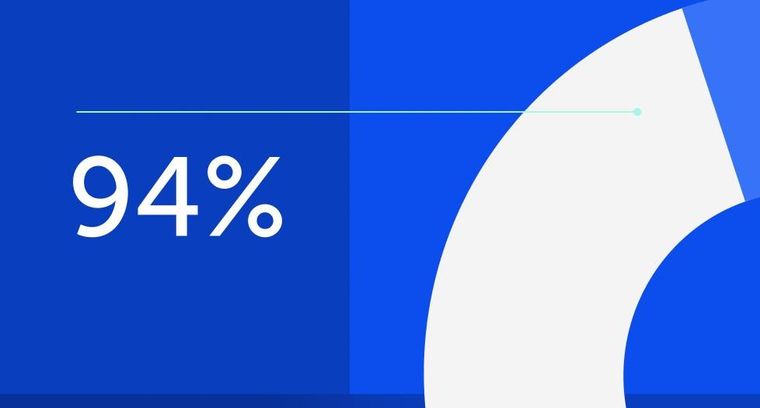
94% of researchers rate our articles as excellent or good
Learn more about the work of our research integrity team to safeguard the quality of each article we publish.
Find out more
REVIEW article
Front. Phys., 01 June 2021
Sec. Low-Temperature Plasma Physics
Volume 9 - 2021 | https://doi.org/10.3389/fphy.2021.683118
This article is part of the Research TopicLow-Temperature Plasma for Biomedical ApplicationsView all 10 articles
Pathogenic viruses cause many human, animal, and plant diseases that are associated with substantial morbidity, mortality and socio-economic impact. Although effective strategies for combatting virus transmission and associated disease are available, global outbreaks of viral pathogens such as the virus responsible for the COVID-19 pandemic demonstrate that there is still a critical need for new approaches that can be used to interrupt the chain of viral infection and mitigate virus-associated pathogenesis. Recent studies point to non-thermal plasma (NTP), a partly ionized gas comprised of a complex mixture of reactive oxygen and nitrogen species along with physical effectors, as the potential foundation for new antiviral approaches. A more thorough understanding of the antiviral properties and safety of NTP has stimulated explorations of NTP as the basis for treatments of viral diseases. The recently described immunomodulatory properties of NTP are also being evaluated for potential use in immunotherapies of viral diseases as well as in antiviral vaccination strategies. In this review, we present the current state-of-the-art in addition to compelling arguments that NTP merits further exploration for use in the prevention and management of viral infections and associated diseases.
Viruses are a major group of ubiquitously present microbes responsible for many acute and chronic infectious diseases. Viral infections spread easily, either from person to person or through contact with contaminated objects or ingestion of contaminated foods. Some of these infections are self-limiting, while others require aggressive therapeutic interventions or lead to loss of life [1]. Viruses also challenge food safety and cause tremendous economic loss in agriculture due to infection of plants or livestock [2, 3]. As an example of the global impact of viruses, the current coronavirus disease-2019 (COVID-19) pandemic has brought to the forefront unresolved challenges in prevention and treatment of viral diseases. Severe Acute Respiratory Syndrome Coronavirus 2 (SARS-CoV-2), the viral cause of this pandemic, has become the source of widespread morbidity, mortality, and economic burden [4, 5].
Despite extensive efforts directed toward new drug development, there are few effective, broad-spectrum antiviral drugs available. Prevention of infection is currently the most effective method of combating virus infection and associated disease. There is a dire need for novel approaches that can be used to prevent and control viral infections. Recently, there has been increased interest in defining roles for non-thermal plasma (NTP), an ionized gas containing a complex mixture of reactive oxygen and nitrogen species, in strategies effective against viral infection and disease. This review discusses the potential for NTP in mitigating viral infections and the points of intervention where state-of-the-art plasma medicine approaches can be applied. We also highlight collaboration opportunities for engineers and virologists to develop novel methods for both prevention and treatment of viral infections.
Viral infections have been the cause of considerable disease and many deaths around the globe for centuries. The Antonine plague in the second century A.D., now believed to be attributed to either the smallpox or measles virus, is the earliest documented instance of a large-scale viral outbreak (although cases of smallpox are suspected to have occurred in China and Egypt in 3 BCE) [6, 7]. In modern history, smallpox outbreaks were documented starting in the early 16th century. Eradicated in 1980, smallpox virus was the cause of disfigurement and loss of sight in many who were infected with this pathogen and, by conservative estimates, the cause of over 500 million deaths during the 20th century [6]. The Spanish flu, attributed to the spread of the H1N1 influenza virus in 1918–1920, had the second highest lethality rate, but took only two years to kill 40–50 million people, representing 2.1% of the world population [8]. Because this pandemic strain of influenza virus disproportionately affected young adults, its socio-economic impact was tremendous.
The acquired immunodeficiency syndrome (AIDS) epidemic, caused by the human immunodeficiency virus type 1 (HIV-1), is another infection associated with widespread loss of life. Current estimates of the HIV-1 global impact have reached 33 million lives lost since the mid-1970s and over 38 million people now living with pharmaceutically-controlled HIV-1 infection [9]. The societal and demographic impact of AIDS at the level of families, communities, and nations has been considerable and has driven major policy decisions [10]. The worldwide spread of HIV-1 fostered the development of new public health measures, new surveillance programs, and expanded international cooperation in the management of emerging diseases.
In the 21st century, coronaviruses have been the cause of the most frequently emerging epidemics and pandemics. These include the 2002 Severe Acute Respiratory Syndrome-1 (SARS-1) outbreak, the 2012 spread of the Middle Eastern Respiratory Syndrome (MERS), and the current COVID-19 pandemic [11]. Just over one year after the initial outbreaks, SARS-CoV-2 continues to spread through populations on all seven continents, resulting in a total of 119,220,681 cases and 2,646,826 deaths (as of March 10th, 2021) [12]. The emergence of new viral variants, such as the B.1.1.7 variant, further complicates efforts to contain the virus and raises important questions about the efficacies of approved vaccines that were developed against the original viral isolate [13]. The COVID-19 pandemic continues to be the cause of considerable morbidity, mortality, socio-economic burden and brings acute attention to the disruptive potential of emerging pathogenic viruses and the urgent need to find new methods for stopping them [4, 5].
While not the cause of massive global outbreaks, other viruses nevertheless present significant public health challenges. For example, the measles virus continues to reemerge in the form of local outbreaks every 2–3 years resulting in loss of life, mainly in children under 5 years of age [14]. Because the measles virus is highly contagious, exposure almost always results in infection in the unimmunized. Since the introduction of the measles vaccine in 1963, global deaths attributable to measles virus infection have gone down by 73% [14]. Other viruses, particularly those associated with foodborne illnesses, continue to cause outbreaks around the world due to inefficient decontamination of consumable products. Such is the case for norovirus, which annually causes close to 700 million cases of gastrointestinal illness worldwide, many of which require hospitalizations [15]. Inefficient decontamination also contributes to ongoing outbreaks caused by respiratory viruses that cause the common cold and influenza. The potentially lethal influenza virus A, the cause of the seasonal flu, causes hundreds of thousands of deaths annually [16]. Analogous to virus outbreaks among humans, loss of livestock due to disease caused by viruses like avian influenza and porcine reproductive respiratory syndrome virus (PRRSV) or loss of economic crops from uncontrolled infections by plant viral pathogens like tobacco mosaic virus result in the loss of billions of dollars annually in the U.S. alone [2].
Viruses are submicroscopic particles that are dependent on a host cell for replication. They are not considered “living” but have unique structural elements that allow them to enter (infect) and hijack living cells for the purpose of replication. In general, most viruses consist of a capsid (protein) that encloses the genetic material (nucleic acid) carrying the codes for their structural and functional components [17] (Figure 1). The capsid is made of small subunits arranged in specific patterns that give each virus its unique shape and host cell specificity. Some viruses are naked, consisting of the protein capsid as the outermost layer of the virus. Other viruses are enveloped, which means that they are surrounded by a membrane derived from the host cell. The envelope is composed of phospholipids acquired from the host cell as well as glycoproteins that are encoded by the virus and are key to virus entry into the host cell. Because of the fragility of the envelope, enveloped viruses are generally more sensitive to environmental factors (e.g., low humidity and increased temperature) that adversely affect the structure of the virus and reduce its infectivity [17]. Both types of viruses—including enveloped (SARS-CoV-2, influenza virus A, and HIV-1) and non-enveloped (norovirus and rhinoviruses)—have caused considerable economic disruption and loss of life [4, 5, 18–22].
FIGURE 1. Structure of a generic enveloped and non-enveloped virus. Viruses may be enveloped, containing an outermost layer of glycoproteins surrounding the capsid (A), or non-enveloped (B). They carry necessary genetic information on their nucleic acid (DNA or RNA) that is enclosed in a capsid.
The information necessary for a virus to replicate and cause disease is stored in the viral genome, which can take the form of DNA or RNA. As a consequence, DNA and RNA viruses use viral enzymes called DNA- or RNA-dependent polymerases, respectively, to facilitate replication in the host cell. During replication, the nucleic acids of RNA viruses are more prone to accumulate mutations relative to DNA viruses. Mutations that confer a survival advantage for the virus include resistance to antiviral drugs [23, 24] or the ability to replicate in new species [25]. Several of the emerging or re-emerging viruses that are contemporary threats to global public health are RNA viruses, including the coronavirus SARS-CoV-2, Zika virus, influenza virus A, Ebola virus, and HIV-1 [23–25].
Despite the simple structure of viruses and their genomes, interactions between viruses and their host cells during viral replication are quite complex, largely because viruses are obligatory intracellular parasites. A single virus particle or virion is sufficient to initiate infection, which is accomplished by the attachment of proteins on the virus capsid or envelope to corresponding receptors on the cell surface. This interaction defines the host range and cellular tropism of a virus and also facilitates the entry of the virus into the cell so that it can access the cellular machinery to replicate its genome and assemble new copies of itself. It is during the stage of egress from the cell that enveloped viruses acquire their host-derived envelope through the process of budding. In contrast, most non-enveloped viruses lyse their host cells as the endpoint of replication cycle. Newly produced progeny virions (infectious virus particles) that exit their host cells by either mechanism can attach to and infect new cells, further facilitating their spread (Figure 2). The time required for a single replication cycle (the process that starts with virus entry and concludes with the release of new viruses from the infected cell) varies considerably between viruses. For example, the replication cycle of the influenza virus can be as short as 6 h while the replication cycle for HIV-1 is completed in approximately 24 h [26, 27]. The consequence of a faster replication cycle can be the production of higher numbers of progeny viruses and faster spread of the infection.
FIGURE 2. Life cycle of a generic virus. Infection of a host cell begins with attachment of glycoproteins (enveloped virus) or capsid proteins (non-enveloped) to receptors on the host cell (1). Replication of the viral DNA or RNA then occurs using the host cell machinery (2), followed by assembly of new virions (3) and release of newly assembled virions (4) that are released to subsequently infect other host cells.
Some viruses, such as herpes simplex virus type 1 (HSV-1) and HIV-1, infect the host cell and then establish a state of dormancy (latency) in which the viral genome persists in the host cell but is not used to synthesize viral proteins or new viruses for some time. Latently infected cells pose a particular challenge to the host, because they may be undetected by host immune defenses and may be unaffected by antiviral drug therapies [28, 29].
A productive virus replication cycle produces more copies of the virus that spread to other cells in the infected person and can also be transmitted to other individuals. Control of transmission can be accomplished through efficient disinfection strategies to prevent exposure of new hosts to the virus. Additionally, strategies that provide prophylactic protection from infection or a therapeutic host immune response to an established infection also reduce the risk of virus transmission.
Viruses spread among populations by a process called transmission, in which uninfected individuals become infected subsequent to virus exposure. Most infectious viruses are spread by direct contact with a contaminated item (fomite) in the environment or an infected individual. Therefore, spread of infection in a community may be controlled by creating spatio-temporal distance between an individual and the source of the virus. This may be achieved by the following measures, implemented separately or in combination: disinfection/sanitation of the environment to reduce the quantity of viruses, physical barriers to reduce contact with viruses, prophylactic drugs and vaccines to prevent disease, isolation of infected individual(s) (quarantine), and therapeutics for infected individuals.
The ease with which a virus becomes transmitted from person to person depends on the production of transmissible virus during an infection and the size of the inoculum. After the initial infection, there is a lag period, called the incubation period, during which time the virus replicates productively. The infected individual may or may not have clinical symptoms during the incubation period but may still be capable of transmitting the virus to others. For example, a person infected with varicella-zoster virus (VZV) is contagious up to 1–2 days before the appearance of lesions characteristic of varicella (chickenpox). Asymptomatic transmission has also been a major challenge in containing the COVID-19 pandemic and the spread of SARS-CoV-2, as infected individuals who are asymptomatic can spread the virus to the vulnerable in close proximity [30]. This is also true of infections by other viruses (e.g., HIV-1), for which clinically apparent symptoms may not develop until several days or months after infection [31, 32] but the asymptomatic individual can serve as a carrier and spread the pathogen.
The route of transmission also has an important role in sustained virus transmission within a population. Viruses like HIV-1 and HSV-1 are spread primarily through direct, physical contact with infected individuals. In contrast, airborne transmission of viruses like influenza virus A and measles virus may involve the spread of the virus over distances exceeding 2 m, resulting in the infection of multiple people simultaneously despite control measures [22, 33]. VZV can be spread not only by direct contact, but also by inhalation of aerosolized fluids from skin lesions and potentially by inhalation of respiratory secretions exhaled by someone infected by VZV. SARS-CoV-2 is transmitted between people through contact with or inhalation of virus-laden respiratory droplets [34, 35]. These droplets also settle on objects and contaminate surfaces with viruses that may remain infectious for several hours to a few days [36, 37].
Infectious large and small droplets have typical travel lengths of less than 6 feet. However, mucus or water evaporation from the droplets create sub-micrometer- to micrometer-sized droplet nuclei that can remain airborne nearly indefinitely and travel due to indoor air currents created by heating, ventilation, and air-conditioning (HVAC) systems and by the movement of building occupants. As an example of long-distance infection, livestock and poultry farms are subject to airborne transmission of disease-causing viruses. Investigations of the distances to which viruses could be carried in the air demonstrated detection of RNA of an animal coronavirus in the air at 16.1 km from an infected farm in the US [38]. Although many viruses are transmitted via aerosols, the potential of airborne transmission has been an overlooked problem [39], at least until the COVID-19 pandemic.
In humans, viruses can be cleared by host defense mechanisms once an infection has been detected. Defense against a viral infection begins with the non-specific innate immune response followed by activation of the virus-specific adaptive immune response, which may be cell-mediated (T cell), or antibody-mediated (B cell). Many common viral infections can be cleared by these host defense mechanisms before clinically apparent symptoms develop [40–43] (Figure 3).
FIGURE 3. Course of disease and the associated immune responses after exposure to an infectious virus. Exposure to an infectious virus is followed by an asymptomatic incubation period (1) during which the virus replicates. During this phase, the tissue-resident innate immune responses are initiated but may still allow disease symptoms to develop (2A). Stimulation of protective, adaptive immune responses results in resolution (3A) of the disease. In some cases, the patient may remain asymptomatic (2B) because of a strong adaptive immune response. Certain viruses can transition to a chronic disease (3B) while others may have a fatal outcome (3C). Successful immune responses may also lead to viral clearance.
The first line of defense against viral infection is the innate immune system, which can detect free virus as well as infected host cells that display distinct markers on their surface that reflect ongoing virus replication and virus-associated cell death [44]. Recognition of either free virus or virus-infected cells is accomplished by dendritic cells or macrophages, often called sentinel cells or antigen presenting cells (APCs), because they constantly survey the body for invading pathogens and infected cells. These cells engulf and break down the virus or virus-infected cell through the process of phagocytosis. Activation and recruitment of APCs is a critical step for engagement of innate cells key for inhibition of viral infection. APCs display the digested viral peptides on their cell surface and migrate to local lymph nodes to present them to the adaptive immune cells (T and B cells) in order to produce a cell or antibody-mediated response, respectively. Both responses target the specific virus against which they are generated and usually have no effect against other types of viruses [40–43].
The second host defense mechanism is the adaptive immune response, which is a long-term response to infection. It takes the form of a cell-mediated response and a process that results in the production of pathogen-specific antibodies. The cell-mediated immune response depends on activation of CD8+ T cells that can recognize the viral peptide displayed on APCs by virtue of an appropriate receptor on their cell membrane. This response can be broadened by engagement of CD4+ T helper cells that also recognize that specific virus and provide additional signals to enhance the antiviral function of CD8+ T cells. Once CD8+ T cells are activated, they proliferate to expand and then migrate to different sites in the body to kill the virus-infected cells displaying the viral antigen, achieving clearance of infection. A small subset of these virus-specific CD8+ T cells become long-lived memory cells and may be called back into service at a later time to prevent disease caused by the same virus. Vaccines rely on the use of pathogen-derived antigens to generate memory responses that provide specific recognition of infected cells and their elimination by CD8+ T cells [40–43].
An antibody-mediated immune response is mounted by B cells, which can be activated by interactions with APCs and/or signals from the CD4+ T helper cells. Activated B cells mature to produce antibodies that specifically bind to free virus, preventing it from binding to uninfected host cells by a process called neutralization. The generation of neutralizing antibodies is thus critical for reducing viral burden in the body, which enables the cell-mediated immune response to be more effective at controlling the disease. Like the CD8+ T cells, memory B cells are formed, providing protection against a later re-exposure to the virus [40–43].
Despite innate and adaptive immune responses, many viruses often succeed in causing clinical disease. A virus may gain a foothold in the host due to deficiencies in immune responses (such as an inability of immune cells to recognize infected cells), a lack of engagement between innate and adaptive immune cells, or inefficient killing of infected cells by either innate or adaptive immune cells [45]. Additionally, mutations in the capsid or envelope of the virus that prevent it from being neutralized by antibodies or provide other survival and transmission benefits contribute to its continued propagation. A poor clinical outcome from viral diseases can also be caused by an intensified immune response. In some diseases (including COVID-19), the clinical outcome may be worsened by severe, dysregulated inflammation that occurs in response to the virus infection [45, 46]. Each of these factors could potentially counter strategies intended to prevent viral infections or treat virus-associated diseases.
Current antiviral strategies are aimed at mitigating host exposure to the virus (prevention of transmission), prevention of infection or disease development following exposure (prophylaxis), or treatment of disease. All three approaches, if effective, can collectively decrease the prevalence of viruses in the population over time, thus minimizing the impact of virus infections in the population and potentially leading to its eradication.
Antiviral strategies that focus on preventing transmission target early events in the spread of the virus. One important preventative strategy that serves to limit individuals’ exposure to a virus in the environment is disinfection, intended to reduce the virus load on surfaces. This can be accomplished by using chemical agents (e.g., bleach), alcohol, and oxidizing agents (e.g., hydrogen peroxide or chlorine) that disrupt the viral envelope or capsid [47]. However, these approaches are not feasible against viruses that are airborne (such as influenza and coronaviruses). For airborne viruses, high-efficiency particulate air (HEPA) filters are the most efficacious approach available for removing virus-containing droplets and aerosols from the air. It is estimated that 99.97% of aerosolized particles of 3 μm in size can be captured with HEPA filters, making them suitable for capturing droplets or aerosols that potentially contain infectious viruses [48, 49]. However, efficacy in viral decontamination and the ability to capture viruses depends critically on airflow [50, 51]. While HEPA filters are highly effective in HVAC systems, most HVAC systems in office buildings have less than one air exchange per hour. As a consequence, removal of virus-containing aerosols and droplets by HEPA filtration of recirculated room air may be less effective. Decontamination of more contained indoor spaces can be accomplished with exposure of room surfaces to ultraviolet light (UV), which causes damage to viral proteins and the genome [52]. Despite the frequent use of these control measures, there remains a risk of exposure to respiratory viruses in especially densely populated environments.
Prophylaxis is a strategy used to prevent initial infection with a virus, prevent the transition to virus-associated disease, or reduce the severity of disease caused by the infection. It may be achieved through the use of vaccines that stimulate immunity and are intended to provide long-term protection against subsequent exposure(s) with the same virus. Antiviral drugs, on the other hand, limit viral replication early in infection, usually prior to emergence of symptoms, and are typically administered after a suspected virus exposure. These drugs may not be effective if the infection has progressed too far or once disease symptoms have developed. For example, oseltamivir (Tamiflu) prevents emergence of influenza symptoms, but only when administered within the first 48 h after exposure to the virus [53, 54]. Prophylaxis for disease prevention or mitigation subsequent to infection is not to be confused with prophylactic approaches intended to prevent the initial infection. For example, pre-exposure prophylaxis (PreP) is a drug-based regimen designed to protect people from becoming infected with HIV-1 rather than a therapy for preventing HIV-1-associated disease in those who are already infected.
The most effective prophylactic measure against viral diseases is a vaccine, which evokes a pathogen-specific immune response. Vaccines function by educating the host immune system to create immunological memory against a specific virus in response to administration of a subset component of the virus or an inactivated virus. This protects the host against disease upon a subsequent encounter with the virus [55] because the reactivated immune cells clear the virus and infected cells soon after re-exposure. Several vaccines specific to viral diseases like smallpox (now eradicated), measles, and poliovirus are part of the regular childhood vaccination schedule. These and other vaccines are advantageous relative to prophylactic drugs because they build lifelong immunity that potentially protects immunized individuals long after the vaccine was administered.
When an infected person develops disease symptoms, one recourse is treatment with antiviral drugs to control the severity of symptoms and also prevent the spread of virus in the community. Since viruses replicate inside the cells of the host, such drugs need to have high selectivity, i.e., a high degree of antiviral efficacy with little or no effect on host cell viability, metabolism, or functions. Antiviral drugs target virus-specific proteins or enzymes to inhibit viral replication and reduce the virus burden. In some cases, this allows the immune system to engage, resulting in the resolution of infection by complete elimination of the virus from the body and establishment of long-term immunity. In other diseases caused by viral pathogens like HIV-1 or hepatitis C, the antiviral drugs control or prevent severe disease but cannot affect the virus that has established a latent infection [28, 56]. The symptoms and severity of a viral infection may also be moderated by drugs that are administered to either boost the host’s antiviral immune response, as in AIDS, or dampen it, as in COVID-19 [57, 58]. The therapeutic options available for viral infections are limited to a few diseases; there is an urgent need for broad-spectrum antiviral approaches, as is highlighted by the emergence of many recent viral outbreaks and global epidemics.
Non-thermal plasma has emerged as a technology with the potential to be used to prevent viral infection and disease. NTP is an ionized gas containing free energetic electrons that enable a highly reactive environment at near ambient temperatures. The plasma-produced free energetic electrons have energies that are large enough to electronically excite, dissociate, and ionize molecules, and produce more than 80 different species in humid air [59, 60]. The resulting highly reactive oxygen and nitrogen species (RONS) are extremely effective in inactivation of pathogens. We provide evidence in this section that NTP technology has potential as a new broad-spectrum antiviral strategy and has several advantages over existing decontamination technologies. Investigation of the antiviral effects of this technology has increased around the world in the last ten years (Figure 4). A summary of the studies investigating antiviral strategies utilizing NTP application is provided in Table 1. We briefly review conventional decontamination technologies with some of their limitations and contrast them with NTP technology.
FIGURE 4. Timeline and distribution of studies by geography investigating the antiviral effects of NTP (data for 2021 includes publications as of March 10, 2021). The number of studies utilizing NTP for antiviral applications increased over the last ten years (A) and were predominantly performed in the United States (B). Abbreviations: AUS: Australia; BRA: Brazil; CZE: Czech Republic; CHN: China; FRA: France; GER: Germany; GBR: Great Britain; IRN: Iran; ITA: Italy; KOR: South Korea; SVN: Slovenia; USA: United States of America.
TABLE 1. Compilation of studies investigating antiviral effects of NTP. These studies used either infectious human viruses or surrogate viruses to evaluate the antiviral effect of NTP.
UV-C germicidal irradiation, in which UV is produced in lamps by plasma (although recently can also be produced by LEDs), is a well-established decontamination technology for pathogen inactivation both in water as well as on surfaces [61–64]. However, UV-C radiation is limited by the requirement for its proximity to the target and the shadowing effect, as UV-C cannot penetrate opaque surfaces [65, 66]. Because plasma can flow around complex 3D surfaces, decontamination by plasma technology is not limited by shadowing. A recent study compared UV-C with plasma-based inactivation of feline calicivirus (FCV) on surfaces and showed that the energy cost of plasma-based inactivation can be similar to UV-C [67]. However, UV-C was effective both on dry and humid surfaces, while plasma was shown to be less effective on dry substrates [67].
Other emerging non-thermal decontamination technologies include pulsed electric fields (PEF) and high hydrostatic pressure (HHP) [68]. Although PEF has been commonly used for destruction of several non-sporing bacterial pathogens [69–72], its effect on viruses has not been well investigated [73–75]. While PEF might have advantages over NTP for specific foods in liquid form such as juices or milk (where oxidation of the food should be minimized), NTP seems more effective against viruses. HHP, on the other hand, has been extensively investigated for both inactivation of bacteria and virus [65, 76, 77] and is an accepted technology in the food industry around the world [78]. While HHP offers homogeneous treatment, its use is limited due to the requirement for sophisticated equipment and its impact on food structure [78]. Furthermore, HHP is limited in its throughput as a batch process, while NTP could be implemented in a continuous treatment process.
Ionizing radiation has the capability to inactivate microorganisms and is being used for food decontamination. However, its development and commercialization have suffered because of unfavorable public opinion [79]. Ionizing radiations, such as x-rays, gamma rays, or electron beams, can deliver energy as they pass through a food product. Absorption of the energy leads to the generation of free radicals, which upon reaction with pathogens, assist decontamination. While NTP technology also intrinsically produces radicals and ions, the associated energies of the energetic species are much smaller. This reduces the penetration depth of plasma-produced species significantly, and the majority of reactions of plasma-produced species are limited to the (near) surface of objects.
In addition to physical decontamination processes, many chemical intervention methods are used [80]. Due to their similarity, fresh produce washing and water treatment share many technologies. Examples of chemicals used for the purpose of decontamination include chlorine, chlorine dioxide, ozone, and hydrogen peroxide [81, 82]. Ozonation, a plasma-based technology, can be a highly effective decontamination approach for water, surfaces, and food, particular in the gas phase. However, its use leads to off-target effects in food decontamination [25, 82, 83].
While NTP-induced decontamination has similarities to chemical decontamination approaches, it can be considered a physical decontamination technology since it only requires air and electricity. Its key advantage is that the RONS are produced in situ and no external supply of chemicals is needed. Nonetheless, similarities between NTP and ozonation are obvious. The more intense interaction of NTP with the substrate being treated relative to ozonation leads to a broader range of species that have been shown to enhance virucidal efficacy.
Although virucidal activity of NTP has been thoroughly established, the underlying pathway leading to virus inactivation is not clear because of the inherent complexity of NTP. While NTP is also an effective source of UV radiation and electric fields, there is growing consensus that RONS are the key enablers of atmospheric pressure NTP virucidal activity, as in the case of plasma-based bacterial inactivation [84–87]. Plasma-produced RONS, including O3, O2 (a), O, OH, HO2/O2−, NO, NO2−, ONOOH/ONOO−, H2O2, HNO2, and N2O5, are proposed as key agents for pathogen inactivation [60, 85–93]. A schematic of these key species with reaction pathways in a gas phase plasma impinging on a liquid surface is shown in Figure 5. Different plasma sources are used by different researchers to investigate the inactivation of different viruses. Since not all plasma sources have been characterized in detail and different viruses might respond differently to NTP treatment, the reported data are difficult to compare quantitatively. We provide a summary of these results that have been obtained mainly in the last decade, draw general conclusions when possible, and outline some remaining important research questions.
FIGURE 5. Schematic of key plasma-produced species relevant to virus inactivation. Reaction pathways are also provided both in the gas phase and liquid phase. In the liquid phase, we also show the production of radicals from long-lived plasma produced chemistry. The horizontal dashed line represents the plasma-liquid interface. This set of reactions is a compilation of the reactions that are believed to be dominant for virus inactivation and is adapted from [59, 94, 184, 238, 239].
Plasmas can be generated with different electrode geometries, plasma power, excitation voltages, dominant reactive species, gas temperatures, feed gases, and gas residence times, all of which alter the plasma impact on virus. In addition to the changes in plasma properties, the target virus, and target substrate (surfaces, solutions, or tissues) could be of key importance for virus inactivation. A schematic of the most common plasma sources used for virus inactivation, including different treatment modalities, is shown in Figure 6. The majority of the reported studies have been performed by three types of plasma sources: plasma jets, dielectric barrier discharges (DBDs), and gliding arcs [94–96]. Plasma jets, one of the most commonly used plasma sources in decontamination studies, are typically generated in a capillary and the plasma and/or reactive species are convectively transported to the substrate being treated [97]. Plasma jets are often operated in a noble gas (Ar or He) with different molecular admixtures (e.g., O2, H2O and N2) and are point sources that facilitate topical treatment. Nonetheless, plasma jets have high virucidal activity, most likely due to the efficient production of short-lived reactive species such as O2(a) and their longer lifetime in a noble gas compared to a humid air environment. However, comparison of results from different research groups becomes rather complex because they often use in-house built plasma jets of different designs. While the majority of plasma jets have the same operating principles, differences in RONS production are to be expected.
FIGURE 6. Schematic of different plasma sources used for virus inactivation including different treatment modalities: (A) DBD for direct treatment, (B) plasma jet in contact with a substrate, (C) plasma jet (remote treatment), (D) indirect treatment (PAL/PAM), (E) volumetric DBD (remote treatment), (F) gliding arc (remote treatment), (G) two-dimensional array of integrated coaxial microhollow DBD (remote treatment), and (H) surface DBD (remote treatment). Substrate treated by each of these plasma sources could either be a surface or a liquid volume. The reactive species provided are the dominant species produced in dry air or O2-containing plasmas. Additional species like HNO2 and H2O2 can be formed for dry substrates when humidity is added to the feed gas.
Different types of DBDs—a plasma discharge generated between two metal electrodes covered by a dielectric material [98]—have been investigated for decontamination applications. The key advantage of DBDs is that they enable the production of near room temperature plasma in air through the generation of self-limiting micro-discharges. DBDs are generated by time varying voltage excitation approaches with varying geometries. Examples include nanosecond-pulsed direct DBDs (Figure 6A) [67, 95], surface DBDs (Figure 6H) [67, 96, 99], and a 2D array of integrated coaxial microhollow DBD (Figure 6G) [95, 100, 101].
Plasma sources less commonly used for virus inactivation include microwave (MW) plasma [102] and gliding arc discharge (Figure 6F) [95, 103, 104]. A key disadvantage of MW and gliding arc discharges is their elevated gas temperature, typically in excess of 1000 K [105–108], although near room temperature MW discharges similar to plasma jets have been developed for biomedical applications [109–111]. The high gas temperatures in MW and gliding arc discharges operating in air favor the production of NO, while for low gas temperatures, the production of O3 and a broader range of RONS is reported [95]. At similar plasma power, the gliding arc discharge was found to be less effective against FCV as compared to DBDs, suggesting that very high concentrations of NO, the dominant species produced in gliding arcs operating in air are needed to inactivate virus [95]. As an example, the inactivation curves of these different plasma sources and configuration against FCV treated on surfaces and in solution are shown in (Figure 7A). Although different plasma sources offer uniquely different inactivation pathways, direct comparisons of different sources for identical treatment conditions suggest that DBD-based devices show significant promise for decontamination applications because of their high virucidal effects at ambient operational temperatures and their ability to be scaled up for large volume or area treatments (Figure 7A).
FIGURE 7. (A) Inactivation curves for FCV treated on surfaces and in liquid solution by different plasma sources and configuration shown in Figure 6. The data are taken from [67, 94, 95, 101, 162] and are normalized to the reference virus titer. The short dashed colored lines represent the detection limit for each of the corresponding plasma treatment. Note that the plasma power was 14.5 W for volumetric DBD, 2D-DBD and gliding arc, while a lower power was used for direct DBD (12 W), surface DBD (0.7 W) and RF plasma jet (2.5 W). The treatment of liquids is solution and volume dependent. In this case, the solution was distilled water and the volume was kept constant at 100 μL. (B) Schematic representation of the inactivation of aerosolized PRRS virus by a volumetric DBD treatment in wind tunnel test conditions at sampling time of 5 min and discharge power of 39.6 W. The graph compares the amount of infectious virus before (control) and after (plasma) treatment as obtained by the TCID50 approach with a measure of the remaining genome obtained with the RT-qPCR method. The results show a reduction of 3.5 log10 (remaining infectivity is below detection limit), while the genome remains, confirming that the majority of the inactivated virus is still collected downstream of the plasma treatment [115].
Depending on the plasma source configuration and the proximity of the virus samples from the discharge, three distinct NTP treatment modalities can be distinguished: direct, remote, and indirect treatments (Figures 6A,D,E). During direct treatment, the electrical current through the discharge flows through the virus samples, which acts as one of the electrodes. Since the virus is in direct contact with plasma, the exposure of virus to short-lived plasma-produced species, including electrons, ions, radicals, and UV, is significantly higher as compared to the other treatment modalities. During direct treatments, the virus will also be subjected to high electric fields at the plasma-substrate interface [112]. For remote treatments, the plasma is generated at a remote location relative to the sample and the plasma effluent is convectively transported to the virus samples (Figures 6C,E–H). The plasma effluent contains reactive species with a lifetime longer than the characteristic convective transport time scales determined by the gas velocity, typically leading to gas residence times on the order of milliseconds to seconds. This gas residence time is larger than the lifetime of many radical and ionic species, which are significantly depleted in the remote treatment modality. While the dominant species in the plasma effluent will often be significantly less reactive than in the plasma, remote treatment modalities have been shown to be highly effective for virus inactivation [95, 101]. Finally, the indirect treatment modality refers to the treatment of virus samples with liquid pre-treated with plasma, more commonly referred to as plasma-activated medium or liquid (PAM or PAL) (Figure 6D). While PAM/PAL has been extensively studied in the context of bacterial inactivation and for cancer treatment [113, 114] and less so for virus decontamination, similarities between virus and bacteria inactivation have been found, as expected [94].
In general, the direct treatment modality is more effective against viruses compared to remote treatment. In particular, it was found that remote DBD treatments were not effective for dry virus, while direct treatment could inactivate virus on both dry and humid substrates [67, 101]. Although complete inactivation (>4 log10 reductions) was achieved for both treatment modalities, the inactivation could be reduced from a minute to a second time scale with direct treatment [95] (Figure 7A). When aerosolized virus was sent through the plasma, the virus inactivation timescale was further reduced to 10 ms [115]. While the direct treatment modality is more effective, it does not always entail larger energy efficiency for surface decontamination expressed as energy per unit area [67]. In first order approximation, this unanticipated result is due to the potential of a remote plasma source to treat surfaces that are much larger than its cross-sectional area.
Plasma-induced inactivation of virus in solution is dependent on the effective transfer of RONS produced in the gas-phase plasma to the liquid. Short-lived species usually have a limited penetration depth (determined by their lifetimes) and will react near the plasma-liquid interface, making the reactivity transfer extremely transport limited. For example, OH radicals have penetration depths up to a few μm in water [116–118]. The solvation of neutral species into liquid water depends on its Henry’s law solubility constant. H2O2 with a large solubility constant can easily be transferred from the gas to the liquid phase while O3 and NO2 only solvate minimally into the liquid due to almost 7 orders of magnitude lower solubility constants [119]. However, enhanced mass transfer of O3 into bulk liquid by vortexing the sample has been shown to induce efficient inactivation of pathogens [101, 120]. Highly convective transport of species can be achieved by using plasma jets, which have been used extensively for virus inactivation in solution [121–127]. Kondeti et al. reported on the decomposition of crystal violet by a plasma jet and found a correlation between decomposition efficacy and liquid phase convection induced by impinging gas jets onto the liquid [128]. Virus inactivation in solution is similarly transport-limited and can be impacted by altering the transport processes, either by diffusion or convection. Note that liquid agitated by a jet airflow will lead to convection of the virus particles in the solution, which might lead to significantly different efficacies compared to treatment of bacterial biofilms or cells attached to the bottom of a well, where plasma-produced species have to be transported through a liquid layer before reaching the cells. The above transport limitations can considerably impact which species are dominant for the inactivation of virus and might explain why often longer-lived species or secondary species derived from these species might dominate virus inactivation compared to plasma-produced radicals (Figure 5).
Virus inactivation by NTP on substrates is augmented by humidity relative to dry substrates [101]. This effect has also been previously reported for virus inactivation by O3 where short duration exposure at >90% relative humidity significantly enhanced virus inactivation [64]. A water layer on the substrate acts as a solvent to facilitate biochemical reactions such as lipid peroxidation at larger water activities [129]. Hence, even for surface decontamination in the gas phase, in the presence of a liquid layer on the substrate, multiphase transport limitations might be important.
As described above, plasma-produced RONS, including O3, O2(a), O, OH, HO2/O2−, NO, NO2−, ONOOH/ONOO−, H2O2, and N2O5, are proposed as key agents for pathogen inactivation [85–87, 92, 93]. A subset of these species (O3, O2(a) and H2O2) has been studied in the context of virus inactivation for other disinfection technologies; we will review the key findings of these species before summarizing the studies of plasma produced RONS and our key conclusions.
Ozone, an ROS produced by commercial ozonizers, has been extensively studied for its potential for virus inactivation both in water and gas phase [80, 130]. At appropriate concentrations, a physiological solution with a dissolved O3/O2 mixture (ozonized saline solution) has proven to be effective against viral pathogens and has been formalized for use in hospitals in Russia and Ukraine [131–133]. Aqueous solutions of O3 were shown to inactivate poliovirus 1 (PV1), Venezuelan Equine Encephalomyelitis virus, and Hepatitis A virus (HAV) in liquid phase [134, 135]. In the gas phase, O3 is shown to have maximal virucidal efficacy against different viruses for a short period in high humidity (>90% RH) at peak concentrations of 20–25 ppm [136]. It was also effective against Norovirus and its animal surrogates, FCV, enterovirus 71 (EV71), and bacteriophages [137–140]. A large range of Ct-values (a value defined as the product of O3 concentration and contact time with virus) for different viruses have been reported, indicating that the sensitivity of different viruses to O3 can vary significantly [138, 141].
Similarly, H2O2 is a well-known disinfectant and has been extensively tested for virus inactivation. Many commercial H2O2-based decontamination systems, registered with the Environmental Protection Agency (EPA) have been tested against different viruses including FCV, adenovirus, TGEV, AIV, African swine fever virus, Hog cholera virus, Newcastle disease virus (NDV), pseudorabies virus, swine vesicular disease virus, and MS2 bacteriophage [142–145]. In all cases, complete inactivation compared to the control was achieved with exposure times ranging from 10 min to 3 h. The effectiveness of H2O2 decontamination was reduced significantly in the presence of blood for some of the viruses [144, 145]. H2O2 has been demonstrated to inactivate FCV on different surfaces such as stainless steel, plastic, glass, vinyl flooring, and ceramic tile [142], and H1N1 influenza viruses on stainless steel coupons [146]. It has also been used to decontaminate laboratory equipment with no adverse effects [144]. Aqueous solutions of H2O2 have been utilized to inactivate both RNA and DNA viruses without significant damage to virus immunogenicity, which might suggest possibilities for the development of an H2O2-based vaccine preparation platform [146, 147].
O2(a) is the key species in photodynamic therapy (PDT), which has been much used against viral infection. The scientific community has known for at least 12 years that HIV-1 can be completely destroyed with PDT. For instance, the lipid envelope of HIV-1 is cholesterol rich with an oxidizable carbonic bond (C=C), which can be oxidized using O2(a) [148]. Several studies have reported the use of PDT for inactivation of HIV-1 using different photosensitizers such as methylene blue, rose Bengal, hypocrellin, and hypericin [149, 150] as well as for HPV and HSV [151, 152]. A list of virus-mediated infectious diseases that have been treated with PDT in clinical settings is previously summarized [153]. Due to its advancement in decontamination of blood components, PDT has also been investigated for blood banking applications [154–156]. O2(a) has been used to inactivate HSV-1 and Suid herpesvirus type 1 (SHV-1) and was shown to inactivate these viruses in the presence of human plasma [157]. Numerous studies also report inactivation of MS2 bacteriophage in aqueous suspension by O2(a) [158–160], which was also confirmed by using histidine as a quencher of O2(a) [161].
Since all of these oxidants along with other reactive species are readily generated by plasmas, it is not surprising that NTP can be highly virucidal. Nonetheless, the required treatment times in plasma can be much smaller than typically used in H2O2 and O3 decontamination approaches, implying that other species contribute to the inactivation. When the role of O3 produced by NTPs was assessed, it was observed that the virus inactivation was enhanced by the presence of RNS [101]. Further studies showed that the combination of O3 and NO2 in the gas phase leads to the production of N2O5, which most likely leads to the formation of peroxynitrite (ONOO−/ONOOH) in the humid water layer on the substrate being treated [92, 95]. RNS were confirmed to be the only active agent in the case of virus inactivation in bulk liquids for the same plasma source [101]. At low pH, the importance of ONOOH produced from H2O2 and NO2− in PAW has already been shown for bacterial inactivation by air plasma [86]. A detailed study to assess the key species responsible for virus inactivation by NTPs through a scavenger study was performed by Aboubakr et al. [94]. These studies suggest that, in addition to O3, peroxynitrous acid (or so-called acidified nitrides at reduced pH [86]) and O2(a) are the dominant species responsible for FCV inactivation in solution in an oxygen plasma [94]. Similar scavenger studies and comparison with positive controls by Yamashiro et al. confirm the role of ONOO− and O2(a) in FCV inactivation with a different plasma source [162]. Guo et al. confirmed that the inactivation of bacteriophages (T4, Φ174, MS2) is similarly strongly mediated by O2(a) [99]. They further showed, through scavenger studies, that OH radicals, superoxide (O2−), and ONOO− make no significant contributions to virus inactivation, although some of these radicals might have been less abundant for the plasma conditions used. The effect of H2O2 is mostly deemed as secondary in the inactivation of FCV, potato virus Y (PVY), and adenovirus, while it is suggested that it might play a dominant role in the inactivation of RSV and influenza A virus [84].
While the aforementioned reactive species are important for virus inactivation in solution, the effect of short-lived reactive species such as O and OH radicals is more likely in airborne virus when the radicals can directly inactivate the virus or have to diffuse into a micrometer sized droplet that is similar to the reactive diffusion length of the radical [115]. While the effect of OH against NDV in plasma-activated solution [163] is suggested, other studies imply that OH had no role in virus inactivation [99, 121]. The demonstration that OH radicals can decompose 50% of the hydrocarbons in a droplet of 60 µm [117] suggests that a similar effect could be anticipated for virus in small droplets or virus particles present in the plasma. Although the role of plasma generated O radicals on virus inactivation has not been reported to date, several studies report plasma conditions that enable the inactivation of Penicillium digitatum spores [164], cancer (THP-1 leukemia) cells [165], bacteria (E. coli) [166], and yeast cells (Saccharomyces cerevisiae) [167] by O radicals. In addition, O atoms react with Cl− in saline solution forming hypochlorite ion (OCl−) at concentrations sufficient to inactive bacteria (Pseudomonas aeruginosa and Staphylococcus aureus) [168]. Similarly, the possible role of HO2/O2−, although well established as bioactive molecules [169, 170], needs to be further established for virus inactivation.
Depending on the treatment mode, conditions and plasma source, the virus inactivation pathways could be through ROS, RNS, or a combination of both. The species that are ultimately responsible for the inactivation of the virus might not only depend on the dominant species produced by the plasmas but also on the environmental and treatment conditions, since transport limitations are exceedingly important for plasma-based decontamination. This might unfortunately add some system- and treatment-specific aspects to the inactivation mechanisms of virus by NTP.
The biological effects of plasma produced RONS on the ability of the virus to bind with host receptors, enter into host cells, and replicate its genome have only partly been quantified to date. Plasma has many different species and hence a variety of mechanisms are to be expected. One of the earlier studies showed that plasma significantly reduced the infectivity as well as the replication ability of human adenovirus [96]. Recent work has also shown that plasma can degrade purified SARS‐CoV‐2 RNA, as well as modify outer components of the virus necessary for attachment such as the spike protein [92, 171, 172]. In addition, several other investigators reported that plasma leads to significant protein oxidation of the virus coat or capsid. Yasuda et al. [173], reported that the inactivation of bacteriophage lambda by NTP was mainly due to oxidation of the coat protein; the plasma impact on the DNA did not significantly contribute to bacteriophage inactivation.
Similarly, Aboubakr et al. showed that, for short treatment times, seemingly intact viruses were still present, as observed by TEM [94, 174]. A proteomics study of the virus showed significant oxidation of the virus capsid and specific amino acids responsible for attachment and entry of the virus into the host cell. Singlet oxygen, a dominant species in the NTP used, was independently reported to inactivate viruses by damaging nucleic acid and the viral envelope [175]. The inactivation of bacteriophage f2 by ozone treatment was similarly attributed to the breakdown of viral coat proteins, which hindered the adsorption of the phage to the host cells [176]. However, other studies have shown that the impact of ozone is mainly on virus genome [177]. Even when the ROS are known, the mechanism can depend significantly on the type of virus investigated or the treatment condition used, as was the case for UV described above.
Not all studies suggest that plasma impacts the capsid or viral coat. Plasma has been shown to impact both the virus surface proteins and RNA genes of bacteriophage MS2 [178]. Sakudo et al. [179] report that a nitrogen plasma inactivated adenovirus by damaging its genomic DNA with limited impact on the capsid protein. The results show that the mechanisms might be both virus- and plasma source-specific. A detailed comparison of the plasma impact on different viruses for the same plasma conditions might shed further light on the mechanisms.
The evaluation of the efficacy of plasma-induced inactivation of pathogenic human viruses can entail significant health hazards, mandating the use of specialized laboratories such as a biosafety level 3 (BSL-3) facility. Such resources are expensive, require specialized training, and do not always justify extensive testing with these viruses. As such, surrogate animal viruses compatible with BSL-1 and BSL-2 laboratories have been favored for initial testing and plasma technology optimization [92, 101, 104, 172, 180, 181].
Animal viruses are also considered good models for airborne human viruses [182, 183]. However, due to the increased risk for testing airborne virus compared to substrates or solutions spiked with virus, bacteriophages are often used as surrogates for airborne pathogenic viruses. They are not only easier to handle but can also be produced in large quantities and quantified by plaque-based infectivity assays [184–186].
While care should be taken in translating established surrogates from one disinfection technology to the other or from one plasma source to another, the reported results on a variety of viruses, further supported by our yet unpublished results, suggest that plasma can be effective against a large range of investigated pathogens and its decontamination efficacy is, in first approximation, largely independent of the virus being treated [180]. While further research in this area is needed to assess whether the effectiveness of NTP against different virus can be impacted by plasma conditions, the complex mixture of reactive species produced by NTP might indeed overcome some of the virus-specific differences observed with other non-thermal technologies, such as O3 or UV, which might be favored because of specific chemical compositions of the RNA, DNA, or proteins in the virus capsid and coating.
Pathogenic viruses can be spread in liquids, by contact with contaminated surfaces, and through airborne routes of dissemination. Each avenue of transmission poses unique challenges with respect to disinfection by NTP.
The interaction of NTP with liquids produces reactive species in the liquid phase which are critical for many chemical and biological applications. Liquids in contact with NTP are rich sources of OH radicals and are in fact considered a form of advanced oxidation technology enabling the breakdown of organic and inorganic compounds in water [187]. More recently, NTP containing air interacting with water have been studied for its exceptional bactericidal effects that can last for several minutes to several days after water is treated by plasma (PAW) [188, 189]. Many studies have shown that the bactericidal effect of plasma treated solutions requires an acidic environment (typically, pH values of 3–4) [189, 190]. These conditions are attributed to reactive nitrogen species (RNS), particularly acidified nitrites, and peroxynitrite chemistry as highlighted above. In some cases, the bactericidal effect of PAW is ascribed to ROS such as O3 and specifically O2− related chemistry [120, 191]. Plasma treatment of solutions is also dependent on the composition of the solution. Treatment of virus suspensions in solutions with different compositions is shown to impact virus inactivation, particularly in the presence of pH buffers (impacting peroxynitrite chemistry), hydrocarbons (scavenging of oxidizing plasma-produced species) and saline (Cl− is a scavenger of OH and can react with plasma-produced O forming OCl−) [168]. PAM for use of cancer treatments is a nice example of how plasma generates long-lived hydrocarbon radicals that have biological impact.
The decontamination of liquids can be highly effective against bacteria [192]; there is no doubt this can be extended to its use for virus decontamination [94, 99]. Nonetheless, the efficacy of the decontamination will diminish with increasing concentrations of organic compounds. Plasma might be most effective in conditions where O3 and UV are also effective.
Both low pressure and atmospheric pressure NTP have been well established over the past 3 decades as excellent tools for decontamination of heat sensitive surfaces [109, 193]. This work was preceded by plasma-assisted decontamination devices, which used disinfectants such as H2O2 injected in the plasma [194]. Nonetheless, all these developments focused on bacteria and were, more recently, extended to wound healing and inactivation of biofilms [195, 196]. Most of these studies were performed in the context of decontamination of medical devices and other heat sensitive substrates.
Until 2015, only a handful of studies reported on the effect of NTP against viruses, including influenza viruses, adenovirus, corneal HSV-1, and MS2 bacteriophage [96, 121, 127, 197, 198]. These studies have been extended significantly; the state-of-the art shows that plasma-based decontamination of surfaces is possible using direct treatment on timescales of ∼1 s [95] and remote treatments on timescales of ∼1 min [101]. A more recent study demonstrated NTP can inactivate SARS-CoV-2 on various surfaces including plastic, cardboard, and metal in less than 180 s [199]. With regard to foodborne viruses, Bae et al. inactivated murine norovirus (MNV) and HAV on meat samples [103]. Ahlfeld et al. observed a significant reduction in human NoV GII-4 on plastic dry surfaces by exposure to air-based plasma generated by a DBD setup [200]. Lacombe et al. reported a significant decrease in the titer of TV and MNV on blueberries after exposure to air-based gliding arc-plasma [104]. The antibacterial efficacy of NTP against foodborne bacterial pathogens in vitro and on food and food packaging materials has been previously reviewed [201–203]. Nonetheless, virucidal effects of plasmas are much less studied and research on NTP treatment of foodborne viruses has only recently begun. Studies report NTP inactivation of murine NoV, hepatitis A virus, HuNoV, TV, and FCV [101, 103, 104, 121, 200]. The effect of NTP-produced oxidative species on the nutritive value, sensory quality attributes, and chemical safety of treated food has not been comprehensively investigated to date [204, 205].
Direct contact between plasma and substrate can lead to more effective inactivation of viruses compared to remote plasma treatments for which long-lived reactive oxygen and nitrogen species (RONS) in the plasma effluent enable inactivation. Nonetheless, direct plasma-substrate interactions are highly substrate dependent and are limited by substrate morphologies and composition. Hence, several researchers have focused on decontamination by remote plasmas. A recent report shows similar inactivation of norovirus and FCV on stainless steel or lettuce [180]. Inactivation of Salmonella heidelberg with the same plasma source on Romaine lettuce, chicken breast, and stainless steel showed significant differences in inactivation efficacies [180]. While the non-smooth structure of lettuce and chicken meat can contribute to the reduced inactivation efficiency, the authors suggested that the inactivation was impacted by the protein concentration present on the substrate, as evidenced by their experiments with different concentrations of bovine serum albumin deposited on the stainless surface. Hence, the reduction in inactivation efficacies on substrates could be enhanced by a competitive process of substrate and virus inactivation.
Until early 2020, only a handful of studies reported the inactivation efficacy of plasmas against airborne pathogens [115, 178, 198, 206]. With the advent of the COVID-19 pandemic, many research groups started investigating the effectiveness of NTP as a decontamination tool against virus transmission [171, 207, 208]. The use of cold oxygen plasma in a single-pass flow tunnel with a particle residence time of 0.44 s in the plasma resulted in 3.1, 2, and 2.1 log10 TCID50/ml reductions in hPIV-3, RSV and influenza virus A (H5N2) titers, respectively [198]. Wu et al. achieved more than 95% inactivation of aerosolized MS2 bacteriophage in ambient air [127]. Xia et al. reported a reduction in the infectivity of MS2 bacteriophage greater than 2.3 log10 using a packed-bed non-thermal plasma reactor at a high air flow rate of 170 slm or a particle residence time of 0.25 s [206]. Using the same packed-bed DBD reactor embedded in a test tunnel, a maximum of 1.3 log10 reduction in the titer of the aerosolized PRRS virus was achieved at a high flow rate of 400 slm [208]. Nayak et al. reported complete inactivation with a 3.5 log10 reduction in the aerosolized PRRS virus using a DBD reactor under all investigated operating conditions [115]. Figure 7B shows a summary of the results in this work. The virus aerosol residence time in most of these studies was in the order of milliseconds, which is a timescale relevant for typical HVAC conditions. This reinforces the importance of direct contact of virus aerosols with the plasma and the presence of abundant short-lived reactive species.
The studies summarized above highlight plasma as a promising technology with similar advantages as electrostatic precipitation [209], but possibly superior to filters. Plasma will directly inactivate the airborne virus with efficacies in excess of 99.9%, comparable to HEPA efficacies. The small pressure-drop across the plasma systems when mounted in air handling systems compared to HEPA filters will potentially require smaller investment of energy for air handling. In some HVAC systems, significant vibrations associated with their operation cause loss of fibers from the HEPA filters, resulting in diminished filtration efficacy. In contrast, NTP technology and virus inactivation by NTP are not affected by physical disturbances. Another advantage of NTP over HEPA filtration is that plasma inactivates infectious virus. In contrast, virus captured on HEPA filters can remain infectious for extended periods of time unless HEPA filtration is combined with UV-C or other decontamination technologies. Air treatment devices based on plasma technology are being currently used, although often in the context of odor control [210, 211]. The potential for broader implementation of NTP technology, already used in selected air handling systems, to enable reductions in virus transmission has considerable potential but requires further collaborative research and development.
Because application of NTP involves the delivery of reactive chemical species that can adversely affect the integrity and structures of nucleic acids, proteins, and lipids that are the building blocks for infectious viruses, early uses of NTP as an antiviral agent involved direct inactivation of viruses on inanimate surfaces. However, the same NTP-associated mechanisms that directly affect virus integrity and infectivity can also be used indirectly to reduce the risk of transmission and affect the course of virus-associated disease. Recent studies have paved the way for developing roles for NTP in vaccine preparation and delivery, treatment of virus-infected cells and tissues, and therapies that leverage the capacity of NTP to induce immunomodulation.
Vaccination remains the most effective antiviral strategy against many pathogenic viruses including poliovirus, HAV, and the measles virus [212]. In any vaccination strategy, vaccine preparation and delivery are both important elements in the developmental process to ensure that a vaccine is highly effective in preventing viral infection or virus-associated disease. Roles for NTP in both of these important aspects of vaccine development are under active exploration.
The essential component of any antiviral vaccine is the antigen, which is derived specifically from the virus. The antigen can take one of several forms, including peptides and whole proteins, inactivated virus preparations, and attenuated live viruses. Antigens to be delivered in vaccine preparations may also be encoded in nucleic acids (RNA or DNA). To date, few studies have explored the use of NTP to enhance the ability of antigens to generate an adaptive immune response.
NTP was demonstrated to inactivate whole viruses which were tested as antigens in vaccine formulations against two poultry viral infections in livestock, Newcastle disease virus and the avian influenza virus. The NTP-created vaccine formulation resulted in better B and T cell responses in chickens as compared to those vaccinated with the traditional formaldehyde inactivated virus [126, 213]. These enhanced immune responses may have been due to better preservation of viral antigen structures by NTP relative to formaldehyde-mediated inactivation. Hypothetically, greater preservation of the antigen structure would translate into a higher fidelity immune response through better recognition of viral antigens in their native conformations. Extrapolating on these studies, it may be possible to use NTP to prepare viral antigens with greater capacities to elicit virus-specific immune responses for pathogens and diseases that do not yet have a vaccine.
Vaccines are typically administered by intradermal, subcutaneous, subdermal, or intramuscular injection, as well as mucosal application and electroporation-assisted injection. The last method shares a common element—application of an electric field to alter cell membrane integrity and enable antigen delivery into cells—with generation and delivery of NTP [214]. This suggests that NTP may also be used for facilitated vaccine delivery. This was investigated in a murine model of vaccination, where NTP was used to aid in the intradermal delivery of an HIV-1 envelope-expressing DNA plasmid vaccine via direct application to the skin at the site of injection. Dermal application of NTP resulted in an increase in stimulated cytotoxic CD8+ T cells as compared to DNA plasmid injected without NTP application. This strongly suggested that NTP enhanced vaccine delivery and promoted an optimal immune response against the vaccine antigen that may not have been achieved due to inefficient delivery by other methods. NTP was proposed to be a better alternative to electroporation because NTP application is non-invasive. Additionally, NTP delivers active effectors (e.g., RONS) in addition to or instead of an electric field, dependent on the device used to generate NTP. These effectors, as shown in other studies, likely stimulate beneficial changes in mechanisms that underlie immune responses (immunomodulation) to vaccine antigens, thereby increasing vaccine efficacy [126, 213–215].
In considering the use of NTP as a therapy for viral infections, the spread of the virus and ensuing infection must be reconciled with the ability to deliver NTP to infected cells. Some virus infections result in localized clinical manifestations e.g., HSV-1 infection of the lip produces a herpetic lesion (i.e., cold sore), while a human papillomavirus (HPV) infection can take the form of a wart. The application of NTP to such localized lesions can be of therapeutic value. In addition, NTP treatment is projected to have a considerable margin of safety, as suggested by animal and human studies where no significant acute or long-term adverse effects of therapeutic NTP application to skin were noted [215, 216]. For infections by viruses that spread to multiple tissues, organs, and compartments (e.g., HIV-1 and SARS-CoV-2), it will be impractical to deliver NTP to all cells that harbor the virus because of the focal nature of NTP application.
The antiviral effect of focal NTP application to an HSV-1 infection was demonstrated in a model of HSV-1 herpes keratitis, a cause of blindness from HSV-1 replication in the cornea [217]. Alekseev et al., modeled this condition using both corneal epithelial cells and corneal explants from human donors in a study that PAL. Application of NTP-conditioned media caused a reduction in HSV-1 infection, as demonstrated by reduced HSV-1-mediated cytotoxicity in corneal epithelial cells, inhibition of viral replication, and a reduction in the number of infectious virus particles produced in corneal explants [197]. Importantly, this study demonstrated that NTP-mediated antiviral effects were accomplished in the absence of any cytotoxicity in the human corneas [197]. These results may be especially useful in guiding the development of topical NTP-based treatments to alleviate local viral infections and the associated pathogenesis.
The antiviral effects of NTP may also be useful in treating warts, which are lesions on the mucosa and skin caused by several strains of the human papillomavirus (HPV). Although warts are typically benign, infection by high-risk subtypes of HPV can result in malignant transformation. Warts can be painful, difficult to treat, and a continued source of infectious virus that can be spread by direct or indirect contact. The resolution of warts in patients after application of NTP suggests the potential of NTP to eliminate local human papillomavirus infections [218, 219]. Since NTP application was followed by lesion resolution over a period of 3–4 months, it is fair to speculate that wart clearance observed in these case studies could be attributed to the immediate antiviral effects of NTP. These patients suffered no immediate or long term side effects, reinforcing the safety of NTP demonstrated in many animal models of cancer [218, 219]. Furthermore, demonstrations of NTP-stimulated immunomodulation in animal models of cancer suggest roles for anti-HPV immune responses stimulated by NTP in establishing long-term clinical outcomes.
NTP was also shown to have antiviral effects against HIV-1-infected cells. To investigate the potential for NTP to affect the course of replication in HIV-1-infected cells, Amiran et al. applied NTP to either cell-free suspensions of HIV-1 prior to infection or to the HeLa cell-based HIV-1 reporter cell line after infecting them with HIV-1 [220]. While inhibition of viral replication was observed under both conditions, the doses of NTP that yielded antiviral effects were also cytotoxic, resulting in considerable losses in cell viability [220]. Potential mechanisms whereby NTP exhibits anti-HIV-1 effects were also explored by Volotskova et al., where monocyte-derived macrophages were exposed to NTP prior to infection. Pre-infection exposure to NTP caused a reduction in the macrophage cell surface markers, CD4 and CCR5, which are critical cell attachment receptors for HIV-1. Reductions in HIV-1 replication subsequent to NTP application were attributed to down regulation of cell surface HIV-1 receptors, which would prevent viral entry into the macrophages by decreasing virus fusion with the cell [221]. Additional assessments of pre-infection NTP application suggested that NTP also inhibited HIV-1 replication by affecting post-entry events in the virus replication cycle. While the results of these investigations do not provide a path toward a practical use for NTP to treat systemic HIV-1 infection, the antiviral effects observed for both HIV-1 (and HSV-1) demonstrate the potential of NTP to inhibit infection in cells infected with disparate viruses.
The focal nature of NTP would seem to preclude the use of NTP to treat viral infections that are widely disseminated in the body. However, recent investigations in plasma medicine suggest an intriguing strategy for addressing systemic viral infections with NTP that overcomes this limitation. This approach relies on the intersection of two growing areas of interest: immunotherapies effective against infectious diseases [222] and NTP-induced immunomodulation in eukaryotic cells [223, 224].
In the absence of effective antiviral drugs available to control or clear a viral infection, immunotherapies are emerging as promising alternatives. They are being investigated for their usefulness as treatments for viral infections and virus-associated diseases, particularly those characterized by dysfunction of the immune system. They typically involve the isolation of cells from infected patients, exposure of the cells ex vivo to appropriate stimulants to achieve a specific immunological outcome, and then administration of the altered cells to the patient as an immunotherapy (similar to vaccination) [225]. Successful treatment promotes development of immune responses against the virus to control infection, diminishes the symptoms associated with infection, and (perhaps) accelerates the clearance of the virus from the body. Such treatments allow control of the infection by the patient’s immune system without the need for antiviral drugs, even if virus is not fully cleared from the body.
NTP is shown to boost immune response against cancer while being well tolerated [215, 226, 227]. In these studies, the induction of immunogenic cell death (ICD), characterized by the release or display of various danger signals from tumor cells that alert innate immune cells, was a common measure of the immunomodulation attributed to NTP [215, 226, 227]. A similar immunotherapy approach for systemic viral disease involving NTP would rely on the delivery of NTP effectors to virus-infected cells ex vivo for the purpose of inducing specific immunological changes (immunomodulation) in those cells for subsequent administration to the patient. Furthermore, the successful use of NTP in combination in with other immunostimulatory agents ex vivo for enhanced therapeutic efficacy in murine cancer models suggests that similar lines of attack may be feasible in viral diseases [215, 226, 227]. The apparent role of NTP in these studies is to enhance the immunostimulatory capacity of cancer cells following NTP exposure in order to facilitate a stronger immune response in vivo when patients are immunized with their own NTP-exposed tumor cells.
This approach may be particularly beneficial for people living with HIV-1 (PLWH) where the infection can be suppressed by a lifelong regimen of antiretroviral drugs but is not cleared from the body [228]. The virus is instead maintained as a latent infection in reservoirs throughout the body. Latent viruses in these reservoirs remain unaffected by concentrations of antiretroviral drugs that effectively inhibit actively replicating virus. Specific deficiencies in HIV-1-specific immune responses further sustain the chronic HIV-1 infection in PLWH [229–231].
Given the capacity of NTP to stimulate immunomodulation in cells and tissues, we are investigating NTP as the basis of an immunotherapy for patients with well suppressed HIV-1 infections. Such a therapy would have parallels to other immune-based therapies, such as CAR T cell therapy and dendritic cell vaccination, in that an NTP-based immunotherapy for HIV-1 infection will elicit an effective immune response against the virus using the patient’s own cells. In this envisioned therapeutic strategy, latently infected T cells will be removed from the patient and exposed ex vivo to NTP which will induce beneficial immunological changes in these HIV-1-infected cells. NTP-exposed cells will then be injected back into the patient as a personalized vaccination. The personalized aspect of this approach is particularly important, since each HIV-1-infected patient harbors a unique population of viral variants (quasispecies) characterized by distinct viral genomic sequences and phenotypes. This personalized approach overcomes a major limitation of traditional vaccines, which are targeted typically to a single viral genotype and would be less effective against viral quasispecies in patients that deviate from that specific genetic sequence.
An NTP-based immunotherapy will rely on effects of NTP exposure in three different phases of the therapy. During the first phase in which patient cells are exposed ex vivo to NTP, one effect of NTP will be to increase antigenicity in latently infected cells. Along these lines, our investigations demonstrated that NTP exposure stimulated viral gene expression in a cell line model of latent HIV-1 infection [232]. The expression of viral proteins is a necessary first step in presenting viral antigens to host immune cells and generating an adaptive immune response to HIV-1.
NTP exposure of latently infected cells will also increase cellular immunogenicity, which will be an important part of the second phase of the therapy: administration of NTP-exposed cells to the patient. A vaccine product injected for the purpose of inducing an antigen-specific immune response must stimulate local responses at the injection site, including recruitment (chemotaxis) of immune cells involved in mounting adaptive immune responses and a level of inflammation that facilitates those immune responses. We have shown that in vitro application of NTP to T lymphocytes stimulates the release of pro-inflammatory cytokines and chemokines [232] that will have roles in creating an immunogenic environment at the site of injection [233].
In the final phase of the therapy, the presentation of viral antigens to cells of the immune system will result in an HIV-1-specific adaptive immune response. Our investigations into the effect of NTP exposure on epitope presentation revealed that NTP application to a human T lymphocyte cell lines altered the arrays of peptides presented on cell surface MHC class I molecules [232]. Comparisons of peptides detected on Jurkat T lymphocyte cells and the latently infected J-Lat cell line showed differences in host cell peptide display attributed to cell type and NTP exposure. Furthermore, NTP exposure of J-Lat cells appeared to alter the HIV-1-specific peptides displayed on these cells [232]. The strongly suggests that NTP exposure will alter the breadth of viral peptides presented in the surfaces of HIV-1-infected cells. A more diverse peptide pool is often desirable for maximizing the CD8+ T cell response, for diseases like HIV-1 infection that are highly dependent on CD8+ T cells for clearance [234, 235]. Hypothetically, altering and/or broadening the range of HIV-1-specific peptides through the effects of NTP exposure of cells during the ex vivo phase will result in a more effective HIV-1-targeted immune response capable of controlling reemergence of infection without the use of antiretroviral drugs.
The development of an NTP-based immunotherapy against HIV-1 still poses many challenges. For example, viral escape mutations and an inability to maintain robust B and T cell responses may reduce the long-term efficacy of the approach. This would be true for any viral infection in which escape variants can emerge. Additionally, NTP-induced immunotherapy will possibly be insufficient on its own to effect control over the infection. While NTP-based immunotherapy is hypothesized to offset deficiencies in the HIV-1-specific immune response, it may have limited capacity to reverse latent infection, which will be necessary to make infected cells “visible” to immune surveillance (as these cells, which do not express HIV-1 proteins, will appear to immune surveillance as uninfected cells). As one solution, NTP immunotherapy may be combined with a latency reversal agent (LRA), which will reactivate viral replication in latently infected cells. Cells harboring latent HIV-1 infections would then become productively infected, producing viral proteins and displaying virus-derived peptides that will be recognized and targeted by immune cells [29].
The hypothesized use of NTP-based immunotherapy against viral infections combined with animal studies that have explored the application of NTP as an immunomodulatory treatment for cancer indicate an additional use for NTP: treating infections by oncogenic viruses, or viruses capable of causing cancer [236]. In treating disease caused by oncogenic viruses, the dual challenge is to effectively mitigate the spread of the virus while simultaneously clearing the body of proliferating cancerous cells (which can also contribute to the spread of the virus). Treatments for proliferative diseases that are systemic, such as Hodgkin lymphoma caused by Epstein-Barr virus infection, will need to rely solely on immunomodulation induced in neoplastic cells that are exposed to NTP ex vivo and then injected into the patient as a form of immunotherapy. For more localized virus-associated cancers, such as pre-cancerous and cancerous cervical lesions caused by HPV-16/18 infection, NTP may have a direct effect on the replicating virus as well as an indirect effect in the form of a more effective adaptive immune response mounted against proliferating cells that form the lesions [237]. These and various other potential applications of NTP to prevent or treat viral diseases are summarized in Figure 8.
FIGURE 8. NTP application has potential roles in mitigating virus transmission, in prophylaxis of viral diseases and in treatments of certain focal and systemic viral infections. NTP may be used to prevent virus transmission through disinfection of viruses from food, surfaces/objects, and air. To prevent disease following exposure to viruses, NTP may be used to enhance antigenicity of viruses in vaccine preparations following inactivation or can be applied to skin to promote antigen presenting cell (APC) functions that are beneficial for vaccination. Treatments for various symptoms of viral diseases such as cold sores or warts can also incorporate NTP to exert antiviral effects and to modulate symptoms. Augmentation of the immune response may be accomplished through stimulation of damage associated molecular pattern (DAMP) emission from NTP-exposed cells, which promotes APC function and a subsequent adaptive immune response.
Efforts focused on the development of novel NTP-based interventions for viruses and viral infections have revealed that non-thermal plasma is an effective virucidal agent. NTP reduces virus burdens on contaminated surfaces and airborne viruses for the purposes of virus inactivation. In the course of investigations in this field, different devices have been used to generate NTP and achieve effective disinfection of viral pathogens. These highlight the promise for NTP as a broad-spectrum disinfectant.
Demonstrations of NTP-mediated virus inactivation have more recently been complemented by investigations of NTP as an antiviral agent that can be applied to prevent infection, interfere with virus replication, or indirectly impact viral infection and virus-associated disease by modifying host virus-specific immune responses. While the direct effects of NTP on virus infection and replication can be viewed as a derivation of the use of NTP for surface decontamination, the use of NTP as a basis for immunotherapies against viral infections is a novel and intriguing advancement of biomedical applications for NTP.
In developing NTP for use as an antiviral agent, numerous challenges remain to be addressed. First, NTP and the active effectors delivered during its generation can vary between devices and plasma forms, necessitating studies to determine the optimal form of plasma and device for a particular application. Second, the effectiveness of virus disinfection by NTP can be diminished by a number of external factors, including environmental conditions, the amount of virus on the contaminated surface, and the materials to be disinfected. Third, in applications involving disinfection of airborne viruses, there are challenges imposed by the need to scale up NTP application to meet the needs of large structures and the need for sufficient contact time between NTP effectors and airborne viruses in high flow air handling systems. Fourth, the focal nature of NTP contrasts with the disseminated nature of some viral infections. The application of NTP to localized manifestations of viral infections relies more on the direct effects of NTP on viral replication and infection. However, the use of NTP to treat viral pathogens that are disseminated throughout the body will rely less on the direct effect of NTP application and more on indirect NTP-mediated immunomodulation to enhance systemic antiviral immune responses. The use of NTP as the basis for immunotherapy or prophylactic vaccination against virus infections represents a challenging but high reward direction for the field of plasma medicine.
As a tool demonstrated to be effective for diverse applications, NTP provides many opportunities for developing effective strategies for preventing virus transmission, mitigating virus-associated disease, and protecting against infection through vaccination. Efforts to expand on these opportunities will require considerable collaboration between plasma scientists, virologists, and immunologists to develop NTP-based antiviral approaches for pathogens that are current global challenges as well as viral pathogens that have yet to emerge.
HM and GN contributed equally to this original article and share first authorship. VM, FK, and PB contributed equally and share senior authorship. NR contributed to research and figures. BW contributed to review and editing of the article. All authors approved the submitted version of the article.
The authors declare that the research was conducted in the absence of any commercial or financial relationships that could be construed as a potential conflict of interest.
HM, FK, and VM acknowledge the support of the Coulter Foundation and the Department of Microbiology and Immunology and the Institute for Molecular Medicine and Infectious Disease at the Drexel University College of Medicine. PB and GN acknowledge the support by the Department of Energy, Office of Fusion Energy Science by Award DE-SC0016053 and by the National Science Foundation by Award PHY 2020695.
1. Rouse BT, Sehrawat S. Immunity and Immunopathology to Viruses: what Decides the Outcome? Nat Rev Immunol (2010) 10(7):514–26. doi:10.1038/nri2802
2. Nicaise V. Crop Immunity against Viruses: Outcomes and Future Challenges. Front Plant Sci (2014) 5:660. doi:10.3389/fpls.2014.00660
3. Bosch A, Gkogka E, Le Guyader FS, Loisy-Hamon F, Lee A, van Lieshout L, et al. Foodborne Viruses: Detection, Risk Assessment, and Control Options in Food Processing. Int J Food Microbiol (2018) 285:110–28. doi:10.1016/j.ijfoodmicro.2018.06.001
4. Hamilton JJ, Turner K, Lichtenstein Cone M. COVID-19 and Public Health: Looking Back, Moving Forward, 27 (2021). p. S80–S86. doi:10.1097/phh.0000000000001299 Responding to the Pandemic: Challenges with Public Health Surveillance Systems and Development of a COVID-19 National Surveillance Case Definition to Support Case-Based Morbidity Surveillance during the Early Response J Public Health Manag Pract Suppl 1.
5. Mishra V, Seyedzenouzi G, Almohtadi A, Chowdhury T, Khashkhusha A, Axiaq A, et al. Health Inequalities during COVID-19 and Their Effects on Morbidity and Mortality. Jhl (2021) Vol. 13:19–26. doi:10.2147/jhl.s270175
6. Henderson DA. The Eradication of Smallpox - an Overview of the Past, Present, and Future. Vaccine (2011) 29(Suppl 4):D7–D9. doi:10.1016/j.vaccine.2011.06.080
7. Mayr A. [Historical Review of Smallpox, the Eradication of Smallpox and the Attenuated Smallpox MVA Vaccine]. Berl Munch Tierarztl Wochenschr (1999) 112(9):322–8.
8. Reid A. The Effects of the 1918-1919 Influenza Pandemic on Infant and Child Health in Derbyshire. Med Hist (2005) 49(1):29–54. doi:10.1017/s0025727300008279
9.World Health Organization (W.H.O). HIV/AIDS (2020). November 30, 2020. [cited 2021.; Available from: https://www.who.int/news-room/fact-sheets/detail/hiv-aids.
10. Zaba B, Whiteside A, Boerma JT. Demographic and Socioeconomic Impact of AIDS. AIDS (2004) 18(Suppl 2):S1–S7. doi:10.1097/00002030-200406002-00001
11. Čivljak R, Markotić A, Kuzman I. The Third Coronavirus Epidemic in the Third Millennium: What's Next? Croat Med J (2020) 61(1):1–4.
12.World Health Organization (W.H.O). Coronavirus (COVID-19) Dashboard (2021). March 14th, 2021. [cited 2021.; Available from: https://covid19.who.int/.
13. Firestone MJ, Lorentz AJ, Wang X, Como-Sabetti K, Vetter S, Smith K, et al. First Identified Cases of SARS-CoV-2 Variant B.1.1.7 in Minnesota — December 2020–January 2021. Morbidity and Mortality Weekly. Febrary (2021) 17:2021 ([cited 2021].
14.World Health Organization (W.H.O). Measles (2019) 2019. [cited 2021.; Available from: https://www.who.int/news-room/fact-sheets/detail/measles.
15. Bartsch SM, Lopman BA, Ozawa S, Hall AJ, Lee BY. Global Economic burden of Norovirus Gastroenteritis. PLoS One (2016) 11(4):e0151219. doi:10.1371/journal.pone.0151219
16. Luliano AD, Roguski KM, Chang HH, Muscatello DJ, Palekar R, Tempia S, et al. Estimates of Global Seasonal Influenza-Associated Respiratory Mortality: a Modelling Study. Lancet (2018) 391(10127):1285–300.
17. Johnson J, Chiu W. Structures of Virus and Virus-like Particles. Curr Opin Struct Biol (2000) 10(2):229–35. doi:10.1016/s0959-440x(00)00073-7
18. Blanco A, Abid I, Al-Otaibi N, Pérez-Rodríguez FJ, Fuentes C, Guix S, et al. Glass Wool Concentration Optimization for the Detection of Enveloped and Non-enveloped Waterborne Viruses. Food Environ Virol (2019) 11(2):184–92. doi:10.1007/s12560-019-09378-0
19. Cho JG, Dee SA. Porcine Reproductive and Respiratory Syndrome Virus. Theriogenology (2006) 66(3):655–62. doi:10.1016/j.theriogenology.2006.04.024
20. Jacobs SE, Lamson DM, St. George K, Walsh TJ. Human Rhinoviruses. Clin Microbiol Rev (2013) 26(1):135–62. doi:10.1128/cmr.00077-12
21. Macias AE, McElhaney JE, Chaves SS, Nealon J, Nunes MC, Samson SI, et al. The Disease burden of Influenza beyond Respiratory Illness. Vaccine (2020) 39:A6–A14. doi:10.1016/j.vaccine.2020.09.048
22. Setti L, Passarini F, De Gennaro G, Barbieri P, Perrone MG, Borelli M, et al. Airborne Transmission Route of COVID-19: Why 2 Meters/6 Feet of Inter-personal Distance Could Not Be Enough. Ijerph (2020) 17(8):2932. doi:10.3390/ijerph17082932
23. Irwin KK, Renzette N, Kowalik TF, Jensen JD. Antiviral Drug Resistance as an Adaptive Process. Virus Evol (2016) 2(1):vew014. doi:10.1093/ve/vew014
24. Domingo E, Holland JJ. RNA Virus Mutations and Fitness for Survival. Annu Rev Microbiol (1997) 51:151–78. doi:10.1146/annurev.micro.51.1.151
25. Parrish CR, Holmes EC, Morens DM, Park E-C, Burke DS, Calisher CH, et al. Cross-species Virus Transmission and the Emergence of New Epidemic Diseases. Mmbr (2008) 72(3):457–70. doi:10.1128/mmbr.00004-08
26. Abdoli A, Soleimanjahi H, Tavassoti Kheiri M, Jamali A, Jamaati A. Determining Influenza Virus Shedding at Different Time Points in Madin-darby Canine Kidney Cell Line. Cell J (2013) 15(2):130–5.
27. Mohammadi P, Desfarges S, Bartha I, Joos B, Zangger N, Munoz M, et al. 24 Hours in the Life of HIV-1 in a T Cell Line. Plos Pathog (2013) 9(1):e1003161. doi:10.1371/journal.ppat.1003161
28. Roizman B, Sears AE. An Inquiry into the Mechanisms of Herpes Simplex Virus Latency. Annu Rev Microbiol (1987) 41:543–71. doi:10.1146/annurev.mi.41.100187.002551
29. Ait-Ammar A, Kula A, Darcis G, Verdikt R, De Wit S, Gautier V, et al. Current Status of Latency Reversing Agents Facing the Heterogeneity of HIV-1 Cellular and Tissue Reservoirs. Front Microbiol (2019) 10:3060. doi:10.3389/fmicb.2019.03060
30. Saurabh S, Vohra C. What Should Be the Criteria for Determining Asymptomatic Status in COVID-19? QJM (2021) ((hcab002).
31. Farzadegan H, Polis MA, Wolinsky SM, Rinaldo Jr. CR, Sninsky JJ, Kwok S, et al. Loss of Human Immunodeficiency Virus Type 1 (HIV-I) Antibodies with Evidence of Viral Infection in Asymptomatic Homosexual Men. Ann Intern Med (1988) 108(6):785–90. doi:10.7326/0003-4819-108-6-785
32. Hyland P, Coulter W, Abu-Ruman I, Fulton C, O'Neill H, Coyle P, et al. Asymptomatic Shedding of HSV-1 in Patients Undergoing Oral Surgical Procedures and Attending for Noninvasive Treatment. Oral Dis (2007) 13(4):414–8. doi:10.1111/j.1601-0825.2007.01316.x
33. Tang S, Mao Y, Jones RM, Tan Q, Ji JS, Li N, et al. Aerosol Transmission of SARS-CoV-2? Evidence, Prevention and Control. Environ Int (2020) 144:106039. doi:10.1016/j.envint.2020.106039
34. Chan K-H, Sridhar S, Zhang RR, Chu H, Fung AY-F, Chan G, et al. Factors Affecting Stability and Infectivity of SARS-CoV-2. J Hosp Infect (2020) 106(2):226–31. doi:10.1016/j.jhin.2020.07.009
35. Tang JW, Bahnfleth WP, Bluyssen PM, Buonanno G, Jimenez JL, Kurnitski J, et al. Dismantling Myths on the Airborne Transmission of Severe Acute Respiratory Syndrome Coronavirus-2 (SARS-CoV-2). J Hosp Infect (2021) 110:89–96. doi:10.1016/j.jhin.2020.12.022
36. van Doremalen N, Bushmaker T, Morris DH, Holbrook MG, Gamble A, Williamson BN, et al. Aerosol and Surface Stability of SARS-CoV-2 as Compared with SARS-CoV-1. N Engl J Med (2020) 382(16):1564–7. doi:10.1056/nejmc2004973
37. Fears AC, Klimstra WB, Duprex P, Hartman A, Weaver SC, Plante KC, et al. Comparative Dynamic Aerosol Efficiencies of Three Emergent Coronaviruses and the Unusual Persistence of SARS-CoV-2 in Aerosol Suspensions. Cold Spring Harbor, NY: medRxiv [Preprint] (2020).
38. Alonso C, Goede DP, Morrison RB, Davies PR, Rovira A, Marthaler DG, et al. Evidence of Infectivity of Airborne Porcine Epidemic Diarrhea Virus and Detection of Airborne Viral RNA at Long Distances from Infected Herds. Vet Res (2014) 45:73. doi:10.1186/s13567-014-0073-z
39. Tellier R, Li Y, Cowling BJ, Tang JW. Recognition of Aerosol Transmission of Infectious Agents: a Commentary. BMC Infect Dis (2019) 19(1):101. doi:10.1186/s12879-019-3707-y
41. Lentz AK, Feezor RJ. Principles of Immunology. Nutr Clin Pract (2003) 18(6):451–60. doi:10.1177/0115426503018006451
42. Mohamed H, Esposito RA, Kutzler MA, Wigdahl B, Krebs FC, Miller V. Nonthermal Plasma as Part of a Novel Strategy for Vaccination. Plasma Process Polym (2020) 17:e2000051. doi:10.1002/ppap.202000051
43. Herzog RW, Ostrov DA. A Decorated Virus Cannot Hide. Science (2012) 338(6108):748–9. doi:10.1126/science.1230342
44. Agol VI. Cytopathic Effects: Virus-Modulated Manifestations of Innate Immunity? Trends Microbiol (2012) 20(12):570–6. doi:10.1016/j.tim.2012.09.003
45. Alcami A, Koszinowski UH. Viral Mechanisms of Immune Evasion. Trends Microbiol (2000) 8(9):410–8. doi:10.1016/s0966-842x(00)01830-8
46. Iwasaki M, Saito J, Zhao H, Sakamoto A, Hirota K, Ma D. Inflammation Triggered by SARS-CoV-2 and ACE2 Augment Drives Multiple Organ Failure of Severe COVID-19: Molecular Mechanisms and Implications. Inflammation (2021) 44(1):13–34. doi:10.1007/s10753-020-01337-3
47. Mayer BK, Yang Y, Gerrity DW, Abbaszadegan M. The Impact of Capsid Proteins on Virus Removal and Inactivation during Water Treatment Processes. Microbiol Insights (2015) 8(Suppl 2):15–28. doi:10.4137/MBI.S31441
48. Dietz L, Horve PF, Coil DA, Fretz M, Eisen JA, Wymelenberg KVD. Novel Coronavirus (COVID-19) Pandemic: Built Environment Considerations to Reduce Transmission. mSystems (2019) 5(2):e00245–20.2020
49. Pan M, Lednicky JA, Wu CY. Collection, Particle Sizing and Detection of Airborne Viruses. J Appl Microbiol (2019) 127(6):1596–611. doi:10.1111/jam.14278
50. Viscusi DJ, Bergman MS, Eimer BC, Shaffer RE. Evaluation of Five Decontamination Methods for Filtering Facepiece Respirators. Ann Occup Hyg (2009) 53(8):815–27. doi:10.1093/annhyg/mep070
51. Nardell EA, Nathavitharana RR. Airborne Spread of SARS-CoV-2 and a Potential Role for Air Disinfection. JAMA (2020) 324(2):141–2. doi:10.1001/jama.2020.7603
52. Budowsky EI, Bresler SE, Friedman EA, Zheleznova NV. Principles of Selective Inactivation of Viral Genome. I. UV-Induced Inactivation of Influenza Virus. Arch Virol (1981) 68(3-4):239–47. doi:10.1007/bf01314577
53. De Clercq E. Antiviral Drugs in Current Clinical Use. J Clin Virol (2004) 30(2):115–33. doi:10.1016/j.jcv.2004.02.009
54. Leneva IA, Roberts N, Govorkova EA, Goloubeva OG, Webster RG. The Neuraminidase Inhibitor GS4104 (Oseltamivir Phosphate) Is Efficacious against A/Hong Kong/156/97 (H5N1) and A/Hong Kong/1074/99 (H9N2) Influenza Viruses. Antiviral Res (2000) 48(2):101–15. doi:10.1016/s0166-3542(00)00123-6
55. Zepp F. Principles of Vaccine Design-Lessons from Nature. Vaccine (2010) 28(Suppl 3):C14–C24. doi:10.1016/j.vaccine.2010.07.020
56. Mocarski ES, Abenes GB, Manning WC, Sambucetti LC, Cherrington JM. Molecular Genetic Analysis of Cytomegalovirus Gene Regulation in Growth, Persistence and Latency. Curr Top Microbiol Immunol (1990) 154:47–74. doi:10.1007/978-3-642-74980-3_3
57. Oldstone MBA. Immunotherapy for Virus Infection. Curr Top Microbiol Immunol (1987) 134:211–29. doi:10.1007/978-3-642-71726-0_9
58. Hadden JW. Immunotherapy of Human Immunodeficiency Virus Infection. Trends Pharmacol Sci (1991) 12(3):107–11. doi:10.1016/0165-6147(91)90517-v
59. Gaens WV, Bogaerts A. Kinetic Modelling for an Atmospheric Pressure Argon Plasma Jet in Humid Air. J Phys D: Appl Phys (2013) 46(27):275201. doi:10.1088/0022-3727/46/27/275201
60. Reuter S, von Woedtke T, Weltmann K-D. The kINPen-A Review on Physics and Chemistry of the Atmospheric Pressure Plasma Jet and its Applications. J Phys D: Appl Phys (2018) 51(23):233001. doi:10.1088/1361-6463/aab3ad
61. Hijnen WAM, Beerendonk EF, Medema GJ. Inactivation Credit of UV Radiation for Viruses, Bacteria and Protozoan (Oo)cysts in Water: A Review. Water Res (2006) 40(1):3–22. doi:10.1016/j.watres.2005.10.030
62. de Roda Husman AM, Bijkerk P, Lodder W, van den Berg H, Pribil W, Cabaj A, et al. Calicivirus Inactivation by Nonionizing (253.7-Nanometer-Wavelength [UV]) and Ionizing (Gamma) Radiation. Aem (2004) 70(9):5089–93. doi:10.1128/aem.70.9.5089-5093.2004
63. Park GW, Linden KG, Sobsey MD. Inactivation of Murine Norovirus, Feline Calicivirus and Echovirus 12 as Surrogates for Human Norovirus (NoV) and Coliphage (F+) MS2 by Ultraviolet Light (254 Nm) and the Effect of Cell Association on UV Inactivation. Lett Appl Microbiol (2011) 52(2):162–7. doi:10.1111/j.1472-765x.2010.02982.x
64. Thurston-Enriquez JA, Haas CN, Jacangelo J, Riley K, Gerba CP. Inactivation of Feline Calicivirus and Adenovirus Type 40 by UV Radiation. Aem (2003) 69(1):577–82. doi:10.1128/aem.69.1.577-582.2003
65. Barbosa-Cánovas GV, Tapia MS, Cano MP. Novel Food Processing Technologies. 1 ed. Boca Raton, FL: CRC Press (2004).
66. Guerrero-Beltrán JA, Barbosa-Cánovas GV. Advantages and Limitations on Processing Foods by UV Light. Food Sci Tech Int (2004) 10(3):137–47.
67. Moldgy A, Aboubakr H, Nayak G, Goyal S, Bruggeman P. Comparative Evaluation of the Virucidal Effect of Remote and Direct Cold Air Plasmas with UV‐C. Plasma Process Polym (2020) 17(4):1900234. doi:10.1002/ppap.201900234
68. Ross AIV, Griffiths MW, Mittal GS, Deeth HC. Combining nonthermal technologies to control foodborne microorganisms. International journal of food microbiology. 2003 Dec 31;89(2-3): 125–38. doi:10.1016/S0168-1605(03)00161-2
69. Deeth HC, Datta N, Ross AI, Dam XT. Advances in Thermal and Non-Thermal Food Preservation. Ames, Iowa, USA: Blackwell Publishing (2007). p. 241–69. Pulsed Electric Field Technology: Effect on Milk and Fruit Juices.
70. Grahl T, Märkl H. Killing of Microorganisms by Pulsed Electric fields. Appl Microbiol Biotechnol (1996) 45:148–57. doi:10.1007/s002530050663
71. Sale AJH, Hamilton WA. Effects of High Electric fields on Micro-organisms. Biochim Biophys Acta (Bba) - Biomembranes (1968) 163(1):37–43. doi:10.1016/0005-2736(68)90030-8
72. Tewari G, Juneja VK. Advances in thermal and Non-thermal Food Preservation. Ames, IA: Blackwell Publishing (2007). p. 1–281.
73. Khadre MA, Yousef AE. Susceptibility of Human Rotavirus to Ozone, High Pressure, and Pulsed Electric Field. J Food Prot (2002) 65(9):1441–6. doi:10.4315/0362-028x-65.9.1441
74. Mizuno A, Inoue T, Yamaguchi S, Sakamoto KI, Saeki T, Matsumoto Y, et al. Conference Record of the 1990. IEEE Industry Applications Society Annual Meeting (1990). p. 713–9. Inactivation of Viruses Using Pulsed High Electric Field.
75. Todd ECD. Encyclopedia of Food Safety. Elsevier (2014). p. 221–42. doi:10.1016/b978-0-12-378612-8.00071-8 Foodborne Diseases: Overview of Biological Hazards and Foodborne Diseases.
76. Barbosa Cánovas GV, Pothakamury UR, Palou E, Swason BG. Nonthermal Preservation of Foods. New York: Marcel Dekker (1997). p. 276.
77. Govaris A, Pexara A. Inactivation of Foodborne Viruses by High-Pressure Processing (HPP). Foods (2021) 10(2):215. doi:10.3390/foods10020215
78. Pereira RN, Vicente AA. Environmental Impact of Novel thermal and Non-thermal Technologies in Food Processing. Food Res Int (2010) 43(7):1936–43. doi:10.1016/j.foodres.2009.09.013
79. Resurreccion AVA, Galvez FCF, Fletcher SM, Misra SK. Consumer Attitudes toward Irradiated Food: Results of a New Study. J Food Prot (1995) 58(2):193–6. doi:10.4315/0362-028x-58.2.193
80. Hirneisen KA, Black EP, Cascarino JL, Fino VR, Hoover DG, Kniel KE. Viral Inactivation in Foods: A Review of Traditional and Novel Food-Processing Technologies. Compr Rev Food Sci Food Saf (2010) 9(1):3–20. doi:10.1111/j.1541-4337.2009.00092.x
81.World Health Organization (W.H.O). Surface Decontamination of Fruits and Vegetables Eaten Raw : A Review (1998). [cited 2021.; Available from: https://apps.who.int/iris/handle/10665/64435.
82. Ramos B, Miller FA, Brandão TRS, Teixeira P, Silva CLM. Fresh Fruits and Vegetables-An Overview on Applied Methodologies to Improve its Quality and Safety. Innovative Food Sci Emerging Tech (2013) 20:1–15. doi:10.1016/j.ifset.2013.07.002
83. Ölmez H, Kretzschmar U. Potential Alternative Disinfection Methods for Organic Fresh-Cut Industry for Minimizing Water Consumption and Environmental Impact. LWT - Food Sci Tech (2009) 42(3):686–93. doi:10.1016/j.lwt.2008.08.001
84. Filipić A, Gutierrez-Aguirre I, Primc G, Mozetič M, Dobnik D. Cold Plasma, a new hope in the Field of Virus Inactivation. Trends Biotechnol (2020) 38(11):1278–91. doi:10.1016/j.tibtech.2020.04.003
85. Graves DB. The Emerging Role of Reactive Oxygen and Nitrogen Species in Redox Biology and Some Implications for Plasma Applications to Medicine and Biology. J Phys D: Appl Phys (2012) 45(26):263001. doi:10.1088/0022-3727/45/26/263001
86. Lukes P, Dolezalova E, Sisrova I, Clupek M. Aqueous-phase Chemistry and Bactericidal Effects from an Air Discharge Plasma in Contact with Water: Evidence for the Formation of Peroxynitrite through a Pseudo-second-order post-discharge Reaction of H2O 2 and HNO2. Plasma Sourc Sci Tech (2014) 23(1):015019. doi:10.1088/0963-0252/23/1/015019
87. Van Gils CAJ, Hofmann S, Boekema BK, Brandenburg R, Bruggeman PJ. Mechanisms of Bacterial Inactivation in the Liquid Phase Induced by a Remote RF Cold Atmospheric Pressure Plasma Jet. J Phys D: Appl Phys (2013) 46(7):175203. doi:10.1088/0022-3727/46/17/175203
88. Weltmann KD, Kindel E, von Woedtke T, Hähnel M, Stieber M, Brandenburg R. Atmospheric-pressure Plasma Sources: Prospective Tools for Plasma Medicine. Pure Appl Chem (2010) 82(6):1223–37. doi:10.1351/pac-con-09-10-35
89. Laroussi M, Lu X, Keidar M. Perspective: The Physics, Diagnostics, and Applications of Atmospheric Pressure Low Temperature Plasma Sources Used in Plasma Medicine. J Appl Phys (2017) 122(2):020901. doi:10.1063/1.4993710
90. Laroussi M, Akan T. Arc-Free Atmospheric Pressure Cold Plasma Jets: A Review. Plasma Process Polym (2007) 4(9):777–88. doi:10.1002/ppap.200700066
91. Lu X, Laroussi M, Puech V. On Atmospheric-Pressure Non-equilibrium Plasma Jets and Plasma Bullets. Plasma Sourc Sci. Technol. (2012) 21(3):034005. doi:10.1088/0963-0252/21/3/034005
92. Guo L, Yao Z, Yang L, Zhang H, Qi Y, Gou L, et al. Plasma-activated Water: An Alternative Disinfectant for S Protein Inactivation to Prevent SARS-CoV-2 Infection. Chem Eng J (2020) 127742. doi:10.1016/j.cej.2020.127742
93. Wende K, Williams P, Dalluge J, Van Gaens W, Aboubakr H, Bischof J, et al. Identification of the Biologically Active Liquid Chemistry Induced by a Nonthermal Atmospheric Pressure Plasma Jet. Biointerphases (2015) 10(2):029518. doi:10.1116/1.4919710
94. Aboubakr HA, Gangal U, Youssef MM, Goyal SM, Bruggeman PJ. Inactivation of Virus in Solution by Cold Atmospheric Pressure Plasma: Identification of Chemical Inactivation Pathways. J Phys D: Appl Phys (2016) 49(20):204001. doi:10.1088/0022-3727/49/20/204001
95. Moldgy A, Nayak G, Aboubakr HA, Goyal SM, Bruggeman PJ. Inactivation of Virus and Bacteria Using Cold Atmospheric Pressure Air Plasmas and the Role of Reactive Nitrogen Species. J Phys D: Appl Phys (2020) 53(4):434004. doi:10.1088/1361-6463/aba066
96. Zimmermann JL, Dumler K, Shimizu T, Morfill GE, Wolf A, Boxhammer V, et al. Effects of Cold Atmospheric Plasmas on Adenoviruses in Solution. J Phys D: Appl Phys (2011) 44(50):505201. doi:10.1088/0022-3727/44/50/505201
97. Schütze A, Jeong JY, Babayan SE, Jaeyoung Park fnm, Selwyn GS, Hicks RF. The Atmospheric-Pressure Plasma Jet: A Review and Comparison to Other Plasma Sources. IEEE Trans Plasma Sci (1998) 26(6):1685–94. doi:10.1109/27.747887
98. Kogelschatz U. Dielectric-barrier Discharges: Their History, Discharge Physics, and Industrial Applications. Plasma Chem Plasma Process (2003) 23:1–46. doi:10.1023/a:1022470901385
99. Guo L, Xu R, Gou L, Liu Z, Zhao Y, Liu D, et al. Mechanism of Virus Inactivation by Cold Atmospheric-Pressure Plasma and Plasma-Activated Water. Appl Environ Microbiol (2018) 84(15):e00726–18. doi:10.1128/aem.00726-18
100. Aboubakr HA, Nisar M, Nayak G, Nagaraja KV, Collins J, Bruggeman PJ, et al. Bactericidal Efficacy of a Two-Dimensional Array of Integrated, Coaxial, Microhollow, Dielectric Barrier Discharge Plasma against Salmonella enterica Serovar Heidelberg. Foodborne Pathog Dis (2020) 17(3):157–65. doi:10.1089/fpd.2019.2698
101. Nayak G, Aboubakr HA, Goyal SM, Bruggeman PJ. Reactive Species Responsible for the Inactivation of Feline Calicivirus by a Two-Dimensional Array of Integrated Coaxial Microhollow Dielectric Barrier Discharges in Air. Plasma Process Polym (2018) 15(1):1700119. doi:10.1002/ppap.201700119
102. Isbary G, Shimizu T, Zimmermann JL, Heinlin J, Al-Zaabi S, Rechfeld M, et al. Randomized Placebo-Controlled Clinical Trial Showed Cold Atmospheric Argon Plasma Relieved Acute Pain and Accelerated Healing in Herpes Zoster. Clin Plasma Med (2014) 2(2):50–5. doi:10.1016/j.cpme.2014.07.001
103. Bae S-C, Park SY, Choe W, Ha S-D. Inactivation of Murine Norovirus-1 and Hepatitis A Virus on Fresh Meats by Atmospheric Pressure Plasma Jets. Food Res Int (2015) 76:342–7. doi:10.1016/j.foodres.2015.06.039
104. Lacombe A, Niemira BA, Gurtler JB, Sites J, Boyd G, Kingsley DH, et al. Nonthermal Inactivation of Norovirus Surrogates on Blueberries Using Atmospheric Cold Plasma. Food Microbiol (2017) 63:1–5. doi:10.1016/j.fm.2016.10.030
105. Czernichowski A. Gliding Arc: Applications to Engineering and Environment Control. Pure Appl Chem (1994) 66(6):1301–10. doi:10.1351/pac199466061301
106. Fridman A, Nester S, Kennedy LA, Saveliev A, Mutaf-Yardimci O. Gliding Arc Gas Discharge. Prog Energ Combustion Sci (1999) 25(2):211–31. doi:10.1016/s0360-1285(98)00021-5
107. Kim HY, Kang SK, Park SM, Jung HY, Choi BH, Sim JY, et al. Characterization and Effects of Ar/air Microwave Plasma on Wound Healing. Plasma Process Polym (2015) 12(12):1423–34. doi:10.1002/ppap.201500017
108. Uhm HS, Hong YC, Shin DH. A Microwave Plasma Torch and its Applications. Plasma Sourc Sci. Technol. (2006) 15:S26–S34. doi:10.1088/0963-0252/15/2/s04
109. Laroussi M. Low Temperature Plasma-Based Sterilization: Overview and State-Of-The-Art. Plasma Process Polym (2005) 2(5):391–400. doi:10.1002/ppap.200400078
110. Shimizu T, Nosenko T, Morfill GE, Sato T, Schmidt HU, Urayama T. Characterization of Low-Temperature Microwave Plasma Treatment with and without UV Light for Disinfection. Plasma Process Polym (2010) 7(3-4):288–93. doi:10.1002/ppap.200900085
111. Shimizu T, Steffes B, Pompl R, Jamitzky F, Bunk W, Ramrath K, et al. Characterization of Microwave Plasma Torch for Decontamination. Plasma Process Polym (2008) 5(6):577–82. doi:10.1002/ppap.200800021
112. Pexara A, Govaris A. Foodborne Viruses and Innovative Non-thermal Food-Processing Technologies. Foods (2020) 9(11):1520. doi:10.3390/foods9111520
113. Kamgang-Youbi G, Herry J-M, Meylheuc T, Brisset J-L, Bellon-Fontaine M-N, Doubla A, et al. Microbial Inactivation Using Plasma-Activated Water Obtained by Gliding Electric Discharges. Lett Appl Microbiol (2009) 48(1):13–8. doi:10.1111/j.1472-765x.2008.02476.x
114. Zhang Q, Liang Y, Feng H, Ma R, Tian Y, Zhang J, et al. A Study of Oxidative Stress Induced by Non-thermal Plasma-Activated Water for Bacterial Damage. Appl Phys Lett (2013) 102:203701. doi:10.1063/1.4807133
115. Nayak G, Andrews AJ, Marabella I, Aboubakr HA, Goyal SM, Olson BA, et al. Rapid Inactivation of Airborne Porcine Reproductive and Respiratory Syndrome Virus Using an Atmospheric Pressure Air Plasma. Plasma Process. Polym. (2020) 17 (10): 1900269. doi:10.1002/ppap.201900269
116. Chen C, Liu DX, Liu ZC, Yang AJ, Chen HL, Shama G, et al. A Model of Plasma-Biofilm and Plasma-Tissue Interactions at Ambient Pressure. Plasma Chem Plasma Process (2014) 34:403–41. doi:10.1007/s11090-014-9545-1
117. Oinuma G, Nayak G, Du Y, Bruggeman PJ. Controlled Plasma-Droplet Interactions: A Quantitative Study of OH Transfer in Plasma-Liquid Interaction. Plasma Sourc Sci Tech (2020) 29(9):095002. doi:10.1088/1361-6595/aba988
118. Rumbach P, Bartels DM, Sankaran RM, Go DB. The Solvation of Electrons by an Atmospheric-Pressure Plasma. Nat Commun (2015) 6:7248. doi:10.1038/ncomms8248
119. Sander R. Compilation of Henry's Law Constants (Version 4.0) for Water as Solvent. Atmos Chem Phys (2015) 15(8):4399–981. doi:10.5194/acp-15-4399-2015
120. Pavlovich MJ, Chang H-W, Sakiyama Y, Clark DS, Graves DB. Ozone Correlates with Antibacterial Effects from Indirect Air Dielectric Barrier Discharge Treatment of Water. J Phys D: Appl Phys (2013) 46:145202. doi:10.1088/0022-3727/46/14/145202
121. Aboubakr HA, Williams P, Gangal U, Youssef MM, El-Sohaimy SAA, Bruggeman PJ, et al. Virucidal Effect of Cold Atmospheric Gaseous Plasma on Feline Calicivirus, a Surrogate for Human Norovirus. Appl Environ Microbiol (2015) 81(11):3612–22. doi:10.1128/aem.00054-15
122. Alshraiedeh NH, Alkawareek MY, Gorman SP, Graham WG, Gilmore BF. Atmospheric Pressure, Nonthermal Plasma Inactivation of MS2 Bacteriophage: Effect of Oxygen Concentration on Virucidal Activity. J Appl Microbiol (2013) 115(6):1420–6. doi:10.1111/jam.12331
123. Filipić A, Primc G, Zaplotnik R, Mehle N, Gutierrez-Aguirre I, Ravnikar M, et al. Cold Atmospheric Plasma as a Novel Method for Inactivation of Potato Virus Y in Water Samples. Food Environ Virol (2019) 11:220–8. doi:10.1007/s12560-019-09388-y
124. Ryan HA, Neuber J, Song S, Beebe SJ, Jiang C. 38th Annual International Conference of the IEEE Engineering in Medicine and Biology Society. EMBC). IEEE (2016). p. 537–40. Effects of a Non-thermal Plasma Needle Device on HPV-16 Positive Cervical Cancer Cell Viability In Vitro.
125. Takamatsu T, Uehara K, Sasaki Y, Hidekazu M, Matsumura Y, Iwasawa A, et al. Microbial Inactivation in the Liquid Phase Induced by Multigas Plasma Jet. PLoS One (2015) 10(8):e0135546. doi:10.1371/journal.pone.0135546
126. Wang G, Zhu R, Yang L, Wang K, Zhang Q, Su X, et al. Non-thermal Plasma for Inactivated-Vaccine Preparation. Vaccine (2016) 34(8):1126–32. doi:10.1016/j.vaccine.2015.10.099
127. Wu Y, Liang Y, Wei K, Li W, Yao M, Zhang J, et al. MS2 Virus Inactivation by Atmospheric-Pressure Cold Plasma Using Different Gas Carriers and Power Levels. Appl Environ Microbiol (2015) 81(3):996–1002. doi:10.1128/aem.03322-14
128. Kondeti VSSK, Bruggeman PJ. The Interaction of an Atmospheric Pressure Plasma Jet with Liquid Water: Dimple Dynamics and its Impact on crystal Violet Decomposition. J Phys D: Appl Phys (2021) 54:045204. doi:10.1088/1361-6463/abbeb5
129. Labuza TP. The Properties of Water in Relationship to Water Binding in Foods: a Review2. J Food Process. Preservation (1977) 1(2):167–90. doi:10.1111/j.1745-4549.1977.tb00321.x
130. Hoff JC. Inactivation of Microbial Agents by Chemical Disinfectants. Cincinnati, Ohio: U. S. Environmental Protection Agency (1986).EPA/600/2-86/067
131. Maslennikov OV, Kontorshchikova CN, Gribkova IA. The State Medical Academy of Nizhny Novgorod-Ozone Therapy in Practice Health Manual (2008). O3 Ministry of Health Service of the Russian Federation.
132. Schwartz A, Martínez Sánchez G, Sabah F. In: Madrid Declaration on Ozone Therapy. 2nd. edition. Madrid, Spain: Official document of ISCO3 (2015).
133. Shmakova IP, Nazarov EI. Methods of Application of Ozone in Medicine (Guidelines). Kiev, Ukraine: Kiev (2004).
134. Akey DH, Walton TE. Liquid-phase Study of Ozone Inactivation of Venezuelan Equine Encephalomyelitis Virus. Appl Environ Microbiol (1985) 50(4):882–6. doi:10.1128/aem.50.4.882-886.1985
135. Roy D, Wong PK, Engelbrecht RS, Chian ES. Mechanism of Enteroviral Inactivation by Ozone. Appl Environ Microbiol (1981) 41(3):718–23. doi:10.1128/aem.41.3.718-723.1981
136. Hudson JB, Sharma M, Vimalanathan S. Development of a Practical Method for Using Ozone Gas as a Virus Decontaminating Agent. Ozone: Sci Eng (2009) 31(3):216–23. doi:10.1080/01919510902747969
137. Hudson JB, Sharma M, Petric M. Inactivation of Norovirus by Ozone Gas in Conditions Relevant to Healthcare. J Hosp Infect (2007) 66(1):40–5. doi:10.1016/j.jhin.2006.12.021
138. Lin Y-C, Wu S-C. Effects of Ozone Exposure on Inactivation of Intra- and Extracellular Enterovirus 71. Antiviral Res (2006) 70(3):147–53. doi:10.1016/j.antiviral.2005.12.007
139. Tseng C, Li C. Inactivation of Surface Viruses by Gaseous Ozone. J Environ Health (2008) 70(10):56–62.
140. Tseng C-C, Li C-S. Ozone for Inactivation of Aerosolized Bacteriophages. Aerosol Sci Tech (2006) 40(9):683–9. doi:10.1080/02786820600796590
141. Thurston-Enriquez JA, Haas CN, Jacangelo J, Gerba CP. Inactivation of Enteric Adenovirus and Feline Calicivirus by Ozone. Water Res (2005) 39:3650–6. doi:10.1016/j.watres.2005.06.006
142. Bentley K, Dove BK, Parks SR, Walker JT, Bennett AM. Hydrogen Peroxide Vapour Decontamination of Surfaces Artificially Contaminated with Norovirus Surrogate Feline Calicivirus. J Hosp Infect (2012) 80(2):116–21. doi:10.1016/j.jhin.2011.10.010
143. Goyal SM, Chander Y, Yezli S, Otter JA. Evaluating the Virucidal Efficacy of Hydrogen Peroxide Vapour. J Hosp Infect (2014) 86:255–9. W.B. Saunders Ltd. doi:10.1016/j.jhin.2014.02.003
144. Heckert RA, Best M, Jordan LT, Dulac GC, Eddington DL, Sterritt WG. Efficacy of Vaporized Hydrogen Peroxide against Exotic Animal Viruses. Appl Environ Microbiol (1997) 63(10):3916–8. doi:10.1128/aem.63.10.3916-3918.1997
145. Pottage T, Richardson C, Parks S, Walker JT, Bennett AM. Evaluation of Hydrogen Peroxide Gaseous Disinfection Systems to Decontaminate Viruses. J Hosp Infect (2010) 74(1):55–61. doi:10.1016/j.jhin.2009.08.020
146. Rudnick SN, McDevitt JJ, First MW, Spengler JD. Inactivating Influenza Viruses on Surfaces Using Hydrogen Peroxide or Triethylene Glycol at Low Vapor Concentrations. Am J Infect Control (2009) 37(10):813–9. doi:10.1016/j.ajic.2009.06.007
147. Pinto AK, Richner JM, Poore EA, Patil PP, Amanna IJ, Slifka MK, et al. A Hydrogen Peroxide-Inactivated Virus Vaccine Elicits Humoral and Cellular Immunity and Protects against Lethal West Nile Virus Infection in Aged Mice. J Virol (2013) 87(4):1926–36. doi:10.1128/jvi.02903-12
148. Stief TW. Singlet Oxygen -oxidazable Lipids in the HIV Membrane, New Targets for AIDS Therapy? Med Hypotheses (2003) 60(4):575–7. doi:10.1016/s0306-9877(03)00046-x
149. Hamblin MR, Hasan T. Photodynamic Therapy: A New Antimicrobial Approach to Infectious Disease? Photochem Photobiol Sci (2004) 3(5):436–50. doi:10.1039/b311900a
150. Lenard J, Rabson A, Vanderoef R. Photodynamic Inactivation of Infectivity of Human Immunodeficiency Virus and Other Enveloped Viruses Using Hypericin and Rose Bengal: Inhibition of Fusion and Syncytia Formation. Proc Natl Acad Sci (1993) 90(1):158–62. doi:10.1073/pnas.90.1.158
151. Dai T, Huang YY, Hamblin MR. Photodynamic Therapy for Localized Infections-State of the Art. Photodiagnosis Photodynamic Ther (2009) 6(3-4):170–88. doi:10.1016/j.pdpdt.2009.10.008
152. Wainwright M. Local Treatment of Viral Disease Using Photodynamic Therapy. Int J Antimicrob Agents (2003) 21(6):510–20. doi:10.1016/s0924-8579(03)00035-9
153. Kharkwal GB, Sharma SK, Huang Y-Y, Dai T, Hamblin MR. Photodynamic Therapy for Infections: Clinical Applications. Lasers Surg Med (2011) 43(7):755–67. doi:10.1002/lsm.21080
154. Matthews J, Newman J, Sogandares-Bernal F, Judy M, Skiles H, Leveson J, et al. Photodynamic Therapy of Viral Contaminants with Potential for Blood Banking Applications. Transfusion (1988) 28(1):81–3. doi:10.1046/j.1537-2995.1988.28188127963.x
155. Mohr H, Lambrecht B, Selz A. Photodynamic Virus Inactivation of Blood Components. Immunological Invest (1995) 24(1-2):73–85. doi:10.3109/08820139509062763
156. Santus R, Grellier P, Schrével J, Mazière JC, Stoltz JF. Photodecontamination of Blood Components: Advantages and Drawbacks. Clin Hemorheol Microcirc (1998) 18(4):299–308.
157. Müller-Breitkreutz K, Mohr H, Briviba K, Sies H. Inactivation of Viruses by Chemically and Photochemically Generated Singlet Molecular Oxygen. J Photochem Photobiol B: Biol (1995) 30(1):63–70. doi:10.1016/1011-1344(95)07150-z
158. Hotze EM, Badireddy AR, Chellam S, Wiesner MR. Mechanisms of Bacteriophage Inactivation via Singlet Oxygen Generation in UV Illuminated Fullerol Suspensions. Environ Sci Technol (2009) 43(17):6639–45. doi:10.1021/es901110m
159. Kohn T, Grandbois M, McNeill K, Nelson KL. Association with Natural Organic Matter Enhances the Sunlight-Mediated Inactivation of MS2 Coliphage by Singlet Oxygen. Environ Sci Technol (2007) 41(13):4626–32. doi:10.1021/es070295h
160. Rule Wigginton K, Menin L, Montoya JP, Kohn T. Oxidation of Virus Proteins during UV254and Singlet Oxygen Mediated Inactivation. Environ Sci Technol (2010) 44(14):5437–43. doi:10.1021/es100435a
161. Kohn T, Nelson KL. Sunlight-mediated Inactivation of MS2 Coliphage via Exogenous Singlet Oxygen Produced by Sensitizers in Natural Waters. Environ Sci Technol (2007) 41(1):192–7. doi:10.1021/es061716i
162. Yamashiro R, Misawa T, Sakudo A. Key Role of Singlet Oxygen and Peroxynitrite in Viral RNA Damage during Virucidal Effect of Plasma Torch on Feline Calicivirus. Sci Rep (2018) 8(1):17947. doi:10.1038/s41598-018-36779-1
163. Su X, Tian Y, Zhou H, Li Y, Zhang Z, Jiang B, et al. Inactivation Efficacy of Nonthermal Plasma-Activated Solutions against Newcastle Disease Virus. Appl Environ Microbiol (2018) 84(9):e02836–17. doi:10.1128/aem.02836-17
164. Iseki S, Hashizume H, Jia F, Takeda K, Ishikawa K, Ohta T, et al. Inactivation ofPenicillium digitatumSpores by a High-Density Ground-State Atomic Oxygen-Radical Source Employing an Atmospheric-Pressure Plasma. Appl Phys Express (2011) 4(11):116201. doi:10.1143/apex.4.116201
165. Bekeschus S, Wende K, Hefny MM, Rödder K, Jablonowski H, Schmidt A, et al. Oxygen Atoms Are Critical in Rendering THP-1 Leukaemia Cells Susceptible to Cold Physical Plasma-Induced Apoptosis. Scientific Rep (2017) 7(2791):1–12. doi:10.1038/s41598-017-03131-y
166. Vujošević D, Cvelbar U, Repnik U, Modic M, Lazović S, ZavašNik-Bergant T, et al. Plasma Effects on the Bacteria Escherichia coli via Two Evaluation Methods. Plasma Sci Tech (2017) 19(7):075504.
167. Hashizume H, Ohta T, Hori M, Ito M. Growth Control of Saccharomyces cerevisiae through Dose of Oxygen Atoms. Appl Phys Lett (2015) 107:093701. doi:10.1063/1.4929952
168. Kondeti VSSK, Phan CQ, Wende K, Jablonowski H, Gangal U, Granick JL, et al. Long-lived and Short-Lived Reactive Species Produced by a Cold Atmospheric Pressure Plasma Jet for the Inactivation of Pseudomonas aeruginosa and Staphylococcus aureus. Free Radic Biol Med (2018) 124(20):275–87. doi:10.1016/j.freeradbiomed.2018.05.083
169. Byczkowski JZ, Gessner T. Biological Role of Superoxide Ion-Radical. Int J Biochem (1988) 20(6):569–80. doi:10.1016/0020-711x(88)90095-x
170. Van Hemmen JJ, Meuling WJA. Inactivation of Escherichia coli by Superoxide Radicals and Their Dismutation Products. Arch Biochem Biophys (1977) 182(2):743–8. doi:10.1016/0003-9861(77)90556-2
171. Bisag A, Isabelli P, Laurita R, Bucci C, Capelli F, Dirani G, et al. Cold Atmospheric Plasma Inactivation of Aerosolized Microdroplets Containing Bacteria and Purified SARS‐CoV‐2 RNA to Contrast Airborne Indoor Transmission. Plasma Process Polym (2020) 17(10):2000154. doi:10.1002/ppap.202000154
172. Attri P, Koga K, Shiratani M. Possible Impact of Plasma Oxidation on the Structure of the C-Terminal Domain of SARS-CoV-2 Spike Protein: a Computational Study. Appl Phys Express (2021) 14(2):027002. doi:10.35848/1882-0786/abd717
173. Yasuda H, Miura T, Kurita H, Takashima K, Mizuno A. Biological Evaluation of DNA Damage in Bacteriophages Inactivated by Atmospheric Pressure Cold Plasma. Plasma Process Polym (2010) 7:301–8. doi:10.1002/ppap.200900088
174. Aboubakr HA, Mor SK, Higgins L, Armien A, Youssef MM, Bruggeman PJ, et al. Cold Argon-Oxygen Plasma Species Oxidize and Disintegrate Capsid Protein of Feline Calicivirus. PLoS One (2018) 13(3):e0194618. doi:10.1371/journal.pone.0194618
175. Lim ME, Lee Y-l., Zhang Y, Chu JJH. Photodynamic Inactivation of Viruses Using Upconversion Nanoparticles. Biomaterials (2012) 33(6):1912–20. doi:10.1016/j.biomaterials.2011.11.033
176. Kim CK, Gentile DM, Sproul OJ. Mechanism of Ozone Inactivation of Bacteriophage F2. Appl Environ Microbiol (1980) 39(1):210–8. doi:10.1128/aem.39.1.210-218.1980
177. Torrey J, von Gunten U, Kohn T. Differences in Viral Disinfection Mechanisms as Revealed by Quantitative Transfection of Echovirus 11 Genomes. Appl Environ Microbiol (2019) 85(14):e00961–19. doi:10.1128/aem.00961-19
178. Wu Y, Liang Y, Wei K, Li W, Yao M, Zhang J, et al. MS2 Virus Inactivation by Atmospheric-Pressure Cold Plasma Using Different Gas Carriers and Power Levels. Appl Environ Microbiol (2015) 81(3):996–1002. doi:10.1128/aem.03322-14
179. Sakudo A, Toyokawa Y, Imanishi Y. Nitrogen Gas Plasma Generated by a Static Induction Thyristor as a Pulsed Power Supply Inactivates Adenovirus. PLoS One (2016) 11(6):e0157922. doi:10.1371/journal.pone.0157922
180. Aboubakr HA, Sampedro Parra F, Collins J, Bruggeman P, Goyal SM. Ìn Situ Inactivation of Human Norovirus GII.4 by Cold Plasma: Ethidium Monoazide (EMA)-coupled RT-qPCR Underestimates Virus Reduction and Fecal Material Suppresses Inactivation. Food Microbiol (2020) 85:103307. doi:10.1016/j.fm.2019.103307
181. Park SY, Ha S-D. Assessment of Cold Oxygen Plasma Technology for the Inactivation of Major Foodborne Viruses on Stainless Steel. J Food Eng (2018) 223:42–5. doi:10.1016/j.jfoodeng.2017.11.041
182. Cimolai N. Environmental and Decontamination Issues for Human Coronaviruses and Their Potential Surrogates. J Med Virol (2020) 92(11):2498–510. doi:10.1002/jmv.26170
183. Otter JA, Donskey C, Yezli S, Douthwaite S, Goldenberg SD, Weber DJ. Transmission of SARS and MERS Coronaviruses and Influenza Virus in Healthcare Settings: The Possible Role of Dry Surface Contamination. J Hosp Infect (2016) 92(3):235–50. doi:10.1016/j.jhin.2015.08.027
184. Gill J, Hyman P. Phage Choice, Isolation, and Preparation for Phage Therapy. Cpb (2010) 11(1):2–14. doi:10.2174/138920110790725311
185. McMinn BR, Ashbolt NJ, Korajkic A. Bacteriophages as Indicators of Faecal Pollution and Enteric Virus Removal. Lett Appl Microbiol (2017) 65(1):11–26. doi:10.1111/lam.12736
186. Verreault D, Moineau S, Duchaine C. Microbiology and Molecular Biology Reviews, 72. American Society for Microbiology (ASM) (2008). p. 413–44. doi:10.1128/mmbr.00002-08 Methods for Sampling of Airborne VirusesMmbr.
187. Locke BR, Sato M, Sunka P, Hoffmann MR, Chang J-S. Electrohydraulic Discharge and Nonthermal Plasma for Water Treatment. Ind Eng Chem Res (2006) 45(3):882–905. doi:10.1021/ie050981u
188. Ikawa S, Tani A, Nakashima Y, Kitano K. Physicochemical Properties of Bactericidal Plasma-Treated Water. J Phys D: Appl Phys (2016) 49(42):425401. doi:10.1088/0022-3727/49/42/425401
189. Traylor MJ, Pavlovich MJ, Karim S, Hait P, Sakiyama Y, Clark DS, et al. Long-term Antibacterial Efficacy of Air Plasma-Activated Water. J Phys D: Appl Phys (2011) 44(47):472001. doi:10.1088/0022-3727/44/47/472001
190. Oehmigen K, Hähnel M, Brandenburg R, Wilke C, Weltmann KD, Von Woedtke T, et al. The Role of Acidification for Antimicrobial Activity of Atmospheric Pressure Plasma in Liquids. Plasma Process Polym (2010) 7(3-4):250–7. doi:10.1002/ppap.200900077
191. Tani A, Ono Y, Fukui S, Ikawa S, Kitano K. Free Radicals Induced in Aqueous Solution by Non-contact Atmospheric-Pressure Cold Plasma. Appl Phys Lett (2012) 100:254103. doi:10.1063/1.4729889
192. Bruggeman P, Locke B. Low Temperature Plasma Technology: Methods and Applications. Boca Raton, FL: CRC Press (2013). p. 367–99. doi:10.1201/b15153-16 Assessment of Potential Applications of Plasma with Liquid Water.
193. Von Keudell A, Awakowicz P, Benedikt J, Raballand V, Yanguas-Gil A, Opretzka J, et al. Inactivation of Bacteria and Biomolecules by Low-Pressure Plasma Discharges. Plasma Process. Polym (2010) 7:327–52. doi:10.1002/ppap.200900121
194. Jacobs PT, Lin S-M. Hydrogen Peroxide Plasma Sterilization System. United States Patent (1987).
195. Haertel B, Woedtke Tv., Weltmann K-D, Lindequist U. Non-thermal Atmospheric-Pressure Plasma Possible Application in Wound Healing. Biomolecules Ther (2014) 22(6):477–90. doi:10.4062/biomolther.2014.105
196. Pei X, Lu X, Liu J, Liu D, Yang Y, Ostrikov K, et al. Inactivation of a 25.5 µmEnterococcus Faecalisbiofilm by a Room-Temperature, Battery-Operated, Handheld Air Plasma Jet. J Phys D: Appl Phys (2012) 45:165205. doi:10.1088/0022-3727/45/16/165205
197. Alekseev O, Donovan K, Limonnik V, Azizkhan-Clifford J. Nonthermal Dielectric Barrier Discharge (DBD) Plasma Suppresses Herpes Simplex Virus Type 1 (HSV-1) Replication in Corneal Epithelium. Trans Vis Sci Tech (2014) 3(2):2. doi:10.1167/tvst.3.2.2
198. Terrier O, Essere B, Yver M, Barthélémy M, Bouscambert-Duchamp M, Kurtz P, et al. Cold Oxygen Plasma Technology Efficiency against Different Airborne Respiratory Viruses. J Clin Virol (2009) 45(2):119–24. doi:10.1016/j.jcv.2009.03.017
199. Chen Z, Garcia G, Arumugaswami V, Wirz RE. Cold Atmospheric Plasma for SARS-CoV-2 Inactivation. Phys Fluids (1994) (1994) 32(11):111702. doi:10.1063/5.0031332
200. Ahlfeld B, Li Y, Boulaaba A, Binder A, Schotte U, Zimmermann JL, et al. Inactivation of a Foodborne Norovirus Outbreak Strain with Nonthermal Atmospheric Pressure Plasma. mBio (2015) 6(1):e02300–14. doi:10.1128/mbio.02300-14
201. López M, Calvo T, Prieto M, Múgica-Vidal R, Muro-Fraguas I, Alba-Elías F, et al. A Review on Non-thermal Atmospheric Plasma for Food Preservation: Mode of Action, Determinants of Effectiveness, and Applications. Front Microbiol (2019) 10:622. doi:10.3389/fmicb.2019.00622
202. Misra NN, Tiwari BK, Raghavarao KSMS, Cullen PJ. Nonthermal Plasma Inactivation of Food-Borne Pathogens. Food Eng Rev (2011) 3:159–70. doi:10.1007/s12393-011-9041-9
203. Niemira BA. Cold Plasma Decontamination of Foods. Annu Rev Food Sci Technol (2012) 3:125–42. doi:10.1146/annurev-food-022811-101132
204. Pankaj SK, Wan Z, Keener KM. Effects of Cold Plasma on Food Quality: A Review. Foods (2018) 7(1):4.
205. Schlüter O, Ehlbeck J, Hertel C, Habermeyer M, Roth A, Engel K-H, et al. Opinion on the Use of Plasma Processes for Treatment of Foods*. Mol Nutr Food Res (2013) 57(5):920–7. doi:10.1002/mnfr.201300039
206. Xia T, Kleinheksel A, Lee EM, Qiao Z, Wigginton KR, Clack HL. Inactivation of Airborne Viruses Using a Packed Bed Non-thermal Plasma Reactor. J Phys D: Appl Phys (2019) 52(25):255201. doi:10.1088/1361-6463/ab1466
207. Schiappacasse C, Peng P, Zhou N, Liu X, Zhai J, Cheng Y, et al. Inactivation of Aerosolized Newcastle Disease Virus with Non-thermal Plasma. Appl Eng Agric (2020) 36(1):55–60. doi:10.13031/aea.13699
208. Xia T, Yang M, Marabella I, Lee EM, Olson B, Zarling D, et al. Inactivation of Airborne Porcine Reproductive and Respiratory Syndrome Virus (PRRSv) by a Packed Bed Dielectric Barrier Discharge Non-thermal Plasma. J Hazard Mater (2020) 393:122266. doi:10.1016/j.jhazmat.2020.122266
209. Kettleson EM, Ramaswami B, Hogan CJ, Lee M-H, Statyukha GA, Biswas P, et al. Airborne Virus Capture and Inactivation by an Electrostatic Particle Collector. Environ Sci Technol (2009) 43(15):5940–6. doi:10.1021/es803289w
210. Okubo M, Kuroki T, Kametaka H, Yamamoto T. Odor Control Using the AC Barrier-type Plasma Reactors. IEEE Trans Ind Applicat (2001) 37:1447–55. doi:10.1109/28.952520
211. Zhang R, Yamamoto T, Bundy DS. Control of Ammonia and Odors in Animal Houses by a Ferroelectric Plasma Reactor. IEEE Trans Industry Appl (1996) 32(1):113–7.
212. Lahariya C. Vaccine Epidemiology: A Review. J Fam Med Prim Care (2016) 5(1):7–15. doi:10.4103/2249-4863.184616
213. Hongzhuan Z, Ying T, Xia S, Jinsong G, Zhenhua Z, Beiyu J, et al. Preparation of the Inactivated Newcastle Disease Vaccine by Plasma Activated Water and Evaluation of its protection Efficacy. Appl Microbiol Biotechnol (2020) 104(1):107–17. doi:10.1007/s00253-019-10106-8
214. Connolly RJ, Chapman T, Hoff AM, Kutzler MA, Jaroszeski MJ, Ugen KE. Non-contact Helium-Based Plasma for Delivery of DNA Vaccines. Hum Vaccin Immunother (2012) 8(11):1729–33. doi:10.4161/hv.21624
215. Khalili M, Daniels L, Lin A, Krebs FC, Snook AE, Bekeschus S, et al. Non-thermal Plasma-Induced Immunogenic Cell Death in Cancer. J Phys D: Appl Phys (2019) 52(42):423001. doi:10.1088/1361-6463/ab31c1
216. Busco G, Robert E, Chettouh-Hammas N, Pouvesle J-M, Grillon C. The Emerging Potential of Cold Atmospheric Plasma in Skin Biology. Free Radic Biol Med (2020) 161:290–304. doi:10.1016/j.freeradbiomed.2020.10.004
217. Thompson HW, Dupuy B, Beuerman RW, Hill JM, Rootman DS, Haruta Y. Neural Activity from the Acutely Infected HSV-1 Rabbit Cornea. Curr Eye Res (1988) 7(2):147–55. doi:10.3109/02713688808995744
218. Friedman PC, Miller V, Fridman G, Fridman A. Use of Cold Atmospheric Pressure Plasma to Treat Warts: a Potential Therapeutic Option. Clin Exp Dermatol (2019) 44(4):459–61. doi:10.1111/ced.13790
219. Friedman PC, Fridman G, Fridman A. Using Cold Plasma to Treat Warts in Children: A Case Series. Pediatr Dermatol (2020) 37(4):706–9. doi:10.1111/pde.14180
220. Amiran MR, Sepahi AA, Zabiollahi R, Ghomi H, Momen SB, Aghasadeghi MR. In Vitro assessment of Antiviral Activity of Cold Atmospheric Pressure Plasma Jet against the Human Immunodeficiency Virus (HIV). J Med Microbiol Infec Dis (2016) 4(3-4):62–6.
221. Volotskova O, Dubrovsky L, Keidar M, Bukrinsky M. Cold Atmospheric Plasma Inhibits HIV-1 Replication in Macrophages by Targeting Both the Virus and the Cells. PLoS One (2016) 11(10):e0165322. doi:10.1371/journal.pone.0165322
222. Ramamurthy D, Nundalall T, Cingo S, Mungra N, Karaan M, Naran K, et al. Recent Advances in Immunotherapies against Infectious Diseases. Immunother Adv (2020) ltaa007.
223. Mahdikia H, Saadati F, Freund E, Gaipl US, Majidzadeh-a K, Shokri B, et al. Gas Plasma Irradiation of Breast Cancers Promotes Immunogenicity, Tumor Reduction, and an Abscopal Effect In Vivo. Oncoimmunology (2020) 10(1):1859731. doi:10.1080/2162402x.2020.1859731
224. Lin A, Truong B, Patel S, Kaushik N, Choi E, Fridman G, et al. Nanosecond-pulsed DBD Plasma-Generated Reactive Oxygen Species Trigger Immunogenic Cell Death in A549 Lung Carcinoma Cells through Intracellular Oxidative Stress. Ijms (2017) 18(5):966. doi:10.3390/ijms18050966
225. Hamblin TJ. Ex Vivo activation and Retransfusion of white Blood Cells. The Immunotherapy of Cancer. Curr Stud Hematol Blood Transfus (1990)(57) 249–66. doi:10.1159/000418564
226. Dubuc A, Monsarrat P, Virard F, Merbahi N, Sarrette JP, Laurencin-Dalicieux S, et al. Use of Cold-Atmospheric Plasma in Oncology: a Concise Systematic Review. Ther Adv Med Oncol (2018) 10:1758835918786475. doi:10.1177/1758835918786475
227. Semmler ML, Bekeschus S, Schäfer M, Bernhardt T, Fischer T, Witzke K, et al. Molecular Mechanisms of the Efficacy of Cold Atmospheric Pressure Plasma (CAP) in Cancer Treatment. Cancers (2020) 12(2):269. doi:10.3390/cancers12020269
228. Volberding PD. Perspectives on the Use of Antiretroviral Drugs in the Treatment of HIV Infection. Infect Dis Clin North America (1994) 8(2):303–17. doi:10.1016/s0891-5520(20)30591-2
229. Akase IE, Musa BOP, Obiako RO, Elfulatiy AA, Mohammed AA. Immune Dysfunction in HIV: A Possible Role for Pro- and Anti-inflammatory Cytokines in HIV Staging. J Immunol Res (2017) 2017:4128398. doi:10.1155/2017/4128398
230. Cha L, Berry CM, Nolan D, Castley A, Fernandez S, French MA. Interferon-alpha, Immune Activation and Immune Dysfunction in Treated HIV Infection. Clin Trans Immunol (2014) 3(2):e10. doi:10.1038/cti.2014.1
231. Kottilil S, Shin K, Jackson JO, Reitano KN, O’Shea MA, Yang J, et al. Innate Immune Dysfunction in HIV Infection: Effect of HIV Envelope-NK Cell Interactions. J Immunol (2006) 176(2):1107–14. doi:10.4049/jimmunol.176.2.1107
232. Mohamed H, Clemen R, Freund E, Lackmann J, Wende K, Connors J, et al. Non-thermal Plasma Modulates Cellular Markers Associated with Immunogenicity in a Model of Latent HIV-1 Infection. PLoS One (2021) 16(3):e0247125. doi:10.1371/journal.pone.0247125
233. Ranieri P, Shrivastav R, Wang M, Lin A, Fridman G, Fridman AA, et al. Nanosecond-Pulsed Dielectric Barrier Discharge-Induced Antitumor Effects Propagate through Depth of Tissue via Intracellular Signaling. Plasma Med (2017) 7(3):283–97. doi:10.1615/plasmamed.2017019883
234. Collins DR, Gaiha GD, Walker BD. CD8+ T Cells in HIV Control, Cure and Prevention. Nat Rev Immunol (2020) 20(8):471–82. doi:10.1038/s41577-020-0274-9
235. Buhrman JD, Slansky JE. Improving T Cell Responses to Modified Peptides in Tumor Vaccines. Immunol Res (2013) 55(1-3):34–47. doi:10.1007/s12026-012-8348-9
236. Luo GG, Ou J-h. J. Oncogenic Viruses and Cancer. Virol Sin (2015) 30(2):83–4. doi:10.1007/s12250-015-3599-y
237. Friedman PC, Miller V, Fridman G, Fridman A. Various Cold Plasma Devices for the Treatment of Actinic Keratosis. J Eur Acad Dermatol Venereol (2018) 32(12):e445–e446. doi:10.1111/jdv.14969
238. Bauer G, Graves DB. Mechanisms of Selective Antitumor Action of Cold Atmospheric Plasma-Derived Reactive Oxygen and Nitrogen Species. Plasma Process Polym (2016) 13(12):1157–78. doi:10.1002/ppap.201600089
239. Tian W, Kushner MJ. Atmospheric Pressure Dielectric Barrier Discharges Interacting with Liquid Covered Tissue. J. Phys. D: Appl. Phys. (2014) 47 (16): 165201. doi:10.1088/0022-3727/47/16/165201
240. Burleson GR, Murray TM, Pollard M. Inactivation of Viruses and Bacteria by Ozone, with and without Sonication. Appl Microbiol (1975) 29(3):340–4. doi:10.1128/am.29.3.340-344.1975
241. Vickery K., Deva AK, Kumaradeva P, Bissett L, Cossart YE. Inactivation of duck hepatitis B virus by a hydrogen peroxide gas plasma sterilization system: laboratory and ‘in use’ testing. Journal of Hospital Infection (1999) 1999(41):317–322. doi:10.1053/jhin.1998.0516
242. Kelly-Wintenberg K., Hodge A., Montie T. C., Deleanu L., Sherman D., Reece Roth J., et al. Use of a one atmosphere uniform glow discharge plasma to kill a broad spectrum of microorganisms. Journal of Vacuum Science & Tech A: Vacuum, Surfaces, and Films (1999) 17(4):1539–1544. doi:10.1116/1.581849
243. Kelly-Wintenberg K., Sherman D. M., Tsai P. P.-Y., Gadri R. B., Karakaya F., Zhiyu Chen F., et al. Air filter sterilization using a one atmosphere uniform glow discharge plasma (the volfilter). IEEE Trans. Plasma Sci. (2000) 28:64–71. doi:10.1109/27.842866
244. Shin G.-A., Sobsey M. D. Reduction of Norwalk virus, poliovirus 1, and bacteriophage MS2 by ozone disinfection of water. Aem (2003) 69(7):3975–3978. doi:10.1128/aem.69.7.3975-3978.2003
245. Roth J.R., Nourgostar S., Bonds T.A. The One Atmosphere Uniform Glow Discharge Plasma (OAUGDP) — A platform technology for the 21st century. IEEE Transactions on Plasma Science (2007) 35(2):233–250.
246. Venezia R. A., Orrico M., Houston E., Yin S.-M., Naumova Y. Y. Lethal activity of nonthermal plasma sterilization against microorganisms. Infect. Control Hosp. Epidemiol. (2008) 29(5):430–436. doi:10.1086/588003
247. Yasuda H., Hashimoto M., Rahman M. M., Takashima K., Mizuno A. States of biological components in bacteria and bacteriophages during Inactivation by atmospheric dielectric barrier discharges. Plasma Process. Polym. (2008) 5(6):615–621. doi:10.1002/ppap.200800036
248. Kalghatgi S., Kelly CM, Cerchar E, Torabi B, Alekseev O, Fridman A, et al. Effects of non-thermal plasma on mammalian cells. PLoS One (2011) 6(1):e16270. doi:10.1371/journal.pone.0016270
249. Brun P., Brun P, Vono M, Venier P, Tarricone E, Deligianni V, et al. Disinfection of ocular cells and tissues by atmospheric-pressure cold plasma. PLoS One (2012) 7(3):e33245. doi:10.1371/journal.pone.0033245
250. Shi X.-M., Zhang G.-J., Wu X.-L., Peng Z.-Y., Zhang Z.-H., Shao X.-J., et al. Effect of Low-Temperature Plasma on Deactivation of Hepatitis B Virus. IEEE Trans. Plasma Sci. (2012) 40(10):2711–2716. doi:10.1109/tps.2012.2210567
251. Min S. C., Roh S. H., Niemira B. A., Sites J. E., Boyd G., Lacombe A. Dielectric barrier discharge atmospheric cold plasma inhibits Escherichia coli O157:H7, Salmonella, Listeria monocytogenes, and Tulane virus in romaine lettuce. International Journal of Food Microbiology (2016) 237:114–120. doi:10.1016/j.ijfoodmicro.2016.08.025
252. Sakudo A., Toyokawa Y., Imanishi Y., Murakami T. Crucial roles of reactive chemical species in modification of respiratory syncytial virus by nitrogen gas plasma. Materials Science and Engineering: C (2017) 74:131–136. doi:10.1016/j.msec.2017.02.007
253. Braga E. A., Lopes Filho G. d. J., Saad S. S. Argon plasma versus electrofulguration in the treatment of anal and perianal condylomata acuminata in patients with acquired immunodeficiency virus. Acta Cir. Bras. (2017) 32(6):482–490. doi:10.1590/s0102-865020170060000009
254. Park S. Y., Ha S.-D. Assessment of cold oxygen plasma technology for the inactivation of major foodborne viruses on stainless steel. Journal of Food Engineering (2018) 223:42–45. doi:10.1016/j.jfoodeng.2017.11.041
255. Štěpánová V., Slavicek P, Kelar J, Prasil J, Smekal M, Stupavska M, et al. Atmospheric pressure plasma treatment of agricultural seeds of cucumber (Cucumis sativus L.) and pepper (Capsicum annuum L.) with effect on reduction of diseases and germination improvement. Plasma Processes and Polymers (2018) 15(2):1700076.
256. Hanbal S. E., Takashima K., Miyashita S., Ando S., Ito K., Elsharkawy M. M., et al. Atmospheric-pressure Plasma Irradiation Can Disrupt Tobacco Mosaic Virus Particles and RNAs to Inactivate Their Infectivity. Arch Virol (2018) 163(10):2835–40. doi:10.1007/s00705-018-3909-4
257. Bunz O, Mese K, Zhang W, Piwowarczyk A, Ehrhardt A. Effect of Cold Atmospheric Plasma (CAP) on Human Adenoviruses Is Adenovirus Type-dependent. PLoS One (2018) 13(10):e0202352. doi:10.1371/journal.pone.0202352
258. Bunz O, Mese K, Funk C, Wulf M, Bailer SM, Piwowarczyk A, et al. Cold Atmospheric Plasma as Antiviral Therapy - Effect on Human Herpes Simplex Virus Type 1. J Gen Virol (2020) 101(2):208–15. doi:10.1099/jgv.0.001382
Keywords: reactive oxygen and nitrogen species, virus inactivation, disinfection, antiviral, immunotherapy, adjuvant, vaccine, SARS-CoV-2
Citation: Mohamed H, Nayak G, Rendine N, Wigdahl B, Krebs FC, Bruggeman PJ and Miller V (2021) Non-Thermal Plasma as a Novel Strategy for Treating or Preventing Viral Infection and Associated Disease. Front. Phys. 9:683118. doi: 10.3389/fphy.2021.683118
Received: 19 March 2021; Accepted: 06 May 2021;
Published: 01 June 2021.
Edited by:
Mounir Laroussi, Old Dominion University, United StatesReviewed by:
Toshiro Kaneko, Tohoku University, JapanCopyright © 2021 Mohamed, Nayak, Rendine, Wigdahl, Krebs, Bruggeman and Miller. This is an open-access article distributed under the terms of the Creative Commons Attribution License (CC BY). The use, distribution or reproduction in other forums is permitted, provided the original author(s) and the copyright owner(s) are credited and that the original publication in this journal is cited, in accordance with accepted academic practice. No use, distribution or reproduction is permitted which does not comply with these terms.
*Correspondence: Vandana Miller, dmFtNTRAZHJleGVsLmVkdQ==
†These authors have contributed equally to this work and share first authorship.
‡These authors have contributed equally to this work and share senior authorship.
Disclaimer: All claims expressed in this article are solely those of the authors and do not necessarily represent those of their affiliated organizations, or those of the publisher, the editors and the reviewers. Any product that may be evaluated in this article or claim that may be made by its manufacturer is not guaranteed or endorsed by the publisher.
Research integrity at Frontiers
Learn more about the work of our research integrity team to safeguard the quality of each article we publish.