles of pump and Stokes at maximum power are included in Figure 2B, which depicts an evidence of beam cleaning during stimulated Raman scattering in diamond [29]. Figure 3B shows the evolution of Stokes output spectrum through SLM to multi-longitudinal modes with the increasing of pump power due to the thermal drift in cavity length and nonlinear spectral broadening. Those spectra were measured by using a cavity mode–spacing resolved spectrometer (HF-8997-2, LightMachinery). Figure 3B shows an SLM spectrum with an output power of 0.6 W at the pump power 13 W, and the FWHM bandwidth is 0.8 GHz, which is the limitation of the spectrometer resolution. At the highest output power of 6.1 W, a multi-longitudinal mode Stokes spectrum was observed with a Lorentzian FWHM linewidth of 13.6 GHz, as shown in Figure 3C.
In summary, a high-power linearly polarized 1,018 nm fiber laser with 7.8 GHz linewidth is demonstrated based on a pair of narrow-bandwidth FBGs. The highest output power was 75 W, corresponding to an optical efficiency of 64%, and the spectral trace had a contrast of approximately 49 dB against the noise floor. One of the important applications for a narrow-linewidth 1,018 nm laser is to pump a diamond Raman laser for generating the first-order Stokes at 1,178 nm which can be frequency doubled to 589 nm for a sodium guide star laser. Here, the 1,018 nm fiber laser was successfully utilized in a standing-wave diamond Raman cavity to generate a power of 6.1 W and near-diffraction limit 1,178 nm output. And the spectral evolution of Stokes from a single mode to multimodes with the increase of pump power was observed.
The raw data supporting the conclusions of this article will be made available by the authors, without undue reservation.
All authors listed have made a substantial, direct, and intellectual contribution to the work and approved it for publication.
This work was funded by the National Natural Science Foundation of China (62005073) and the Natural Science Foundation of Hebei Province (F2020202026).
The authors declare that the research was conducted in the absence of any commercial or financial relationships that could be construed as a potential conflict of interest.
All claims expressed in this article are solely those of the authors and do not necessarily represent those of their affiliated organizations, or those of the publisher, the editors and the reviewers. Any product that may be evaluated in this article, or claim that may be made by its manufacturer, is not guaranteed or endorsed by the publisher.
We are thankful for the support from Key Laboratory of OptoElectronic Information Technology, Ministry of Education (Tianjin University).
1. Stiles E. New Developments in IPG Fiber Laser Technology. Presented in 5th International Workshop on Fiber Lasers. Germany: Dresden (2009).
2. Zhu J, Zhou P, Ma Y, Xu X, Liu Z. Power Scaling Analysis of Tandem-Pumped Yb-Doped Fiber Lasers and Amplifiers. Opt Express (2011) 19:18645–54. doi:10.1364/oe.19.018645
3. Du X, Zhang H, Xiao H, Zhou P, Liu Z. Temporally Stable Random Fiber Laser Operates at 1070 Nm. IEEE Photon J. (2015) 7:1–7. doi:10.1109/JPHOT.2015.2477279
4. Yu W, Xiao Q, Wang L, Zhao Y, Qi T, Yan P, et al. 2196 W Large-Mode-Area Er:Yb Codoped Fiber Amplifier Operating at 1600 Nm Pumped by 1018 Nm Fiber Lasers. Opt Lett (2021) 46:2192–5. doi:10.1364/OL.424368
5. Yan P, Wang X, Li D, Huang Y, Sun J, Xiao Q, et al. High-power 1018 Nm Ytterbium-Doped Fiber Laser with Output of 805 W. Opt Lett (2017) 42:1193–6. doi:10.1364/OL.42.001193
6. Xiao H, Zhou P, Wang XL, Xu XJ, Liu ZJ. High Power 1018 Nm Ytterbium Doped Fiber Laser with an Output Power of 309 W. Laser Phys Lett (2013) 10:065102. doi:10.1088/1612-2011/10/6/065102
7. Zhou P, Xiao H, Leng J, Xu J, Chen Z, Zhang H, et al. High-power Fiber Lasers Based on Tandem Pumping. J Opt Soc Am B (2017) 34:A29–A36. doi:10.1364/JOSAB.34.000A29
8. Khitrov V, Farley K, Leveille R, Galipeau J, Majid I, Christensen S, et al. kW Level Narrow Linewidth Yb Fiber Amplifiers for Beam Combining. SPIE Defense, Security, and Sensing, 8. Bellingham WA: SPIE (2010). doi:10.1117/12.862648
9. Rye HS, Yue S, Wemmer DE, Quesada MA, Haugland RP, Mathies RA, et al. Stable Fluorescent Complexes of Double-Stranded DNA with Bis-Intercalating Asymmetric Cyanine Dyes: Properties and Applications. Nucl Acids Res (1992) 20:2803–12. doi:10.1093/nar/20.11.2803
10. Villwock P, Siol S, Walther T. Magneto-optical Trapping of Neutral Mercury. Eur Phys J D (2011) 65:251–5. doi:10.1140/epjd/e2011-20064-9
11. Feng Y, Taylor LR, Calia DB. 25 W Raman-Fiber-Amplifier-Based 589 Nm Laser for Laser Guide star. Opt Express (2009) 17:19021–6. doi:10.1364/oe.17.019021
12. Yang X, Zhang L, Cui S, Fan T, Dong J, Feng Y. Sodium Guide star Laser Pulsed at Larmor Frequency. Opt Lett (2017) 42:4351–4. doi:10.1364/ol.42.004351
13. Mildren RP. Intrinsic Optical Properties of Diamond. Wiley-VCH, Wiley (2013). doi:10.1002/9783527648603.ch1
14. Lux O, Sarang S, Kitzler O, Spence DJ, Mildren RP. Intrinsically Stable High-Power Single Longitudinal Mode Laser Using Spatial Hole Burning Free Gain. Optica (2016) 3:876–81. doi:10.1364/optica.3.000876
15. Bai Z, Zhang Z, Wang K, Gao J, Zhang Z, Yang X, et al. Comprehensive Thermal Analysis of Diamond in a High-Power Raman Cavity Based on FVM-FEM Coupled Method. Nanomaterials (2021) 11:1572. doi:10.3390/nano11061572
16. Yang X, Kitzler O, Spence DJ, Williams RJ, Bai Z, Sarang S, et al. Single-frequency 620 Nm diamond Laser at High Power, Stabilized via Harmonic Self-Suppression and Spatial-hole-burning-free Gain. Opt Lett (2019) 44:839–42. doi:10.1364/ol.44.000839
17. Babin SA, Churkin DV, Ismagulov AE, Kablukov SI, Podivilov EV. Spectral Broadening in Raman Fiber Lasers. Opt Lett (2006) 31:3007–9. doi:10.1364/ol.31.003007
18. Kablukov SI, Zlobina EA, Podivilov EV, Babin SA. Output Spectrum of Yb-Doped Fiber Lasers. Opt Lett (2012) 37:2508–10. doi:10.1364/OL.37.002508
19. Soh DBS, Koplow JP, Moore SW, Schroder KL, Hsu WL. The Effect of Dispersion on Spectral Broadening of Incoherent Continuous-Wave Light in Optical Fibers. Opt Express (2010) 18:22393–405. doi:10.1364/oe.18.022393
20. Liu W, Miao Y, Ma P, Zhou P, Jiang Z. Theoretical Study of Narrow-Linewidth Hybrid Rare-Earth-Raman Fiber Amplifiers. Opt Express (2019) 27:14523–35. doi:10.1364/oe.27.014523
21. Jiang M, Zhou P, Xiao H, Ma P. A High-Power Narrow-Linewidth 1018 Nm Fiber Laser Based on a Single-Mode-Few-Mode-Single-Mode Structure. High Pow Laser Sci Eng (2015) 3:e25. doi:10.1017/hpl.2015.24
22. Midilli Y, Efunbajo OB, şimşek B, Ortaç B. 1018 Nm Yb-Doped High-Power Fiber Laser Pumped by Broadband Pump Sources Around 915 Nm with Output Power above 100 W. Appl Opt (2017) 56:7225–9. doi:10.1364/ao.56.007225
23. Palma-Vega G, Walbaum T, Heinzig M, Kuhn S, Hupel C, Hein S, et al. Ring-up-doped Fiber for the Generation of More Than 600 W Single-Mode Narrow-Band Output at 1018 Nm. Opt Lett (2019) 44:2502–5. doi:10.1364/OL.44.002502
24. Nilsson J, Minelly JD, Paschotta R, Tropper AC, Hanna DC. Ring-doped Cladding-Pumped Single-Mode Three-Level Fiber Laser. Opt Lett (1998) 23:355–7. doi:10.1364/ol.23.000355
25. Krause M, Cierullies S, Renner H, Brinkmeyer E. Pump-to-Stokes RIN Transfer in Raman Fiber Lasers and its Impact on the Performance of Co-pumped Raman Amplifiers. Opt Commun (2006) 260:656–61. doi:10.1016/j.optcom.2005.10.077
26. Ulrich R. Polarization Stabilization on Single‐mode Fiber. Appl Phys Lett (1979) 35:840–2. doi:10.1063/1.90999
27. Kidoh Y, Suematsu Y, Furuya K. Polarization Control on Output of Single-Mode Optical Fibers. IEEE J Quan Electron. (1981) 17:991–4. doi:10.1109/JQE.1981.1071206
28. Sabella A, Spence DJ, Mildren RP. Pump-Probe Measurements of the Raman Gain Coefficient in Crystals Using Multi-Longitudinal-Mode Beams. IEEE J Quan Electron. (2015) 51:1–8. doi:10.1109/JQE.2015.2503404
Keywords: fiber laser, stimulated Raman scattering, second harmonic generation, solid state laser, diamond Raman laser
Citation: Yang X, Bai Z, Jiang H, Mildren RP and Feng Y (2021) A Narrow-Linewidth Linearly Polarized 1018-nm Fiber Source for Pumping Diamond Raman Laser. Front. Phys. 9:727109. doi: 10.3389/fphy.2021.727109
Received: 18 June 2021; Accepted: 12 July 2021;
Published: 16 August 2021.
Edited by:
Zhi-Han Zhu, Harbin University of Science and Technology, ChinaReviewed by:
Shijie Fu, University of Arizona, United StatesCopyright © 2021 Yang, Bai, Jiang, Mildren and Feng. This is an open-access article distributed under the terms of the Creative Commons Attribution License (CC BY). The use, distribution or reproduction in other forums is permitted, provided the original author(s) and the copyright owner(s) are credited and that the original publication in this journal is cited, in accordance with accepted academic practice. No use, distribution or reproduction is permitted which does not comply with these terms.
*Correspondence: Xuezong Yang, eHVlem9uZy55YW5nQHVjYXMuYWMuY24=
Disclaimer: All claims expressed in this article are solely those of the authors and do not necessarily represent those of their affiliated organizations, or those of the publisher, the editors and the reviewers. Any product that may be evaluated in this article or claim that may be made by its manufacturer is not guaranteed or endorsed by the publisher.
Research integrity at Frontiers
Learn more about the work of our research integrity team to safeguard the quality of each article we publish.