- 1School of Information and Communication Engineering, Sichuan Provincial Engineering Research Center of Communication Technology for Intelligent IoT, University of Electronic Science and Technology of China, Chengdu, China
- 2Shenzhen Graduate School of Tsinghua University, Shenzhen, China
- 3Shenzhen Intellifusion Technologies Co., Ltd., Shenzhen, China
Metamaterials with novel properties have excited much research attention in the past several decades. Many applications have been proposed and developed for the reported metamaterials in various engineering areas. Specifically, for the resonant-type metamaterials with narrow resonance line width and strong resonance strength, the resonant frequency and strength are highly depended on the changings of meta-atom structure and/or substrate media properties induced by the environment physical or chemistry parameters varying. Therefore, physical or chemistry sensing applications for the resonant-type metamaterial units or arrays are developed in recent years. In this mini review, to help the researchers in those fields to catch up with the newly research advances, we would like to summarize the recently reported high-performance metamaterial-inspired sensing applications, especially the temperature sensing applications, based on different kinds of metamaterials. Importantly, by analyzing the advantages and disadvantages of several conventional metamaterial units, the newly proposed high quality-factor metamaterial units are discussed for high-precision sensing applications, in terms of the sensitivity and resolution. This mini review can guide researchers in the area of metamaterial-inspired sensors to find some new design routes for high-precision sensing.
Introduction
Electromagnetic metamaterials are kinds of synthetic structural materials with novel electromagnetic properties not found in nature [1]. Researchers have found very wide applications for the electromagnetic metamaterials in the fields of electromagnetism, optics, and materials sciences [1–4]. Specifically, the resonant frequency and strength of resonant-type electromagnetic metamaterials are strongly correlated with unit cell structural parameters and dielectric material properties of substrates, and those structural parameters and/or dielectric properties can be tuned by changing the environment physical and/or chemistry parameters. Therefore, new sensing technologies based on the resonant-type electromagnetic metamaterials can be developed [5–9]. For examples, the electromagnetic metamaterials can be widely used to sense as well as detect the changes of media parameters, pressure, humidity, temperature, and chemistry/biology molecules in the environments [10–12]. Comparing with the conventional sensing techniques, the metamaterial-inspired sensing has the potential advantages including the high precision, label-free, safety, and can work properly in long-distance wireless situation. The explorations of sensing mechanism, sensing technology and device engineering based on the electromagnetic metamaterials are well-developed and its application methods in environmental sensing, chemical detection, biosensing and IoT emerging technologies are widely studied in recent years [13–18]. Previously reported review works have focused on the analysis of different sensing mechanisms, sensing methods, and wide application explorations [14–18]. However, the analysis on how to further improve the sensing performance is absent. Therefore, this mini review would like to summarize the recently reported high-precision sensing applications (especially the temperature sensing) based on the advanced high-performance resonant-type electromagnetic metamaterials.
Research Progress
Overall Sensing Applications Analysis of Metamaterials
In the field of sensing applications based on the electromagnetic metamaterials, a variety of sensing technologies and design methods have been developed as mentioned above [5–13]. The sensing principle for most of the reported metamaterial-based sensors can be summarized as follows. At the resonant frequency of the electromagnetic metamaterial, a large number of electric field/magnetic field components are concentrated inside the basic unit of the electromagnetic metamaterial (meta-atom) and thus the macroscopic resonant frequency/strength characteristics of the electromagnetic metamaterial will follow the structural/material parameter changings inside the meta-atom. That means the characteristic changings of the dielectric material as well as the changes of surrounding environments will accordingly result in the changings of the resonant frequency or the resonant strength. Therefore, the external detection circuit and processing algorithm can be flexibly used to realize the changings monitoring of chemical/biological molecule types, gas concentrations, pressure, humidity and temperature. This sensing mechanism based on the metamaterials has many advantages compared to the conventional sensing techniques.
For examples, in the sensing fields of biology, chemistry, medicine, etc., traditional biosensors need to be labeled with fluorophores in the target. So the process is complicated, time consumed and expensive. Considering this problem, the researchers have developed different kinds of metamaterial-based sensors worked at optics [11, 12, 19], THz [20–26] and microwave [27–32], achieved groundbreaking realization methods and performances. Specifically, the refractive index of the medium around the meta-atom will be changed with the lesion level and chemical composition/concentration changings [33]. By detecting and analyzing the changes of the electromagnetic wave transmission/reflection amplitude at resonance or the resonant frequency caused by the corresponding refractive index changes, the lesion level and chemistry composition/concentration can be determined. In the field of hazardous gas or chemical sensing applications, researchers have also designed hydrogen concentration detectors based on nanorod-structured electromagnetic metamaterials [11] and hazardous chemical concentration detectors based on metal split ring resonator (SRR) [34]. When the used metamaterial is exposed to the hydrogen or there are different kinds and/or concentrations of hazardous chemical materials at the split of the metal SRR, the transmission/reflection characteristics (resonant frequency or strength) of the electromagnetic metamaterial will be changed. As a result, the hydrogen can be detected and analyzed accordingly and the concentration characteristics of other hazardous chemical materials can be detected as well.
In addition, the resonant frequency/strength characteristics of the electromagnetic metamaterial are not only strongly related to the properties of the dielectric material surrounding the meta-atom, but also depended on the distance between the meta-atom and the substrate dielectric material. The relative position changes of the dielectric material around the meta-atom will result in the changes of equivalent refractive index near the meta-atom. Accordingly, the researchers designed microwave and/or THz bands pressure sensor based on the electromagnetic metamaterials [33, 35]. In the field of humidity sensing applications, the humidity changes of the medium around the meta-atom can also cause the changes of equivalent refractive index of the medium [36]. Thereby, determining the humidity is achieved by detecting the resonant frequency/strength of the electromagnetic metamaterial. For example, Romero in [37] proposed a wireless capacitive sensing tag loaded with a metamaterial unit in a single-layer design. The selected metamaterial structure is the conventional SRR, which allowed the tag to be miniaturized and the sensor to be highly sensitive.
Metamaterial-Based Temperature Sensing Technology
Among the various metamaterial-based sensing applications, the temperature sensing is one of the key researches and application fields for the electromagnetic metamaterials. This is because some of the used substrate materials and/or constructed sub-wavelength structures have high temperature sensitive property. According to the sensing mechanism of resonant-type metamaterial-inspired sensors mentioned above, the temperature sensitive dielectric substrate materials and the sub-wavelength nano/micro mechanical structure with thermal expansion coefficient differences will result in the changings of resonant frequency/strength under different temperatures [38–42].
Temperature Sensing Based on Temperature-Sensitive Dielectric Inspired Metamaterials
Generally, for the electromagnetic metamaterials formed on the temperature-sensitive dielectric substrate, the resonant frequency/strength is highly related to the equivalent dielectric constant varying of such substrate induced by temperature changing. Various temperature-sensitivity dielectric substrates can be used, such as the low-temperature co-fired ceramic (LTCC) substrate, sea water, barium titanate, lithium niobate, etc. For examples, in 2010, Varadan and Ji pioneered the experimental studies for the resonant frequency/strength changing amounts of electromagnetic metamaterials based on the LTCC substrate due to the changings of the dielectric constant, electrical conductivity, and the thermal expansion of the medium during the temperature changes [43]. The results shown in this work indicated that the dielectric constant change has the main function (accounting for 84.03%).
In 2012, Ekmekci and Turhan-Sayan explored the temperature-sensing characteristics of SRR filling with sea water as the background medium [44]. For the proposed miniaturized metamaterial sensor prototype operating at X-band, a 158-MHz resonant frequency shift corresponds to a 20°C temperature change is achieved, leading to an average sensitivity level of 7.9 MHz/°C. In 2014, Zhang et al. used barium titanate (Ba0.5Sr0.5TiO3, BST) as a temperature-sensitive medium of the dielectric-type metamaterial and analyzed the temperature sensing mechanism [45]. An electric resonance characteristic with Lorentz-type dispersion of effective permittivity is seen around the resonant frequency. The relative permittivity of dielectric cut-wire is decreased with the increase in environmental temperature, hence, resulted in the blue shift of electric resonant frequency, with a calculated temperature sensitivity of 25 MHz/°C. In 2015, Karim et al. designed a closed-ring resonator (CRR) and a variety of open-ring resonators based on lithium niobate (LiNbO3) and compared their respective temperature sensitivity performances [46, 47]. This CRR structure-based sensor has a sensitivity up to 7.286 MHz/°C. At the same year, Zemouli et al. proposed a metamaterial sensor consisting of two concentric metallic rings and a thin metallic wire deposited on the surface of BaTiO3 substrate and studied the variations of the resonant frequency according to the permittivity changing under varied temperatures [40].
In 2017, Karim et al. further designed an array of CRRs embedded in a multi-layer dielectric substrate [48]. A mixture of 70 vol% Boron Nitride (BN) and 30 vol% Barium Titanate (BTO) was used as the dielectric substrate. It was observed that for a temperature change from 23 to 200°C, the change in resonant frequency is 81.75 MHz, corresponding to a temperature sensitivity of 0.462 MHz/°C. At the same year, Qiu and Liu presented a thermally tunable Fano resonator obtained by asymmetrically coupling a conductive rubber-based H-shaped split ring resonator (SRR) and a copper C-shaped SRR coated on a Teflon fiberglass slab substrate [49]. At the Fano resonance, surface current distributions are anti-symmetric since the current excited in the H-shaped conductive rubber-based SRR and the C-shaped copper SRR are opposite and almost equal in magnitude. Consequently, the electrical and magnetic fields are canceled out, resulting in a high quality factor. Therefore, with the increase in temperature, the Fano resonant frequency was slightly shifted from 11 to 10.5 GHz, and the transmission loss gradually increased as well. For more details about the high quality factor metamaterials used in the high-performance temperature sensing area will be discussed in later.
In general, the temperature-sensing technology based on temperature-sensitive dielectric substrates has the advantages of miniaturization, high flexibility and simple preparation process. However, its dielectric constant changes with temperature increase/decrease are in a very limited linear range. The inherent drawbacks such as low sensitivity and small dynamic range of this kind of metamaterial-based sensors will be limited for the practical application.
Temperature Sensing Based on the Thermal Expansion Coefficient Difference
Based the different thermal expansion coefficients of different substrates used in the metamaterials, the bending deformations due to the changes of background temperature will alter the equivalent capacitance/inductance parameters of the meta-atom, thereby causing the resonant frequency shift or resonance strength change. For examples, Thai et al. firstly loaded a cantilever arm at the open slot of the metal SRR as shown in Figure 1A-i [38, 50]. The arm consisted of two layers of heterogeneous materials with different thermal expansion coefficients. The upper layer was silicon with a smaller thermal expansion coefficient while the lower layer and the arm are all aluminum with larger thermal expansion coefficient. When the temperature is changed the cantilever will bend upwards or downwards, as a result affecting the equivalent capacitance value of the metal SRR. Figures 1A-ii,iii show the experimental transmission curve changes with different background temperatures, and a frequency shift of 800 MHz from 4.8 to 4.0 GHz can be seen.
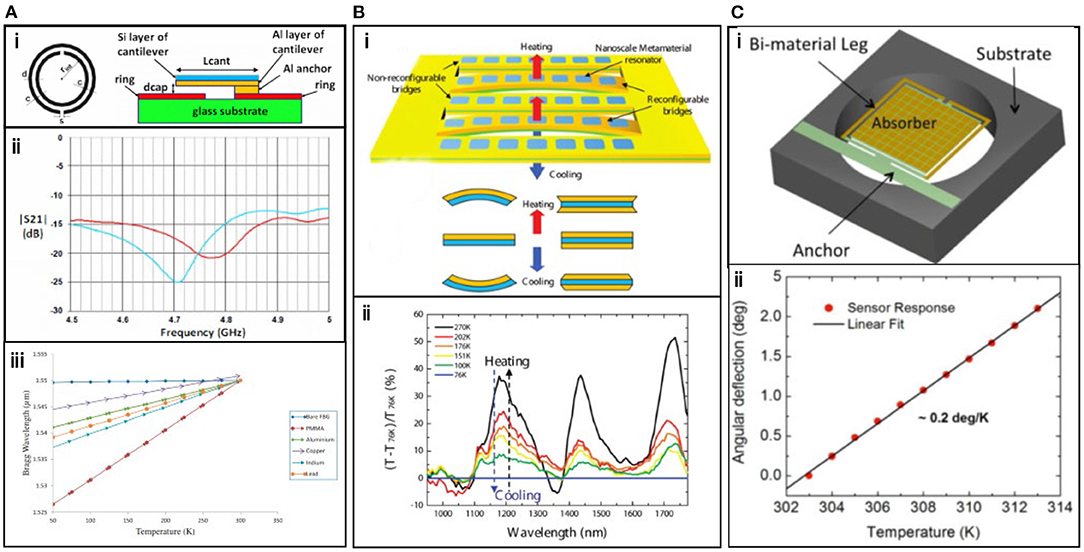
Figure 1. Temperature sensitivity metamaterial units. (A) SRR on a cantilever arm, and the transmission spectra and resonant frequency response under different temperatures [38, 50], (B) nanoscale reconfigurable photonic metamaterials and the corresponding temperature changing performances [51], (C) Metamaterial absorber configuration fabricated on a microcantilevers and the accordingly temperature changing inspired structure deformation performance [29].
Moreover, based the well-developed nano-fabrication process, temperature sensing and detection can be realized in the THz and optical frequency bands by constructing nano-scale MEMS metamaterial structures. For examples, in 2011, Ou et al. designed the nanoscale reconfigurable photonic metamaterials and the structure is shown in Figure 1B-i [51]. The Au-Si3N4-Au sandwich symmetrical structure shown in this figure has a very small deformation due to temperature change, while the two-layer structure composed of metal-semiconductor (Au-Si3N4) can show obviously deformation. The resonant properties of this system utterly depend on the coupling between neighboring bridges. For example, from Figure 1B-ii it can be seen that as the background temperature is increased, a dramatic increase of its transmission amplitude near its resonant frequency is achieved. Importantly, as the metamaterial structure was cooled back to its initial temperature these changes of its transmission spectrum were reversed.
In addition, Alves et al. constructed a MEMS temperature-sensing micro-mechanical arm on a semiconductor body and explored the temperature sensing technique in the terahertz band [29]. The sensor's absorbing element is designed with a resonant frequency that matches the source of the quantum cascade laser illumination. At the same time the semiconductor layer provides structural support, desired thermomechanical properties. As shown in Figure 1C-i, the absorbing element is connected to two Al/SiOx microcantilevers (legs), anchored to a silicon substrate, which acts as a heat sink, allowed the sensor to return to its undisturbed position when the excitation was stopped. Figure 1C-ii shows the experimental results for the temperature sensing properties, which indicates a sensitivity of 0.2 deg/°C.
Generally speaking, the temperature sensing based on the difference in thermal expansion coefficient, especially for nano-scale MEMS micro-mechanical structure, mainly worked in the optical and THz frequency bands and had the advantages of reconfigurability, miniaturization and easy to integration. However, the shape deformation due to the difference in thermal expansion coefficient of heterogeneous composites is weak. And it also has complicated preparation process, high processing difficulty and high cost.
Sensitivity Enhancement Design of Electromagnetic Metamaterial Based Sensor
The above research progresses and results demonstrated the feasibility of electromagnetic metamaterials in the field of sensing application. However, the researchers did not thoroughly study the specific technical methods to optimize and improve the sensitivity and resolution of sensing. According to the resonance type sensing theory, high sensing sensitivity and resolution require the sensing unit's resonance quality factor and the FOM (FOM is defined as the ratio of the sensitivity to the resonance 3-dB bandwidth) to be as high as possible [52, 53]. A resonant-type sensor based on the meta-atom the has a large amount of electric field/magnetic field components accumulated inside the resonance unit (e.g., at the opening gap of the SRR) during resonance. Therefore, it is an effective way to further improve the sensitivity and resolution of the resonant-type sensors by enhancing the electric field/magnetic field components of the electromagnetic metamaterial while effectively reducing the loss, to increase the resonant quality factor and improve the FOM of the sensor devices.
Based on the above suggestions, researchers have explored a variety of technical methods to improve the sensitivity of sensor, starting from the study of the resonance characteristics of new electromagnetic metamaterials. Firstly, the planar absorber composed of electromagnetic metamaterial can be equivalent to a Fabry-Perot cavity. The electric and magnetic field energy during resonance is well-bounded inside the cavity, and the radiation loss is small, resulting in the high resonant quality factor. Thus, the metamaterial absorbers can be used to improve sensing sensitivity and resolution for sensing application. For example, Cong et al. and Yahiaoui et al. studied the THz band single-frequency and multi-frequency metamaterial absorber based high-sensitivity sensors [25, 26], as shown in Figure 2A-i. It was observed from this figure that the FOM value of metamaterial absorber sensors have been found to be significantly higher than those of planar metamaterial resonators. The measured frequency shift of the sensors for different analyte thicknesses is shown in Figure 2A-ii. It was analyzed that the total frequency shift saturated at about 14.0%.
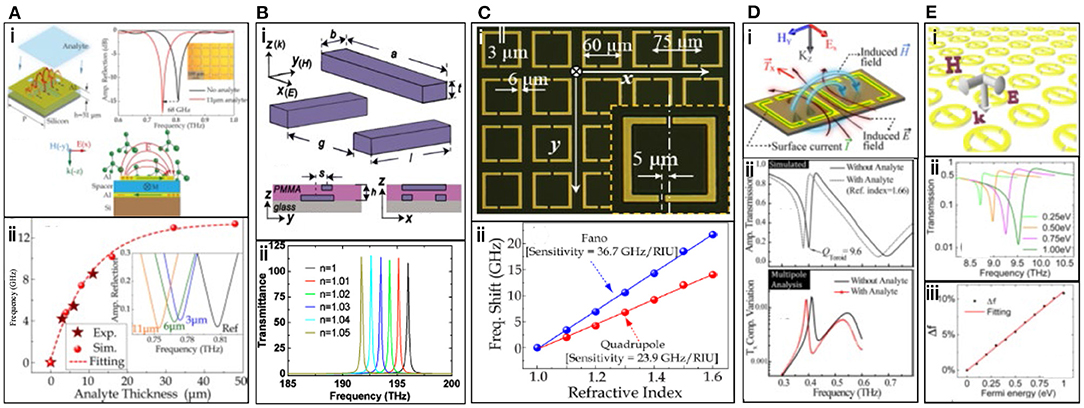
Figure 2. Performance enhanced metamaterial unit based sensors. (A) CSA and CCSA based Fabry-Perot cavity type sensors and the sensing performance [25, 26], (B) schematic illustration of EIT-like metamaterial unit and the enhanced narrow transparency windows at different refraction indexes [54], (C) microscopic image of the terahertz asymmetric Fano resonances shift with the change in refractive indexes [24], (D) operating mechanism for the toroidal resonance and the corresponding resonance changing performance [55], and (E) schematic illustration for the newly developed graphene-inspired anapole resonator and the accordingly resonance changings under different Femi levels [56].
Moreover, Dong et al. achieved an electromagnetic-induced transparency (EIT) resonance characteristics based on the interaction between different electromagnetic metamaterial units and incident electromagnetic waves [54]. They used the three-bar configuration to investigate the active plasmon analog of the EIT in order to improve the performance of the refractive-index fluctuation sensing of the surrounding medium which can be seen in Figure 2B-i. The result shown in this work (e.g., Figure 2B-ii) is higher than the traditional metamaterial resonance unit, which can be used to improve sensing sensitivity and resolution.
On another hand, Singh et al. broke the basic electromagnetic metamaterial unit structure and proposed a new Fano asymmetric resonance which can be seen in Figure 2C-i [24]. From that method they were able to achieve sensitivity levels of 7.75 × 103 nm/refractive index unit (RIU) for quadrupole and 5.7 × 104 nm/RIU with the Fano resonance. The sensitivity of Fano resonance gets enhanced due to much stronger interaction of analyte layer with the enhanced electric field in the capacitive gaps as shown in Figure 2C-ii. Semouchkina et al. also applied a full-scale electromagnetic metamaterial in a parallel version of the metal waveguide to achieve an ultra-high-Q Fano resonance characteristic, and explored the design method of a high-sensitivity sensor [57]. In addition, in 2016, Campione et al. presented a new approach that relies on a single resonator and produces robust, high quality-factor Fano resonance, by breaking the highly symmetric resonator geometries, such as cubes, to induce couplings between the orthogonal resonance modes. In particular, they designed perturbations that couple “bright” dipole modes to “dark” dipole modes whose radiative decay is suppressed by local field effects, achieving a quality-factor of ~600 [58].
For those proposed high quality-factor metamaterials, it can be used very easily in the high-precision temperature sensing area if those meta-atoms are designed on the temperature sensitivity substrates or the meta-atom is composed directly by the temperature sensitivity metals.
Discussion And Perspective
Based on the performance enhanced sensing designs by using the asymmetric high quality-factor resonance mode, in the past several years, researchers further proposed other kinds of high quality-factor metamaterial units for sensing applications, including the toroidal resonance [55, 59–61], anapole resonance [56, 62–67], and enhanced magnetic plasmon resonance [68–74]. For examples, by placing the period symmetric arrangement of the Fano resonator shown in Figure 2C-i as the mirror symmetric arrangement shown in Figure 2D, one can get the toroidal resonance with quality factor larger than the regular Lorentz resonance [63]. By concentrating the electric and magnetic field within the resonator of the new kind of anapole resonator shown in Figure 2E, the sensing resolution can be further enhanced as anticipated [67].
Conclusion
In summary, the sensing based on the electromagnetic metamaterials has developed rapidly in terms of sensing mechanism and implementation methods. Especially in the field of temperature sensing area, a variety of resonant-type temperature sensing technologies and many achievements have emerged in the past years, and researches and explorations have been carried out for further optimization and improvement of sensing sensitivity and resolution. Most of the existing temperature-sensing technologies based on temperature-sensitive dielectric materials, thermal expansion coefficient difference, and nano-scale MEMS structure are all derived from the dielectric constant, material shape, mechanical structure, etc. The sensing sensitivity improvement technologies based on high-quality-factor electromagnetic metamaterials are explored.
Author Contributions
LM, DC, WZ, JL, and YH discussed together and proposed the review paper content. LM, SZ, YH, and GW modified the review paper frame. DC, YL, and YZ finished the references collection and figure preparation. LM and DC finished the whole manuscript writing. JL, YH, and GW finished the whole manuscript modification and finalization. All authors finished the proofread.
Funding
This work was supported in part by the National Natural Science Foundation of China under Project Contracts Nos. 61701082, 61971113, and 61901095, in part by National Key R&D Program under Project Nos. 2018YFB1802102 and 2018AAA0103203, in part by Guangdong Provincial Research and Development Plan in Key Areas under Project Contract Nos. 2019B010141001 and 2019B010142001, in part by Sichuan Provincial Science and Technology Planning Program under Project Contracts Nos. 2019YFG0418, 2019YFG0120, 2020YFG0039, 2021YFG0013, and 2021YFH0133, in part by the Ministry of Education-China Mobile Fund Program under Project Contract No. MCM20180104, in part by Yibin Science and Technology Program—Key Projects under Project Contract Nos. 2018ZSF001 and 2019GY001, in part by the fundamental research funds for the Central Universities under Project Contract No. ZYGX2019Z022 and in part by Intelligent Terminsal Key Laboratory of Sichuan Province (Project Contract No. SCITLAB-0010).
Conflict of Interest
WZ was employed by company Shenzhen Intellifusion Technologies Co., Ltd.
The remaining authors declare that the research was conducted in the absence of any commercial or financial relationships that could be construed as a potential conflict of interest.
References
1. Cui TJ, Smith DR, Liu R. Metamaterials: Theory, Design and Applications. New York, NY: Spring-Verlag (2010).
2. Xu HX, Ma S, Luo W, Cai T, Sun S, He Q, et al. Aberration-free and functionality-switchable meta-lenses based on tunable metasurfaces. Appl Phys Lett. (2016) 109:193506. doi: 10.1063/1.4967438
3. Xu HX, Sun S, Tang S, Ma S, He Q, Wang GM, et al. Dynamical control on helicity of electromagnetic waves by tunable metasurfaces. Sci Rep. (2016) 6:1–10. doi: 10.1038/srep27503
4. Ren X Das R Tran P Ngo TD Xie YM Auxetic metamaterials and structures: a review. Smart Mat Struct. (2018) 27:023001. doi: 10.1088/1361-665X/aaa61c
5. Ishimaru A, Jaruwatanadilok S, Kuga Y. Generalized surface plasmon resonance sensors using metamaterials and negative index materials. Prog Electromag Res. (2005) 51:139–52. doi: 10.2528/PIER04020603
6. Driscoll T, Andreev GO, Basov DN, Palit S, Cho SY, Jokerst NM, et al. Tuned permeability in terahertz split-ring resonators for devices and sensors. Appl Phys Lett. (2007) 91:062511. doi: 10.1063/1.2768300
7. Al-Naib IAI, Jansen C, Koch M. Thin-film sensing with planar asymmetric metamaterial resonators. Appl Phys Lett. (2008) 93:083507. doi: 10.1063/1.2976636
8. Cubukcu E, Zhang S, Park YS, Bartal G, Zhang X. Split ring resonator sensors for infrared detection of single molecular monolayers. Appl Phys Lett. (2009) 95:043113. doi: 10.1063/1.3194154
9. Papasimakis N, Luo Z, Shen ZX, De Angelis F, Di Fabrizio E, Nikolaenko AE, et al. Graphene in a photonic metamaterial. Optics Express. (2010) 18:8353–9. doi: 10.1364/OE.18.008353
10. Withayachumnankul W, Jaruwongrungsee K, Fumeaux C, Abbott D. Metamaterial-inspired multichannel thin-film sensor. IEEE Sensors. (2012) 12:1455–8. doi: 10.1109/JSEN.2011.2173762
11. Lee HJ Lee JH Moon HS Jang IS Choi JS Yook JG . A planar split-ring resonator-based microwave biosensor for label-free detection of biomolecules. Sens Actuators B Chem. (2012) 169:26–31. doi: 10.1016/j.snb.2012.01.044
12. Nasir ME, Dickson W, Wurtz GA, Wardley WP, Zayats AV. Hydrogen detected by the naked eye: optical hydrogen gas sensors based on core/shell plasmonic nanorod metamaterials. Adv Mater. (2014) 26:3532–7. doi: 10.1002/adma.201305958
13. Xu X, Peng B, Li D, Zhang J, Wong LM, Zhang Q, et al. Flexible visible–infrared metamaterials and their applications in highly sensitive chemical and biological sensing. Nano Lett. (2011) 11:3232–8. doi: 10.1021/nl2014982
14. Xu W, Xie L, Ying Y. Mechanisms and applications of terahertz metamaterial sensing: a review. Nanoscale. (2017) 9:13864–78. doi: 10.1039/C7NR03824K
15. Salim A, Lim S. Review of recent metamaterial microfluidic sensors. Sensors. (2018) 18:232. doi: 10.3390/s18010232
16. Yoo SJ, Park QH. Metamaterials and chiral sensing: a review of fundamentals and applications. Nanophotonics. (2019) 8: 249–61. doi: 10.1515/nanoph-2018-0167
17. Vivek A, Shambavi K, Alex Z C. A review: metamaterial sensors for material characterization. Sensor Rev. (2019) 39:417–32. doi: 10.1108/SR-06-2018-0152
18. Bogue R. Sensing with metamaterials: a review of recent developments. Sensor Rev. (2017) 37:305–11. doi: 10.1108/SR-12-2016-0281
19. Kabashin AV, Evans P, Pastkovsky S, Hendren W, Wurtz GA, Atkinson R, et al. Plasmonic nanorod metamaterials for biosensing. Nat. (2009) 8:867–71. doi: 10.1038/nmat2546
20. Wu X, Pan X, Quan B, Xu X, Gu C, Wang L. Self-referenced sensing based on terahertz metamaterial for aqueous solutions. Appl Phys Lett. (2013) 102:151109. doi: 10.1063/1.4802236
21. Drexler C, Shishkanova TV, Lange C, Danilov SN, Weiss D, Ganichev SD, et al. Terahertz split-ring metamaterials as transducers for chemical sensors based on conducting polymers: a feasibility study with sensing of acidic and basic gases using polyaniline chemosensitive layer. Microchim Acta. (2014) 181:1857–62. doi: 10.1007/s00604-014-1263-0
22. Cheng Y, Mao XS, Wu C, Wu L, Gong R. Infrared non-planar plasmonic perfect absorber for enhanced sensitive refractive index sensing. Opt Mater. (2016) 53:195–200. doi: 10.1016/j.optmat.2016.01.053
23. Li J, Shah CM, Withayachumnankul W, Ung BS-Y, Mitchell A, Sriram S, et al. Flexible terahertz metamaterials for dual-axis strain sensing. Opt Lett. (2013) 38:2104–6. doi: 10.1364/OL.38.002104
24. Singh R, Cao W, Al-Naib I, Cong L, Withayachumnankul W, Zhang W. Ultrasensitive terahertz sensing with high-Q Fano resonances in metasurfaces. Appl Phys Lett. (2014) 105:171101. doi: 10.1063/1.4895595
25. Cong L, Tan S, Yahiaoui R, Yan F, Zhang W, Singh R. Experimental demonstration of ultrasensitive sensing with terahertz metamaterial absorbers: a comparison with the metasurfaces. Appl Phys Lett. (2015) 106:31107. doi: 10.1063/1.4906109
26. Yahiaoui R, Tan S, Cong L, Singh R, Yan F, Zhang W. Multispectral terahertz sensing with highly flexible ultrathin metamaterial absorber. J Appl Phys. (2015) 118:83103. doi: 10.1063/1.4929449
27. Alahnomi RA, Zakaria Z, Ruslan E, Ab Rashid SR, Mohd Bahar AA. High-Q sensor based on symmetrical split ring resonator with spurlines for solids material detection. IEEE Sens J. (2017) 17:2766–75. doi: 10.1109/JSEN.2017.2682266
28. Tao H, Kadlec EA, Strikwerda AC, Fan K, Padilla WJ, Averitt RD, et al. Microwave and terahertz wave sensing with metamaterials. Opt Express. (2011) 19:21620–6. doi: 10.1364/OE.19.021620
29. Alves F, Grbovic D, Kearney B, Karunasiri G. High sensitivity metamaterial based bi-material terahertz sensor. Terahertz RF Millimeter Submillimeter Wave Technol Appl VI. (2013) 8624:862411. doi: 10.1117/12.2005272
30. Melik R, Unal E, Kosku Perkgoz N, Puttlitz C, Demir HV. Flexible metamaterials for wireless strain sensing. Appl Phys Lett. (2009) 95:3–5. doi: 10.1063/1.3250175
31. Penirschke A, Schussler M, Jakoby R. New microwave flow sensor based on a left-handed transmission line resonator. In: 2007 IEEE/MTT-S International Microwave Symposium (Honolulu, HI). (2007). p. 393–6.
32. Xu K, Liu Y, Chen S, Zhao P, Peng L, Dong L, et al. Novel microwave sensors based on split ring resonators for measuring permittivity. IEEE Access. (2018) 6:26111–20. doi: 10.1109/ACCESS.2018.2834726
33. Liu Y, Zhan S, Cao G, Li J, Yang H, Liu Q, et al. Theoretical design of plasmonic refractive index sensor based on the fixed band detection. IEEE J Sel Top Quantum Electron. (2018) 25:4600206. doi: 10.1109/JSTQE.2018.2827661
34. Rawat V, Kitture R, Kumari D, Rajesh H, Banerjee S, Kale SN. Hazardous materials sensing: an electrical metamaterial approach. J Magn Magn Mater. (2016) 415:77–81. doi: 10.1016/j.jmmm.2015.11.023
35. Melik R, Unal E, Perkgoz N, Santoni B, Kamstock D, Puttlitz C, et al. Nested metamaterials for wireless strain sensing. IEEE J Sel Top Quantum Electron. (2010) 16:450–8. doi: 10.1109/JSTQE.2009.2033391
36. Kim H S, Cha S H, Roy B, Kim S, Ahn YH. Humidity sensing using THz metamaterial with silk protein fibroin. Opt Express. (2018) 26:33575–81. doi: 10.1364/OE.26.033575
37. Romero RA, Feitoza RS, Rambo CR, Sousa FR. A low-cost passive wireless capacitive sensing tag based on split-ring resonator. IEEE International Instrumentation and Measurement Technology Conference (I2MTC) Proceedings (Pisa). (2015), 434–9. doi: 10.1109/I2MTC.2015.7151307
38. Thai TT, Mehdi JM, Aubert H, Pons P, DeJean GR, Tentzeris MM, et al. A novel passive wireless ultrasensitive RF temperature transducer for remote sensing. In: 2010 IEEE MTT-S International Microwave Symposium (Anaheim, CA). (2010). p. 473–6.
39. Li J, Gai L, Li H, Hu H. A high sensitivity temperature sensor based on packaged microfibre knot resonator. Sens Actuators A Phys. (2017) 263:369–72. doi: 10.1016/j.sna.2017.06.031
40. Zemouli S, Chaabi A, Talbi HS. Design of a compact and high sensitivity temperature sensor using metamaterial. Int J Antennas Propag. (2015) 2015:301358. doi: 10.1155/2015/301358
41. Liu M, Hwang HY, Tao H, Strikwerda AC, Fan K, Keiser GR, et al. Terahertz-field-induced insulator-to-metal transition in vanadium dioxide metamaterial. Nature. (2012) 487:345–8. doi: 10.1038/nature11231
42. Lv TT, Li YX, Ma HF, Zhu Z, Li ZP, Guan CY, et al. Hybrid metamaterial switching for manipulating chirality based on VO 2 phase transition. Sci Rep. (2016) 6:1–9. doi: 10.1038/srep23186
43. Varadan V, Ji L. Temperature dependence of resonances in metamaterials. IEEE Trans Microw Theory Tech. (2010) 58:2673–81. doi: 10.1109/TMTT.2010.2065910
44. Ekmekci E, Turhan-Sayan G. Multi-functional metamaterial sensor based on a broad-side coupled SRR topology with a multi-layer substrate. Appl Phys A. (2013) 110:189–97. doi: 10.1007/s00339-012-7113-1
45. Zhang F, Chen L, Wang Y, Zhao Q, He X, Chen K. Thermally tunable electric mie resonance of dielectric cut-wire type metamaterial. Opt Express. (2014) 22:24908. doi: 10.1364/OE.22.024908
46. Karim H, Shuvo MAI, Delfin D, Lin Y, Choudhuri A, Rumpf RC. Development of metamaterial based low cost passive wireless temperature sensor. SPIE Sens Smart Struct Technol Civil Mech Aerospace Syst. (2014) 9061:662–70. doi: 10.1117/12.2045242
47. Kairm H, Delfin D, Shuvo MAI, Chavez LA, Garcia CR, Barton JH, et al. Concept and model of a metamaterial-based passive wireless temperature sensor for harsh environment applications. IEEE Sens J. (2015) 15:1445–52. doi: 10.1109/JSEN.2014.2363095
48. Karim H, Delfin D, Chavez LA, Delfin L, Martinez R, Avila J, et al. Metamaterial based passive wireless temperature sensor. Adv Eng Mater. (2017) 19:1600741. doi: 10.1002/adem.201600741
49. Qiu K, Liu Z. Thermally tunable fano resonance of a conductive rubber-based metamaterial. Microw Opt Technol Lett. (2017) 59:2960–4. doi: 10.1002/mop.30867
50. Thai TT, Chebila F, Mehdi JM, Pons P, Aubert H, DeJean GR, et al. Design and development of a millimetre-wave novel passive ultrasensitive temperature transducer for remote sensing and identification. In: The 40th European Microwave Conference (Paris). (2010). p. 45–8.
51. Ou JY, Plum E, Jiang L, Zheludev NI. Reconfigurable photonic metamaterials. Nano Lett. (2011) 11:2142–4. doi: 10.1021/nl200791r
52. Yang S, Tang C, Liu Z, Wang B, Wang C, Li J, et al. Simultaneous excitation of extremely high-Q-factor trapped and octupolar modes in terahertz metamaterials. Opt Express. (2017) 25:15938–46. doi: 10.1364/OE.25.015938
53. Du L-H, Li J, Liu Q, Zhao J-H, Zhu L-G. High-Q Fano-like resonance based on a symmetric dimer structure and its terahertz sensing application. Opt Mater Express. (2017) 7:1335–42. doi: 10.1364/OME.7.001335
54. Dong Z-G, Liu H, Cao J-X, Li T, Wang S-M, Zhu S-N, et al. Enhanced sensing performance by the plasmonic analog of electromagnetically induced transparency in active metamaterials. Appl Phys Lett. (2010) 97:114101. doi: 10.1063/1.3488020
55. Gupta M, Srivastava YK, Manjappa M, Singh R. Sensing with toroidal metamaterial. Appl Phys Lett. (2017) 110:121108. doi: 10.1063/1.4978672
56. Algorri JF, Zografopoulos DC, Ferraro A, García-Cámara B, Vergaz R, Beccherelli R, et al. Anapole modes in hollow nanocuboid dielectric metasurfaces for refractometric sensing. Nanomaterials. (2019) 9:30. doi: 10.3390/nano9010030
57. Semouchkina E, Duan R, Semouchkin G, Pandey R. Sensing based on Fano-type resonance response of all-dielectric metamaterials. Sensors. (2015) 15:9344–59. doi: 10.3390/s150409344
58. Campione S, Liu S, Basilio LI, Warne LK, Langston WL, Luk TS, et al. Broken symmetry dielectric resonators for high quality factor Fano metasurfaces. ACS Photonics. (2016) 3:2362–7. doi: 10.1021/acsphotonics.6b00556
59. Kaelberer T, Fedotov VA, Papasimakis N, Tsai DP, Zheludev NI. Toroidal dipolar response in a metamaterial. Science. (2010) 330:1510–2. doi: 10.1126/science.1197172
60. Liu Z, Du S, Cui A, Li Z, Fan Y, Chen S, et al. High-quality-factor mid-infrared toroidal excitation in folded 3D metamaterials. Adv Mater. (2017) 29:1606298. doi: 10.1002/adma.201606298
61. Ma L, Chen D, Zheng W, Li J, Wang W, Liu Y, et al. Thermally tunable high-Q metamaterial and sensing application based on liquid metals. Optics Express. (2021) 29:6069–79. doi: 10.1364/OE.418024
62. Evlyukhin AB, Yu YF, Bakker RM, Chipouline A, Kuznetsov AI, Luk'yanchuk B, et al. Nonradiating anapole modes in dielectric nanoparticles. Nat Commun. (2015) 6:8069. doi: 10.1038/ncomms9069
63. Basharin AA, Chuguevsky V, Volsky N, Kafesaki M, Economou EN. Extremely high Q-factor metamaterials due to anapole excitation. Phys Rev B. (2017) 95:035104. doi: 10.1103/PhysRevB.95.035104
64. Wu PC, Liao CY, Savinov V, Chung TL, Chen WT, Huang YW, et al. Optical anapole metamaterial. ACS Nano. (2018) 12:1920–7. doi: 10.1021/acsnano.7b08828
65. Savinov V, Papasimakis N, Tsai DP, Zheludev NI. Optical anapoles. Commun Phys. (2019) 2:69. doi: 10.5258/SOTON/D0914
66. Sabri L, Huang Q, Liu JN, Cunningham BT. Design of anapole mode electromagnetic field enhancement structures for biosensing applications. Optics Express. (2019) 27:7196–212. doi: 10.1364/OE.27.007196
67. Liu X, Liu Z, Hua M, Wang L, Wang K, Zhang W, et al. Tunable terahertz metamaterials based on anapole excitation with graphene for reconfigurable sensors. ACS Appl Nano Mater. (2020) 3:2129–33. doi: 10.1021/acsanm.0c00141
68. Chen J, Nie H, Zha T, Mao P, Tang C, Shen X, et al. Optical magnetic field enhancement by strong coupling in metamaterials. J Lightwave Technol. (2018) 36:2791–5. doi: 10.1364/JLT.36.002791
69. Chen J, Nie H, Peng C, Qi S, Tang C, Zhang Y, et al. Enhancing the magnetic plasmon resonance of three-dimensional optical metamaterials via strong coupling for high-sensitivity sensing. J Lightwave Technol. (2018) 36:3481–5. doi: 10.1109/JLT.2018.2846019
70. Chen J, Nie H, Tang C, Cui Y, Yan B, Zhang Z, et al. Highly sensitive refractive-index sensor based on strong magnetic resonance in metamaterials. Appl Phys Express. (2019) 12:052015. doi: 10.7567/1882-0786/ab14fa
71. Chen J, Qi S, Hong X, Gu P, Wei R, Tang C, et al. Highly sensitive 3D metamaterial sensor based on diffraction coupling of magnetic plasmon resonances. Results Phys. (2019) 15:102791. doi: 10.1016/j.rinp.2019.102791
72. Chen J, Peng C, Qi S, Zhang Q, Tang C, Shen X, et al. Photonic microcavity-enhanced magnetic plasmon resonance of metamaterials for sensing applications. IEEE Photonics Technol Lett. (2018) 31:113–6. doi: 10.1109/LPT.2018.2881989
73. Chen J, Chen S, Gu P, Yan Z, Tang C, Xu Z, et al. Electrically modulating and switching infrared absorption of monolayer graphene in metamaterials. Carbon. (2020) 162:187–94. doi: 10.1016/j.carbon.2020.02.032
Keywords: metamaterial, sensor, resonance, high quality factor, high precision sensing
Citation: Ma L, Chen D, Zheng W, Li J, Zahra S, Liu Y, Zhou Y, Huang Y and Wen G (2021) Advanced Electromagnetic Metamaterials for Temperature Sensing Applications. Front. Phys. 9:657790. doi: 10.3389/fphy.2021.657790
Received: 24 January 2021; Accepted: 29 March 2021;
Published: 30 April 2021.
Edited by:
Weiren Zhu, Shanghai Jiao Tong University, ChinaReviewed by:
Jinhui Shi, Harbin Engineering University, ChinaHe-Xiu Xu, Air Force Engineering University, China
Jing Chen, Nanjing University of Posts and Telecommunications, China
Copyright © 2021 Ma, Chen, Zheng, Li, Zahra, Liu, Zhou, Huang and Wen. This is an open-access article distributed under the terms of the Creative Commons Attribution License (CC BY). The use, distribution or reproduction in other forums is permitted, provided the original author(s) and the copyright owner(s) are credited and that the original publication in this journal is cited, in accordance with accepted academic practice. No use, distribution or reproduction is permitted which does not comply with these terms.
*Correspondence: Jian Li, bGowMDEmI3gwMDA0MDt1ZXN0Yy5lZHUuY24=; Yongjun Huang, eW9uZ2p1bmgmI3gwMDA0MDt1ZXN0Yy5lZHUuY24=