- 1Prokhorov General Physics Institute of the Russian Academy of Sciences, Moscow, Russia
- 2V. M. Gorbatov Federal Research Center for Food Systems of Russian Academy of Sciences, Moscow, Russia
- 3Institute of Cell Biophysics of the Russian Academy of Sciences, PSCBR RAS, Moscow, Russia
The development of antibiotic resistance of bacteria is one of the most pressing problems in world health care. One of the promising ways to overcome microbial resistance to antibiotics is the use of metal nanoparticles and their oxides. In particular, numerous studies have shown the high antibacterial potential of zinc oxide nanoparticles (ZnO-NP) in relation to gram-positive and gram-negative bacteria. This mini-review includes an analysis of the results of studies in recent years aimed at studying the antibacterial activity of nanoparticles based on zinc oxide. The dependence of the antibacterial effect on the size of the applied nanoparticles in relation to E. coli and S. aureus is given. The influence of various ways of synthesis of zinc oxide nanoparticles and the main types of modifications of NP-ZnO to increase the antibacterial efficiency are also considered.
Introduction
Today, antibiotics are the “gold standard” in treatment of many bacterial infections [1, 2]. However, microorganisms can develop antibiotic resistance. The majority of pathogenic microorganisms have an ability to develop resistance to at least some antimicrobial agents [3]. Antibiotic resistance in bacteria is achieved by several mechanisms: prevention of drug penetration into a cell [4, 5], changes in an antibiotic target [6, 7], enzymatic inactivation of antibiotics [8], active excretion of an antibiotic from a cell [4] and so on.
According to the data of the World Health Organization (WHO), lower respiratory infections and gastrointestinal infections are among the top ten factors of morbidity and mortality [9]. Appearance of antibiotic resistant strains significantly increased the number of deaths and severity of bacterial infections. Deaths of patients due to antibiotic resistant bacterial strains exceed the total number of global deaths due to cancer and diabetes mellitus [10, 11]. Despite the significant quantity of available antibiotics, resistance to almost all of them was confirmed. Antibiotic resistance emerges shortly after a new drug is approved for use [3, 12]. The indicated events urged WHO to endorse the Global action plan on antimicrobial resistance in 2015 [13]. Secondary bacterial infections can be a cause of increased lethality among patients in intensive care; in particular, bacterial co-infection and secondary infection are found in patients with COVID-19 [14, 15]. All above mentioned make a search for new antimicrobial preparations a high priority task of public health in the world.
The number of scientific publications devoted to a search for new antimicrobial compounds is about 99000 only in 2018–2020; 5900 of them are devoted to a search for antibacterial compounds based on metal compounds [16].
Humans have been used antimicrobial properties of several metals and their ions since ancient times. For example, utensils from Cu and Ag were used in ancient Persia, Rome and Egypt [17]. It is known today that a wide range of metals has the antimicrobial activity: Ag, Al, As, Cd, Co, Cr, Cu, Fe, Ga, Hg, Mo, Mn, Ni, Pb, Sb, Te, Zn [18–20].
The basis of the antimicrobial activity of metals is an ability of metal ions to inhibit enzymes [21, 22], facilitate generation of reactive oxygen species (the Fenton reaction) [23], cause the damage of cell membranes [24], prevent uptake of vitally important microelements by microbes [25]; moreover, several metals can exert the direct genotoxic activity [26–28].
The use of nanoparticles based on metals and their oxides is of great interest. One of the well-studied metals affecting biological objects is zinc (Zn) and its oxide (ZnO). Zinc is an active element and exhibits strong reduction properties. It can easily oxidize to form zinc oxide. Zinc plays an important role in the human body, since it is one of the most important trace elements [29]. Zinc is found in all tissues of the human body, with the highest concentration found in myocytes (85% of the total zinc content in the body) [30]. Zinc has been shown to be critical for the proper functioning of a large number of macromolecules and enzymes, where it plays both a catalytic (coenzyme) and structural role. In turn, structures called Zincfinger provide a unique scaffold that allows protein subdomains to interact with either DNA or other proteins [31].
Zinc is also essential for the functioning of metalloproteins. Although zinc is considered relatively non-toxic, there is growing evidence that free zinc ions can cause negative effects on cells. To assess the toxicity of a test substance in vitro, animal cell cultures are usually used. It is known that nerve cells are the most sensitive to exogenous influences [32–34]. It has been reported that exposure to zinc ions leads to neuronal degradation [35]. To eliminate the cytotoxic effect, zinc cations are bound with bioactive ligands (for example, proteins) and zinc oxide nanoparticles are synthesized. Nanostructured ZnO can have various morphological forms and properties.
At present, there is a growing interest to nanoparticles of metals and metal oxides as compounds with antibacterial potential: Ag [36, 37], Au [38], ZnO [10], TiO2 [39, 40], CuO [41, 42], Fe2O3 [43, 44]. ZnO has many applications in engineering and medicine. In engineering, ZnO nanoparticles are used in solar cells [45, 46], gas sensors, in particular, sensors for Liquefied petroleum gas (LPG) and EtOH [47], chemical sensors and biosensors, in LEDs, photodetectors [48, 49]. In biology and medicine, the cytostatic activity of ZnO nanoparticles (ZnO-NPs) against cancer cells [50], antimicrobial and fungicidal activities [51, 52], anti-inflammatory activity [53, 54], ability to accelerate wound healing [55], a possibility to use in bioimaging due to chemiluminescent properties of nanoparticles [56, 57], antidiabetic properties [58, 59] are of great interest.
ZnO nanoparticles have several advantages: high antibacterial effectiveness at low concentrations (0.16–5.00 mmol/L), activity against a wide range of strains, relatively low cost [43, 51, 60]. ZnO nanoparticles are synthesized by the physio-chemical sol-gel method from zinc salts [43, 61], sol-gel combustion method [62], solochemical method [63], chemical synthesis at low temperatures [64] and mechanical method [65]. In several cases, stabilizing agents, for example, chitosan are added [66, 67].
The mechanisms of action of zinc oxide nanoparticles can be reduced to the following: disruption of the cell membrane [68, 69], binding to proteins and DNA, generation of reactive oxygen species (ROS) [10, 70, 71], disturbance of the processes of bacterial DNA amplification, alteration (more often, down-regulation) of expression in a wide range of genes [72]. The direct bactericidal action of ZnO nanoparticles against both gram-negative and gram-positive bacteria and fungi was shown [73, 66, 74].
Nanoparticles of a number of metal oxides lead to the production of ROS upon interaction with bacteria [75]. The metal ions released by the nanoparticles affect the respiratory chain and inhibit some enzymes. This leads to the formation and accumulation of singlet oxygen, hydroxyl radical, hydrogen peroxide, superoxide anions, and other ROS. ROS can cause damage to the internal components of bacteria, such as proteins and DNA [76].
It has been shown that exposure to sublethal ROS concentrations can stimulate the manifestation of defense reactions. This process is called hormesis [77]. Hormesis induces defense mechanisms on two levels. The first level is enzymatic (short-term reaction). At this level, antioxidant enzymes are activated. The second level is long-term adaptation. Long-term adaptation consists of two sublevels: transcriptional and genomic. At the level of transcription, ROS induces adaptation due to the activation of antioxidant mechanisms within a few hours or days [78]. At the genomic level, ROS can cause damage to the DNA structure, which activates the mechanisms for repairing DNA damage. These mechanisms include homologous recombination and excisional repair. In these mechanisms, two of the DNA polymerases responsible for DNA synthesis have poor validation activity and may include abnormal bases in DNA strands, which leads to a high frequency of spontaneous mutations and genome plasticity under adverse influences [79]. Such plasticity of the genome can lead to the development of resistance to metals and metal oxide nanoparticles [80].
The adaptation mechanisms of bacteria in relation to nanoparticles also include overexpression of extracellular substances by bacterial cells, such as flagellin, which form an extracellular matrix that promotes agglomeration and deactivation of nanoparticles [81]. Despite the existing mechanisms of adaptation of bacteria to the impact, numerous studies have noted the high antibacterial potential of ZnO nanoparticles.
Literature Review
Despite the apparent wide range of strains, against which nanoparticles exert the antimicrobial activity, their effectiveness against particular strains can be significantly different. As a rule, gram-negative bacteria are less sensitive to ZnO nanoparticles than gram-positive bacteria [62, 66, 82]. Somewhat higher resistance of gram-negative bacteria can be explained by the peculiarities of their cell wall structure. In contrast to gram-positive bacteria, the cell wall of gram-negative bacteria includes the additional outer membrane containing lipopolysaccharides (LPS) [83]. It is shown that LPS can improve the barrier properties of the outer membrane and, therefore, increase bacterial resistance, in particular, to antibiotics [84]. Epidemiologically significant microorganisms deserve a special attention, for example, Mycobacterium tuberculosis, against which ZnO nanoparticles exert the bacteriostatic effect but not bactericidal [85].
On the contrary, several microorganisms (for instance, Campylobacter jejuni) have an increased sensitivity to ZnO nanoparticles, which make them a convenient model for studying molecular 126 mechanisms of the antimicrobial effect of nanoparticles [24]. ZnO nanoparticles (ZnONPs) disturb the processes of bacterial DNA amplification, reduce expression of a wide range of genes of C. jejuni that are responsible for virulence, significantly alter expression of genes of oxidative and general stress [24]. An important feature of ZnO nanoparticles used in one of the studies is the antibacterial activity against resistant bacterial strains, for example, carbapenem-resistant Acinetobacter baumannii (RS-307 and RS-6694) [86]. The dependence of effectiveness on a bacterial growth phase was shown for ZnO nanoparticles. In particular, ZnO nanoparticles are effective against gram- negative and gram-positive bacteria at the exponential growth phase; however, the antibacterial properties of nanoparticles are significantly decreased at the lag and stationary phases [52]. A range of bactericidal concentrations of ZnO nanoparticles is usually significantly less than a range of 4 [62]. At present, an active search for methods to increase the antimicrobial action of nanoparticles is carried out. Below we present the literature search. Nanoparticles are classified by the method for synthesis, size, structure, form, absence or presence of the envelope or nucleus. The objects, on which nanoparticles influenced, are classified by types, biological effect of nanoparticles, concentration of nanoparticles, duration of exposure, temperature and environment. The data are presented in table 1.
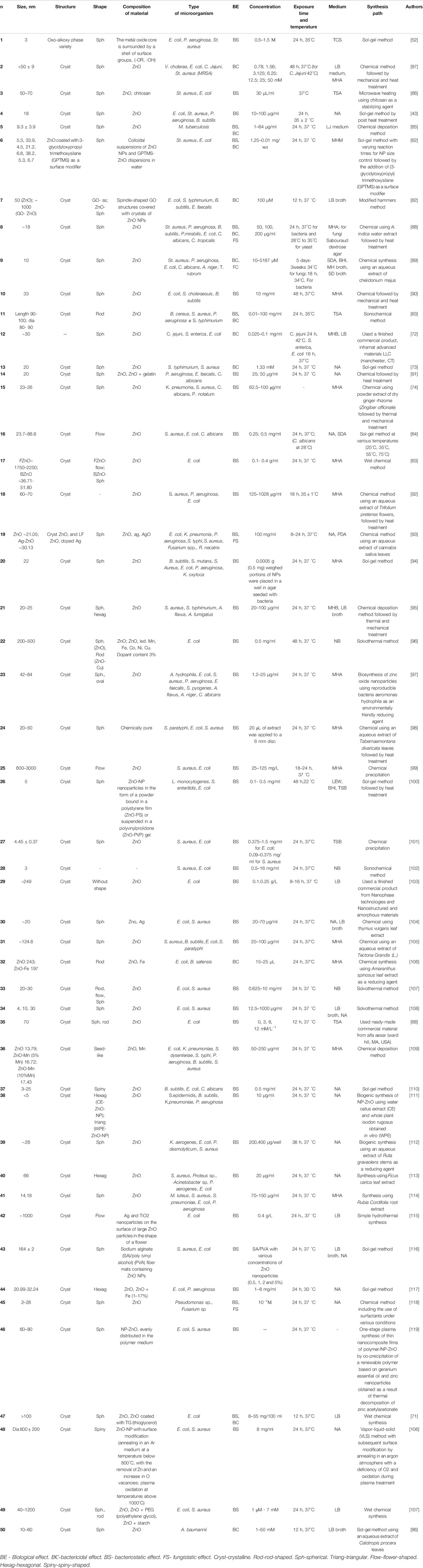
TABLE 1. Main characteristics, physicochemical and biological parameters of ZnO nanoparticles presented in the review.
Let us consider proposed methods for increasing antibacterial properties of ZnO nanoparticles. The first method for increasing antibacterial properties of ZnO nanoparticles is to use a combination of different metal compounds [52, 90]. For example, the CuO and ZnO have comparable effectiveness against gram-negative Escherichia coli and gram-positive Staphylococcus aureus at the exponential growth phase. ZnO nanoparticles were practically inactive at the lag and stationary phases, while CuO nanoparticles retained the significant activity [52]. Ag and ZnО nanoparticles in different ratios inhibit the growth of antibiotic resistant Mycobacterium tuberculosis strains but did not lead to bacterial death [85]. ZrO2-ZnO nanoparticles have the pronounced antimicrobial action in contrast to ZrO2 nanoparticles, but the antimicrobial effect of ZrO2-ZnO nanoparticles does not exceed that of ZnO nanoparticles [94]. However, combinations of metal oxides not always give the synergetic effect. In particular, CdO-ZnO nanoparticles have the antimicrobial action comparable with that of CdO nanoparticles [90]. Doping of ZnO nanoparticles with the Fe ions enables achieving a significant antibacterial effect against E. coli, Pseudomonas aeruginosa [117]. TiO2/ZnO nanoparticles have more pronounced bactericidal effect against E. coli compared to ZnO nanoparticles. Ag/TiO2/ZnO nanoparticles are more effective than TiO2/ZnO nanoparticles [115]. Compared to ZnO nanoparticles, ZnO-Mn nanoparticles have higher antimicrobial activity against K. pneumoniae, Shigella dysenteriae, S. enterica Typhimurium, P. aeruginosa and other bacteria [109].
The second method for increasing antimicrobial effectiveness is to use combinations of ZnO nanoparticles and carbon nanoparticles, in particular, spindle-shaped graphene oxide (GO) nanoparticles [68, 108, 109]. It is shown that GO-ZnO nanoparticles effectively inhibit the growth of gram-negative (E. coli, S. typhimurium) and gram-positive (Bacillus subtilis, Enterococcus faecalis) bacteria [68]. With that, the antibacterial effectiveness of the mixture of GO-ZnO nanoparticles turned to be nearly twice as high as that of ZnO nanoparticles and almost four times higher than that of GO nanoparticles [82].
The third method is coating ZnO nanoparticles with modifying agents. Gelatin-coated ZnO nanoparticles showed higher inhibition of the growth of gram-negative bacteria compared to gram-positive bacteria [91]. As was mentioned above, overcoming antibiotic resistance in gram-negative bacteria is a more difficult task. Gelatin-coated ZnO nanoparticles inhibit biofilm formation of C. albicans (an additional resistance factor) [91]. These nanoparticles also inhibit angiogenesis in chick embryos, which makes them candidates for the development of preparations preventing undesirable angiogenesis [91]. The chemical surface modification of nanoparticles using (3-glycidyloxypropyl) trimethoxysilane (GPTMS) and decrease in a size up to 5 nm lead to an increase in antimicrobial effectiveness of nanoparticles against S. aureus [62]. Treatment with polystyrene increased the bacteriostatic effect of ZnO nanoparticles against E. coli and Listeria monocytogenes; with that, uncoated ZnO nanoparticles did not have the bacteriostatic effect against L. monocytogenes [100]. Modification of ZnO nanoparticles with polyethylene glycol or starch also alters properties of nanoparticles [121]. Modification with polyethylene glycol increased the bacteriostatic effect of ZnO nanoparticles against E. coli и S. aureus; with that, effectiveness against gram-negative bacteria was higher. Polyethylene enhanced cytotoxicity of ZnO nanoparticles toward the cancer cell line (MG-63) by induction of apoptosis. Modification with starch allowed retention of antibacterial properties of ZnO nanoparticles and reduction of cytotoxicity compared to modification with polyethylene glycol [121]. Treatment with thioglycerol, contrary to the expectations, did not increase the bacteriostatic and bactericidal activity of ZnO nanoparticles [71]. Polymer films from sodium alginate/polyvinyl alcohol gained bacteriostatic properties after incorporation of ZnO nanoparticles, which can be used in the development of more durable materials [116].
The fourth method is modification of the synthesis method leading to changes in the geometrical characteristics of nanoparticles. ZnO nanoparticles synthesized by the sonochemical method have more pronounced inhibitory properties against Bacillus cereus, S. aureus, S. Typhimurium and Pseudomonas aeruginosa than ZnO nanoparticles synthesized by the classical physio-chemical methods [63]. Nanoparticles synthesized at comparatively low temperatures are flower-shaped and have the comparable antimicrobial activity against gram-positive (S. aureus) and gram-negative (E. coli) bacteria and, to a lesser extent, fungi (C. albicans) [64]. When using ROS photocatalytic generation and release of Zn2+, flower-shaped ZnO nanoparticles show more pronounced antimicrobial activity against E. coli than more lacunary hexagon-shaped ZnO-NPs [70].
Antibacterial properties of nanoparticles depend on their size [122-124]. For several nanoparticles, the highest antibacterial activity is achieved at the smallest size [103, 107, 125]; however, we have not found in the literature a clear dependence of antibacterial effectiveness on a nanoparticle size. We had to analyze literature by ourselves and build a graph reflecting a dependence of an inhibition zone size on a size of ZnO nanoparticles (Figure 1). Analysis of literature allows stating that the highest potential antimicrobial effectiveness of nanoparticles against both E. coli, and S. aureus is observed at a nanoparticle size of about 100 nm. It is necessary to note that “green chemistry” not always leads to synthesis of effective nanoparticles. For example, in studies on S. aureus, only two types of nanoparticles out of six (33%) had the antibacterial activity at a level higher than average. When studying on E. coli, only one of five (20%) types of nanoparticles generated using “green chemistry” exerted the antibacterial activity at a level higher than average. Therefore, it can be suggested that nanoparticles generated by “green chemistry” still have insufficient effectiveness.
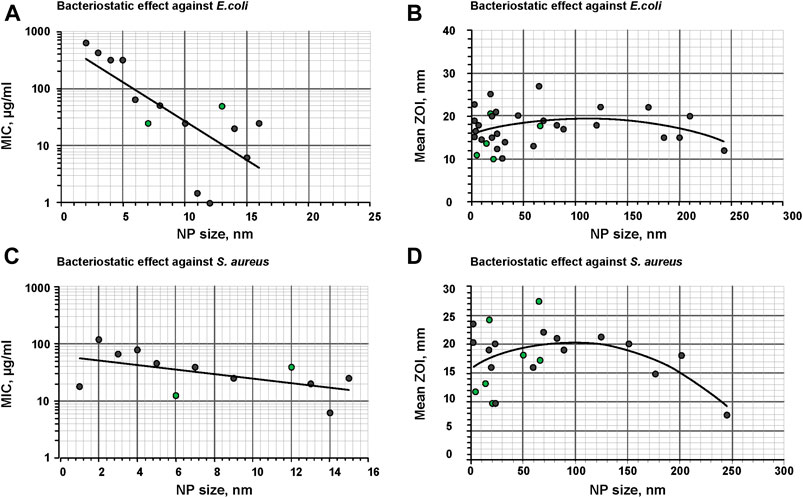
FIGURE 1. Dependence of the minimum inhibitory capacity on the size of NP-ZnO, as well as the dependence of the size of the zone of inhibition on the size of NP-ZnO against gram-negative bacteria by the example of E. coli(A and B) and gram-positive bacteria by the example of S. aureus(C and D). Gray dots are samples synthesized by methods without using “green synthesis”, green dots are samples obtained using “green synthesis”.
As can be seen in Figures 1B,D, quite high dispersion of effectiveness is seen in the region of small sizes of nanoparticles (1–50 nm). Therefore, we studied the dependence of the minimum inhibitory concentration (MIC) on sizes of ZnO nanoparticles (Figures 1A,C). It is shown that the use of nanoparticles with sizes of up to 10 nm is not effective. Usually, at these average sizes of nanoparticles, distribution of nanoparticles by sizes is rather complex and not always narrow. Nanoparticles with small sizes are quite prone to aggregation. Apparently, high dispersion of antibacterial activity at small sizes of nanoparticles can be explained by this fact.
Flower-shaped ZnO nanoparticles can reach large sizes (up to 3 µm) and demonstrate the antimicrobial activity against both gram-positive (S. aureus) and gram-negative (E. coli) bacteria [99]. For spherical ZnO nanoparticles, the antimicrobial activity practically does not depend on the type of a targeted organism. Hexagonal ZnO nanoparticles have higher bactericidal activity against antibiotic resistant Staphylococcus epidermidis, B. subtilis, Klebsiella pneumoniae and P. aeruginosa strains compared to ZnO-NPs with the triangular shape [111]. Thorn-like ZnO nanoparticles cause significant reduction in the growth of B. subtilis, E. coli and C. albicans colonies demonstrating the antibacterial and antifungal activities [110].
The fifth method is modification by physio-chemical methods, for example, by annealing in the Ar environment at high temperatures, or plasma oxidation. With that, the effects of modification can be different: Ar annealing decreases the antibacterial activity of ZnO nanoparticles, while plasma oxidation improves antibacterial properties of ZnO nanoparticles against E. coli and S. aureus [120]. The sixth method is the use of additives causing photocatalysis of reactive oxygen species (ROS). This modification enables a significant increase in antibacterial properties of ZnO nanoparticles [70, 93]. The seventh method is the so-called “green synthesis” [126–128]. ZnO nanoparticles generated by “green synthesis” have the antimicrobial activity against gram-negative and gram-positive bacteria, as well as several fungi of the genus Candida [88]. In turn, nanoparticles synthesized using the Tabernaemontana divaricata extract demonstrated the antibacterial activity against S. aureus, E. coli and lower activity against S. enterica Paratyphoid [98]. The eight method is a change in the environment conditions. At acidic pH levels, ZnO nanoparticles had higher bacteriostatic action against S. aureus and E. coli than at neutral pH [101]. The combination of all approaches described above can be most promising, for example, the use of Ag-ZnO nanoparticles synthesized in the Cannabis sativa extract. The generated nanoparticles can be used in combination with photocatalysis and have the antibacterial and antifungal activities.
Conclusion
Zinc oxide nanoparticles have significant antibacterial potential. The use of various methods of synthesis, chemical modification, as well as joint use with other nanomaterials affects the physical and morphological characteristics of nanoparticles, which, in turn, leads to a change in their antibacterial properties. As a result, nanoparticles based on zinc oxide are increasingly used not only in nanoelectronics and optics, but also in such industrial areas as cosmetic, food, rubber, pharmaceutical, household chemicals, etc. The use of packaging with incorporated zinc oxide nanoparticles is possible will allow in the future to prevent the growth of microorganisms and spoilage of food. In turn, the use of medical dressing materials containing ZnO nanoparticles will allow avoiding microbial contamination of the wound and promotes its early healing. Thus, zinc oxide nanoparticles can be considered as a promising new generation antimicrobial agent.
Author Contributions
SG designed this topic. DB, MR contributed to collecting related references. DB made a table. DS, SG, MR wrote most of the manuscript. AS and AL were involved in discussing the manuscript and translating it into English.
Funding
This work was supported by the Ministry of Science and Education of the Russian Federation (Grant Agreement 075-15-2020-775).
Conflict of Interest
The authors declare that the research was conducted in the absence of any commercial or financial relationships that could be construed as a potential conflict of interest.
Acknowledgments
Authors acknowledge the immense help received from the scholars whose articles are cited and included in references to this manuscript. The authors are also grateful to authors/editors/publishers of all those articles, journals and books from where the literature for this article has been reviewed and discussed.
References
1. Davies J, Davies D. Origins and evolution of antibiotic resistance. Microbiol Mol Biol Rev (2010) 74(3):417–33. doi:10.1128/MMBR.00016-10
2. Cavalieri F, Tortora M, Stringaro A, Colone M, Baldassarri L. Nanomedicines for antimicrobial interventions. J Hosp Infect (2014) 88(4):183–90. doi:10.1016/j.jhin.2014.09.009
3. Reygaert W. An overview of the antimicrobial resistance mechanisms of bacteria. J AIMS microbiology (2018) 4(3):482–501. doi:10.3934/microbiol.2018.3.482
4. Kumar A, Schweizer HP. Bacterial resistance to antibiotics: active efflux and reduced uptake. Adv Drug Deliv Rev (2005) 57(10):1486–513. doi:10.1016/j.addr.2005.04.004
5. Blair JM, Richmond GE, Piddock LJ. Multidrug efflux pumps in Gram-negative bacteria and their role in antibiotic resistance. Future Microbiol (2014) 9(10):1165–77. doi:10.2217/fmb.14.66
6. Reygaert W. Methicillin-resistant Staphylococcus aureus (MRSA): molecular aspects of antimicrobial resistance and virulence. Clin Lab Sci (2009) 22(2):115–9. doi:10.29074/ascls.22.2.115
7. Randall C, Mariner K, Mariner KR, Chopra I, O'Neill AJ. The target of daptomycin is absent from Escherichia coli and other gram-negative pathogens. Antimicrob Agents Chemother (2013) 57(1):637–9. doi:10.1128/AAC.02005-12
8. Blair JM, Webber MA, Baylay AJ, Ogbolu DO, Piddock LJ. Molecular mechanisms of antibiotic resistance. Nat Rev Microbiol (2015) 13(1):42–51. doi:10.1038/nrmicro3380
9.World Health Organization. Global action plan on antimicrobial resistance. Geneva, Switzerland: WHO (2015).
10. Gold K, Slay B, Knackstedt M, Gaharwar A. Antibacterial activity of metal and metal-oxide based nanoparticles. Adv Ther (2018) 1(3):1700033. doi:10.1002/adtp.201700033
11. Zaman SB, Hussain MA, Nye R, Mehta V, Mamun KT, Hossain N. A review on antibiotic resistance: alarm bells are ringing. Cureus (2017) 9(6):e1403. doi:10.7759/cureus.1403
12. Habboush Y, Guzman N. Antibiotic resistance. StatPearls [internet] (2020), Available at: https://www.ncbi.nlm.nih.gov/books/NBK513277/.
14. Langford B, So M, Raybardhan S, Leung V, Westwood D, MacFadden D, et al. Bacterial co-infection and secondary infection in patients with COVID-19: a living rapid review and meta-analysis. Clin Microbiol Infect (2020) 26(12):1622–29. doi:10.1016/j.cmi.2020.07.016
15. Sharifipour E, Shams S, Esmkhani M, Khodadadi J, Fotouhi-Ardakani R, Koohpaei A, et al. Evaluation of bacterial co-infections of the respiratory tract in COVID-19 patients admitted to ICU. BMC Infect Dis (2020) 20(1):646. doi:10.1186/s12879-020-05374-z
16. Ncbi (2020) Available at: https://pubmed.ncbi.nlm.nih.gov/?term=antibacterial&filter=years.2017-2021&sort=date
17. Alexander J History of the medical use of silver. Surg Infect (2009) 10(3):289–92. doi:10.1089/sur.2008.9941
18. Yasuyuki M, Kunihiro K, Kurissery S, Kanavillil N, Sato Y, Kikuchi Y Antibacterial properties of nine pure metals: a laboratory study using Staphylococcus aureus and Escherichia coli. Biofouling (2010) 26(7):851–8. doi:10.1080/08927014.2010.527000
19. Lemire JA, Harrison JJ, Turner RJ Antimicrobial activity of metals: mechanisms, molecular targets and applications. Nat Rev Microbiol (2013) 11(6):371–84. doi:10.1038/nrmicro3028
20. Turner R Metal-based antimicrobial strategies. Microb Biotechnol (2017) 10(5):1062–5. doi:10.1111/1751-7915.12785
21. Stadtman E Oxidation of free amino acids and amino acid residues in proteins by radiolysis and by metal-catalyzed reactions. Annu Rev Biochem (1993) 62:797–821. doi:10.1146/annurev.bi.62.070193.004053
22. Stadtman ER, Levine RL Free radical-mediated oxidation of free amino acids and amino acid residues in proteins. Amino Acids (2003) 25(3–4):207–18. doi:10.1007/s00726-003-0011-2
23. Valko M, Morris H, Cronin MT Metals, toxicity and oxidative stress. Curr Med Chem (2005) 12(10):1161–208. doi:10.2174/0929867053764635
24. Li WR, Xie XB, Shi QS, Zeng HY, Ou-Yang YS, Chen YB Antibacterial activity and mechanism of silver nanoparticles on Escherichia coli. Appl Microbiol Biotechnol (2009) 85(4):1115–22. doi:10.1007/s00253-009-2159-5
25. Pereira Y, Lagniel G, Godat E, Baudouin-Cornu P, Junot C, Labarre J Chromate causes sulfur starvation in yeast. Toxicol Sci (2008) 106(2):400–12. doi:10.1093/toxsci/kfn193
26. Nishioka H Mutagenic activities of metal compounds in bacteria. Mutat Res (1975) 31(3):185–9. doi:10.1016/0165-1161(75)90088-6
27. Green MH, Muriel WJ, Bridges BA Use of a simplified fluctuation test to detect low levels of mutagens. Mutat Res (1976) 38(1):33–42. doi:10.1016/0165-1161(76)90077-7
28. Wong P Mutagenicity of heavy metals. Bull Environ Contam Toxicol (1988) 40(4):597–603. doi:10.1007/BF01688386
29. Maret W Metals on the move: zinc ions in cellular regulation and in the coordination dynamics of zinc proteins. Biometals (2011) 24(3):411–8. doi:10.1007/s10534-010-9406-1
30. Król A, Pomastowski P, Rafińska K, Railean-Plugaru V, Buszewski B Zinc oxide nanoparticles: synthesis, antiseptic activity and toxicity mechanism. Adv Colloid Interface Sci (2017) 249:37–52. doi:10.1016/j.cis.2017.07.033
31. Klug A, Rhodes D Zinc fingers: a novel protein fold for nucleic acid recognition. Cold Spring Harbor Symp Quant Biol (1987) 52:473–82. doi:10.1101/sqb.1987.052.01.054
32. Timashev P, Bardakova K, Minaev N, Demina T, Mishchenko T, Mitroshina E, et al. Compatibility of cells of the nervous system with structured biodegradable chitosan-based hydrogel matrices. Appl Biochem Microbiol (2016) 52:508–14. doi:10.1134/S0003683816050161
33. Sakharnova T, Vedunova MV, Mukhina IV Brain-derived neurotrophic factor (BDNF) and its role in the functioning of the central nervous system. Neurochem J (2012) 6:251–9. doi:10.1134/S1819712412030129
34. Mitroshina EV, Krivonosov MI, Burmistrov DE, Savyuk MO, Mishchenko TA, Ivanchenko MV, et al. Signatures of the consolidated response of astrocytes to ischemic factors in vitro. Int J Mol Sc (2020) 21(21):7952. doi:10.3390/ijms21217952
35. Frederickson C, Koh J, Bush AI The neurobiology of zinc in health and disease. Nat Rev Neurosci (2005) 6:449–62. doi:10.1038/nrn1671
36. Kim JS, Kuk E, Yu KN, Kim JH, Park SJ, Lee HJ, et al. Antimicrobial effects of silver nanoparticles. Nanomedicine (2007) 3(1):95–101. doi:10.1016/j.nano.2006.12.001
37. Salomoni R, Léo P, Montemor A, Rinaldi B, Rodrigues M Antibacterial effect of silver nanoparticles in Pseudomonas aeruginosa. Nanotechnol Sci Appl (2017) 10:115–21. doi:10.2147/NSA.S133415
38. Karimi Zarchi AA, Amini SM, Salimi A, Kharazi S Synthesis and characterization of liposomal doxorubicin with loaded gold nanoparticles. IET Nanobiotechnol (2018) 12(6):846–9. doi:10.1049/iet-nbt.2017.0321
39. Allahverdiyev AM, Abamor ES, Bagirova M, Rafailovich M Antimicrobial effects of TiO(2) and Ag(2)O nanoparticles against drug-resistant bacteria and leishmania parasites. Future Microbiol (2011) 6(8):933–40. doi:10.2217/fmb.11.78
40. Heinlaan M, Ivask A, Blinova I, Dubourguier HC, Kahru A Toxicity of nanosized and bulk ZnO, CuO and TiO2 to bacteria Vibrio fischeri and crustaceans Daphnia magna and Thamnocephalus platyurus. Chemosphere (2008) 71(7):1308–16. doi:10.1016/j.chemosphere.2007.11.047
41. Ren G, Hu D, Cheng EW, Vargas-Reus MA, Reip P, Allaker RP Characterization of copper oxide nanoparticles for antimicrobial applications. Int J Antimicrob Agents (2009) 33(6):587–90. doi:10.1016/j.ijantimicag.2008.12.004
42. Mahapatra O, Bhagat M, Gopalakrishnan C, Arunachalam K Ultrafine dispersed CuO nanoparticles and their antibacterial activity. J Exp Nanosci (2008) 3(3):185–93. doi:10.1080/17458080802395460
43. Azam A, Ahmed AS, Oves M, Khan MS, Habib SS, Memic A Antimicrobial activity of metal oxide nanoparticles against Gram-positive and Gram-negative bacteria: a comparative study. Int J Nanomed (2012) 7:6003–9. doi:10.2147/IJN.S35347
44. Rufus A, Sreeju N, Vilas V, Philip D Biosynthesis of hematite (α-Fe2O3) nanostructures: size effects on applications in thermal conductivity, catalysis, and antibacterial activity. J Mol Liq (2017) 242:537–49. doi:10.1016/j.molliq.2017.07.057
45. Beek W, Wienk M, Janssen R Efficient hybrid solar cells from zinc oxide nanoparticles anda conjugated polymer. Adv Mater (2004) 16(12):1009–13. doi:10.1002/adma.200306659
46. Suliman A, Tang Y, Xu L Preparation of ZnO nanoparticles and nanosheets and their application to dye-sensitized solar cells. Sol Energy Mater Sol Cell (2007) 91(18):1658–62. doi:10.1016/j.solmat.2007.05.014
47. Baruwati B, Kumar D, Manorama S Hydrothermal synthesis of highly crystalline ZnO nanoparticles: a competitive sensor for LPG and EtOH. Sensor Actuator B Chem (2006) 119(2):676–82. doi:10.1016/j.snb.2006.01.028
48. Vaseem M, Umar A, Hahn Y ZnO nanoparticles: growth, properties, and applications. In: Metal oxide nanostructures and their applications. 5(1). Stevenson Ranch, CA: American Scientific Publishers (2010). 1–36.
49. Chang S, Chen K Zinc oxide nanoparticle photodetector. J Nanomater (2012) 602–398. doi:10.1155/2012/602398
50. Mishchenko T, Mitroshina E, Balalaeva I, Krysko O, Vedunova M, Krysko D An emerging role for nanomaterials in increasing immunogenicity of cancer cell death. Biochim Biophys Acta Rev Canc (2019) 1871(1):99–108. doi:10.1016/j.bbcan.2018.11.004
51. Houskova V, Stengl V, Bakardjieva S, Murafa N, Kalendova A, Oplustil F Zinc oxide prepared by homogeneous hydrolysis with thioacetamide, its destruction of warfare agents, and photocatalytic activity. J Phys Chem A (2007) 111(20):4215–21. doi:10.1021/jp070878d
52. Dadi R, Azouani R, Traore M, Mielcarek C, Kanaev A Antibacterial activity of ZnO and CuO nanoparticles against gram positive and gram negative strains. Mater Sci Eng C Mater Biol Appl (2019) 104:109968. doi:10.1016/j.msec.2019.109968
53. Agarwal H, Shanmugam V A review on anti-inflammatory activity of green synthesized zinc oxide nanoparticle: mechanism-based approach. Bioorg Chem (2020) 94:103423. doi:10.1016/j.bioorg.2019.103423
54. Nagajyothi PC, Cha SJ, Yang IJ, Sreekanth TV, Kim KJ, Shin HM Antioxidant and anti-inflammatory activities of zinc oxide nanoparticles synthesized using Polygala tenuifolia root extract. J Photochem Photobiol B Biol (2015) 146:10–7. doi:10.1016/j.jphotobiol.2015.02.008
55. Mishra PK, Mishra H, Ekielski A, Talegaonkar S, Vaidya B Zinc oxide nanoparticles: a promising nanomaterial for biomedical applications. Drug Discov Today (2017) 22(12):1825–34. doi:10.1016/j.drudis.2017.08.006
56. Wang Y, Song S, Liu J, Liu D, Zhang H ZnO-functionalized upconverting nanotheranostic agent: multi-modality imaging-guided chemotherapy with on-demand drug release triggered by pH. Angew Chem Int Ed Engl (2015) 54(2):536–40. doi:10.1002/anie.201409519
57. Eixenberger JE, Anders CB, Wada K, Reddy KM, Brown RJ, Moreno-Ramirez J, et al. Defect engineering of ZnO nanoparticles for bioimaging applications. ACS Appl Mater Interfaces (2019) 11(28):24933–44. doi:10.1021/acsami.9b01582
58. Umrani RD, Paknikar KM Zinc oxide nanoparticles show antidiabetic activity in streptozotocin-induced Type 1 and 2 diabetic rats. Nanomedicine (2014) 9(1):89–104. doi:10.2217/nnm.12.205
59. El-Gharbawy RM, Emara AM, Abu-Risha SE Zinc oxide nanoparticles and a standard antidiabetic drug restore the function and structure of beta cells in Type-2 diabetes. Biomed Pharmacother (2016) 84:810–20. doi:10.1016/j.biopha.2016.09.068
60. Manzoor U, Siddique S, Ahmed R, Noreen Z, Bokhari H, Ahmad I Antibacterial, structural and optical characterization of mechano-chemically prepared ZnO nanoparticles. PLoS One (2016) 11(5):e0154704. doi:10.1371/journal.pone.0154704
61. Alias S, Ahmad A. Synthesis of zinc oxide by sol-gel method for photoelectrochemical cells. Singapore: Springer (2014) 41–59. doi:10.1007/978-981-4560-77-1
62. Lallo da Silva B, Caetano B, Chiari-Andréo BG, Pietro RCLR, Chiavacci LA Increased antibacterial activity of ZnO nanoparticles: influence of size and surface modification. Colloids Surf B Biointerfaces (2019) 177:440–7. doi:10.1016/j.colsurfb.2019.02.013
63. Souza R, Haberbeck L, Riella H, Ribeiro D, Carciofi B Antibacterial activity of zinc oxide nanoparticles synthesized by solochemical process. Braz J Chem Eng (2019) 36(2):885–93. doi:10.1590/0104-6632.20190362s20180027
64. Khan MF, Hameedullah M, Ansari AH, Ahmad E, Lohani MB, Khan RH, et al. Flower-shaped ZnO nanoparticles synthesized by a novel approach at near-room temperatures with antibacterial and antifungal properties. Int J Nanomed (2014) 9:853–64. doi:10.2147/IJN.S47351
65. Tran N, Mir A, Mallik D, Sinha A, Nayar S, Webster TJ Bactericidal effect of iron oxide nanoparticles on Staphylococcus aureus. Int J Nanomed (2010) 5:277–83. doi:10.2147/ijn.s9220
66. Yusof NAA, Zain NM, Pauzi N Synthesis of ZnO nanoparticles with chitosan as stabilizing agent and their antibacterial properties against Gram-positive and Gram-negative bacteria. Int J Biol Macromol (2019) 124:1132–6. doi:10.1016/j.ijbiomac.2018.11.228
67. AbdElhady M Preparation and characterization of chitosan/zinc oxide nanoparticles for imparting antimicrobial and UV protection to cotton fabric. Int J Carbohydr Chem (2012) 2012(12): 840591. doi:10.1155/2012/840591
68. Liu Y, He L, Mustapha A, Li H, Hu ZQ, Lin M Antibacterial activities of zinc oxide nanoparticles against Escherichia coli O157:H7. J Appl Microbiol (2009) 107(4):1193–201. doi:10.1111/j.1365-2672.2009.04303.x
69. Sirelkhatim A, Mahmud S, Seeni A, Kaus NHM, Ann LC, Bakhori SKM, et al. Review on zinc oxide nanoparticles: antibacterial activity and toxicity mechanism. Nano-Micro Lett (2015) 7(3):219–42. doi:10.1007/s40820-015-0040-x
70. Saha R, Debanath M, Paul B, Medhi S, Saikia E Antibacterial and nonlinear dynamical analysis of flower and hexagon-shaped ZnO microstructures. Sci Rep (2020) 10(1):1–14. doi:10.1038/s41598-020-59534-x
71. Dutta RK, Nenavathu BP, Gangishetty MK, Reddy AV Studies on antibacterial activity of ZnO nanoparticles by ROS induced lipid peroxidation. Colloids Surf B Biointerfaces (2012) 94:143–50. doi:10.1016/j.colsurfb.2012.01.046
72. Xie Y, He Y, Irwin PL, Jin T, Shi X Antibacterial activity and mechanism of action of zinc oxide nanoparticles against Campylobacter jejuni. Appl Environ Microbiol (2011) 77(7):2325–31. doi:10.1128/AEM.02149-10
73. Akbar A, Sadiq M, Imran A, Muhammad N, Rehman Z, et al. Synthesis and antimicrobial activity of zinc oxide nanoparticles against foodborne pathogens Salmonella typhimurium and Staphylococcus aureus. Biocatal Agric Biotechnol (2019) 17:36–42. doi:10.1016/j.bcab.2018.11.005
74. Janaki AC, Sailatha E, Gunasekaran S Synthesis, characteristics and antimicrobial activity of ZnO nanoparticles. Spectrochim Acta A Mol Biomol Spectrosc (2015) 144:17–22. doi:10.1016/j.saa.2015.02.041
75. Li M, Zhu L, Lin D Toxicity of ZnO nanoparticles to Escherichia coli: mechanism and the influence of medium components. Environ Sci Technol (2011) 45(5):1977–83. doi:10.1021/es102624t
76. Tang S, Zheng J Antibacterial activity of silver nanoparticles: structural effects. Adv Healthc Mater (2018) 7(13):e1701503. doi:10.1002/adhm.201701503
77. Gudkov SV, Grinberg MA, Sukhov V, Vodeneev V Effect of ionizing radiation on physiological and molecular processes in plants. J Environ Radioact (2019) 202:8–24. doi:10.1016/j.jenvrad.2019.02.001
78. Rochat T, Nicolas P, Delumeau O, Rabatinová A, Korelusová J, Leduc A, et al. Genome-wide identification of genes directly regulated by the pleiotropic transcription factor Spx in Bacillus subtilis. Nucleic Acids Res (2012) 40(19):9571–83. doi:10.1093/nar/gks755
79. Tkachenko A Stress responses of bacterial cells as mechanism of development of antibiotic tolerance. Appl Biochem Microbiol (2018) 54:108–27. doi:10.1134/S0003683818020114
80. Graves , Tajkarimi M, Cunningham Q, Campbell A, Nonga H, Harrison SH, et al. Rapid evolution of silver nanoparticle resistance in Escherichia coli. Front Genet (2015) 6:42. doi:10.3389/fgene.2015.00042
81. Niño-Martínez N, Salas Orozco MF, Martínez-Castañón GA, Torres Méndez F, Ruiz F Molecular mechanisms of bacterial resistance to metal and metal oxide nanoparticles. Int J Mol Sci (2019) 20(11):2808. doi:10.3390/ijms20112808
82. Zhong L, Liu H, Samal M, Yun K Synthesis of ZnO nanoparticles-decorated spindle-shaped graphene oxide for application in synergistic antibacterial activity. J Photochem Photobiol B (2018) 183:293–301. doi:10.1016/j.jphotobiol.2018.04.048
83. Kashef N, Huang YY, Hamblin MR Advances in antimicrobial photodynamic inactivation at the nanoscale. Nanophotonics (2017) 6(5):853–79. doi:10.1515/nanoph-2016-0189
84. Nikaido H Molecular basis of bacterial outer membrane permeability revisited. Microbiol Mol Biol Rev (2003) 67(4):593–656. doi:10.1128/mmbr.67.4.593-656.2003
85. Heidary M, Zaker Bostanabad S, Amini SM, Jafari A, Ghalami Nobar M, Ghodousi A, et al. The anti-mycobacterial activity of Ag, ZnO, and Ag- ZnO nanoparticles against MDR- and XDR-Mycobacterium tuberculosis. Infect Drug Resist (2019) 12:3425–35. doi:10.2147/IDR.S221408
86. Tiwari V, Mishra N, Gadani K, Solanki PS, Shah NA, Tiwari M Mechanism of anti-bacterial activity of zinc oxide nanoparticle against carbapenem-resistant acinetobacter baumannii. Front Microbiol (2018) 9:1218. doi:10.3389/fmicb.2018.01218
87. Siddique S, Shah ZH, Shahid S, Yasmin F Preparation, characterization and antibacterial activity of ZnO nanoparticles on broad spectrum of microorganisms. Acta Chim Slov (2013) 60(3):660–5.
88. Elumalai K, Velmurugan S Green synthesis, characterization and antimicrobial activities of zinc oxide nanoparticles from the leaf extract of Azadirachta indica (L.). Appl Surf Sci (2015) 345:329–36. doi:10.1016/j.apsusc.2015.03.176
89. Dobrucka R, Dlugaszewska J, Kaczmarek M Cytotoxic and antimicrobial effects of biosynthesized ZnO nanoparticles using of Chelidonium majus extract. Biomed Microdevices (2018) 20(1):5. doi:10.1007/s10544-017-0233-9
90. Al-Hada NM, Mohamed Kamari H, Abdullah CAC, Saion E, Shaari AH, Talib ZA, et al. Down-top nanofabrication of binary (CdO)x (ZnO)1-x nanoparticles and their antibacterial activity. Int J Nanomed (2017) 12:8309–23. doi:10.2147/IJN.S150405
91. Divya M, Vaseeharan B, Abinaya M, Vijayakumar S, Govindarajan M, Alharbi NS, et al. Biopolymer gelatin-coated zinc oxide nanoparticles showed high antibacterial, antibiofilm and anti-angiogenic activity. J Photochem Photobiol B Biol (2017) 178:211–8. doi:10.1016/j.jphotobiol.2017.11.008
92. Dobrucka R, Długaszewska J Biosynthesis and antibacterial activity of ZnO nanoparticles using Trifolium pratense flower extract. Saudi J Biol Sci (2016) 23(4):517–23. doi:10.1016/j.sjbs.2015.05.016
93. Chauhan A, Verma R, Kumari S, Sharma A, Shandilya P, Li X, et al. Photocatalytic dye degradation and antimicrobial activities of Pure and Ag-doped ZnO using Cannabis sativa leaf extract. Sci Rep (2020) 10(1):1–16. doi:10.1038/s41598-020-64419-0
94. Precious Ayanwale A, Reyes-López SY ZrO2-ZnO nanoparticles as antibacterial agents. ACS Omega (2019) 4(21):19216–24. doi:10.1021/acsomega.9b02527
95. Navale G, Thripuranthaka M, Late D, Shinde S Antimicrobial activity of ZnO nanoparticles against pathogenic bacteria and fungi. JSM Nanotechnol Nanomed (2015) 3(1):1033.
96. Qi K, Xing X, Mengyu L, Wang Q, Liu S, Lin H, et al. Transition metal doped ZnO nanoparticles with enhanced photocatalytic and antibacterial performances: Experimental and DFT studies. Ceram Int (2019) 46(2):1494–502. doi:10.1016/j.ceramint.2019.09.116
97. Jayaseelan C, Rahuman AA, Kirthi AV, Marimuthu S, Santhoshkumar T, Bagavan A, et al. Novel microbial route to synthesize ZnO nanoparticles using Aeromonas hydrophila and their activity against pathogenic bacteria and fungi. Spectrochim Acta A Mol Biomol Spectrosc (2012) 90:78–84. doi:10.1016/j.saa.2012.01.006
98. Raja A, Ashokkumar S, Pavithra Marthandam R, Jayachandiran J, Khatiwada CP, Kaviyarasu K, et al. Eco-friendly preparation of zinc oxide nanoparticles using Tabernaemontana divaricata and its photocatalytic and antimicrobial activity. J Photochem Photobiol B (2018) 181:53–8. doi:10.1016/j.jphotobiol.2018.02.011
99. Kumar K, Mandal B, Naidu E, Sinha M, Kumar S, Reddy P Synthesis and characterization of flower shaped Zinc Oxide nanostructures and its antimicrobial activity. Spectrochim Acta Part A Molecular and Biomolecular Spectroscopy (2012) 104C:171–4. doi:10.1016/j.saa.2012.11.025
100. Jin T, Sun D, Su JY, Zhang H, Sue HJ Antimicrobial efficacy of zinc oxide quantum dots against Listeria monocytogenes, Salmonella Enteritidis, and Escherichia coli O157:H7. J Food Sci (2009) 74(1):M46–52. doi:10.1111/j.1750-3841.2008.01013.x
101. Saliani M, Jalal R, Kafshdare Goharshadi E Effects of pH and temperature on antibacterial activity of zinc oxide nanofluid against Escherichia coli O157: H7 and Staphylococcus aureus. Jundishapur J Microbiol (2015) 8(2):e17115. doi:10.5812/jjm.17115
102. Emami-Karvani Z, Chehrazi P Antibacterial activity of ZnO nanoparticle on gram-positive and gram-negative bac-teria. Afr J Microbiol Res (2011) 5(18):1368–73. doi:10.5897/AJMR10.159
103. Zhang L, Jiang Y, Ding Y, Povey M, York D Investigation into the antibacterial behaviour of suspensions of ZnO nanoparticles (ZnO nanofluids). J Nanoparticle Res (2007) 9:479–89. doi:10.1007/s11051-006-9150-1
104. Zare M, Namratha K, Alghamdi S, Mohammad YHE, Hezam A, Zare M, et al. Novel green biomimetic approach for synthesis of ZnO-Ag nanocomposite; antimicrobial activity against food-borne pathogen, biocompatibility and solar photocatalysis. Sci Rep (2019) 9:8303. doi:10.1038/s41598-019-44309-w
105. Senthilkumar N, Nandhakumar E, Priya P, Soni D, Vimalan M, Vetha Potheher I Synthesis, anti-bacterial, anti-arthritic, anti-oxidant and in-vitro cytotoxicity activities of ZnO nanoparticles using leaf extract of Tectona grandis (L.). New J Chem (2017) 41(18):10347–56. doi:10.1039/C7NJ02664A
106. Aiswarya Devi S, Harshiny M, Udaykumar S, Gopinath P, Matheswaran M Strategy of metal iron doping and green-mediated ZnO nanoparticles: dissolubility, antibacterial and cytotoxic traits. Toxicol Res (2017) 6(6):854–65. doi:10.1039/c7tx00093f
107. Zare M, Namratha K, Byrappa K, Surendra D, Yallappa S, Hungund B Surfactant assisted solvothermal synthesis of ZnO nanoparticles and study of their antimicrobial and antioxidant properties. J Mater Sci Technol (2018) 34(6):1035–43. doi:10.1016/j.jmst.2017.09.014
108. Bai X, Li L, Liu H, Tan L, Liu T, Meng X Solvothermal synthesis of ZnO nanoparticles and anti-infection application in vivo. ACS Appl Mater Interfaces (2015) 7(2):1308–17. doi:10.1021/am507532p
109. Rekha K, Nirmala M, Nair MG, Anukaliani A Structural, optical, photocatalytic and antibacterial activity of zinc oxide and manganese doped zinc oxide nanoparticles. Phys B Condens Matter (2010) 405(15):3180–5. doi:10.1016/j.physb.2010.04.042
110. Khan MF, Ansari AH, Hameedullah M, Ahmad E, Husain FM, Zia Q, et al. Sol-gel synthesis of thorn-like ZnO nanoparticles endorsing mechanical stirring effect and their antimicrobial activities: potential role as nano-antibiotics. Sci Rep (2016) 6:27689. doi:10.1038/srep27689
111. Siddiquah A, Hashmi S, Mushtaq S, Renouard S, Blondeau JP, Abbasi R, et al. Exploiting in vitro potential and characterization of surface modified Zinc oxide nanoparticles of Isodon rugosus extract: their clinical potential towards HepG2 cell line and human pathogenic bacteria. EXCLI J (2018) 17:671–87. doi:10.17179/excli2018-1327
112. Lingaraju K, Raja Naika H, Manjunath K, Basavaraj R, Nagabhushana H, Nagaraju G, et al. Biogenic synthesis of zinc oxide nanoparticles using Ruta graveolens (L.) and their antibacterial and antioxidant activities. Appl Nanosci (2016) 6(5):703–10. doi:10.1007/s13204-015-0487-6
113. Ehsan S, Sajjad M Bioinspired synthesis of zinc oxide nanoparticle and its combined efficacy with different antibiotics against multidrug resistant bacteria. J Biomater Nanobiotechnol (2017) 8(2):159–75. doi:10.4236/jbnb.2017.82011
114. Prachi A, Patel R, Singh N, Negi D, Rawat S Green synthesis of zinc oxide nanoparticles using Rubia Cordifolia root extract against different bacterial pathogens. Indo American journal of farmaceutical research (2017) 07(09):759–65. doi:10.5281/zenodo.1036347
115. Pant H, Pant B, Sharma R, Amarjargal A, Kim H, Park C, et al. Antibacterial and pho-tocatalytic properties of Ag/TiO2/ZnO nano-owers pre-pared by facile one-pot hydrothermal process. Ceram Int (2013) 39:1503–10. doi:10.1016/j.cera-mint.2012.07.097
116. Shalumon KT, Anulekha KH, Nair SV, Nair SV, Chennazhi KP, Jayakumar R Sodium alginate/poly(vinyl alcohol)/nano ZnO composite nanofibers for antibacterial wound dressings. Int J Biol Macromol (2011) 49(3):247–54. doi:10.1016/j.ijbiomac.2011.04.005
117. Kayani Z, Abbas E, Saddiqe Z, Riaz S, Naseem S Photocatalytic, antibacterial, optical and magnetic properties of Fe-doped ZnO nano-particles prepared by sol-gel. Mater Sci Semicond Process (2018) 88:109–19. doi:10.1016/j.mssp.2018.08.003
118. Sharma D, Rajput J, Kaith B, Kaur M, Sharma S Synthesis of ZnO nanoparticles and study of their antibacterial and antifungal properties. Thin Solid Films (2010) 519(3):1224–9. doi:10.1016/j.tsf.2010.08.073
119. Al-Jumaili A, Mulvey P, Kumar A, Prasad K, Bazaka K, Warner J, et al. Eco-friendly nanocomposites derived from geranium oil and zinc oxide in one step approach. Sci Rep (2019) 9:5973. doi:10.1038/s41598-019-42211-z
120. Mehmood S, Rehman MA, Ismail H, Mirza B, Bhatti AS Significance of postgrowth processing of ZnO nanostructures on antibacterial activity against gram-positive and gram-negative bacteria. Int J Nanomed (2015) 10(1):4521–33. doi:10.2147/IJN.S83356
121. Nair S, Sasidharan A, Divya Rani VV, Menon D, Nair S, Manzoor K, et al. Role of size scale of ZnO nanoparticles and microparticles on toxicity toward bacteria and osteoblast cancer cells. J Mater Sci Mater Med (2009) 20(Suppl 1):S235–41. doi:10.1007/s10856-008-3548-5
122. Yousefi M, Dadashpour M, Hejazi M, Hasanzadeh M, Behnam B, de la Guardia M, et al. Anti-bacterial activity of graphene oxide as a new weapon nanomaterial to combat multidrug-resistance bacteria. Mater Sci Eng C Mater Biol Appl (2017) 74:568–81. doi:10.1016/j.msec.2016.12.125
123. Kavitha T, Gopalan AI, Lee KP, Park SY Glucose sensing, photocatalytic and antibacterial properties of graphene–ZnO nanoparticle hybrids. Carbon (2012) 50(8):2994–3000. doi:10.1016/j.carbon.2012.02.082
124. Leung YH, Chan CM, Ng AM, Chan HT, Chiang MW, Djurišić AB, et al. Antibacterial activity of ZnO nanoparticles with a modified surface under ambient illumination. Nanotechnology (2012) 23(47):475703. doi:10.1088/0957-4484/23/47/475703
125. Bai X, Li L, Liu H, Tan L, Liu T, Meng X Solvothermal synthesis of ZnO nanoparticles and anti-infection application in Vivo. ACS Appl Mater Interfaces (2020) 7(2):1308–17. doi:10.1021/am5075310.1021/am507532p
126. Selim YA, Azb MA, Ragab I, H M Abd El-Azim M Green synthesis of zinc oxide nanoparticles using aqueous extract of deverra tortuosa and their cytotoxic activities. Sci Rep (2020) 10:3445. doi:10.1038/s41598-020-60541-1
127. Vidya C, Hiremath S, Chandraprabha MN, Lourdu Antonyraj MA, Venu Gopal I, Jain A, et al. Green synthesis of ZnO nanoparticles by Calotropis gigantea. Int J Curr Eng Technol (2013) 1:118–20.
Keywords: nanoparticles, zinc oxide, antibiotics, antibacterial, bacteriostatic, bactericidal, fungicidal, green synthesis
Citation: Gudkov SV, Burmistrov DE, Serov DA, Rebezov MB, Semenova AA and Lisitsyn AB (2021) A Mini Review of Antibacterial Properties of ZnO Nanoparticles. Front. Phys. 9:641481. doi: 10.3389/fphy.2021.641481
Received: 15 December 2020; Accepted: 19 January 2021;
Published: 11 March 2021.
Edited by:
Nikolai F. Bunkin, Bauman Moscow State Technical University, RussiaReviewed by:
Muthu Thiruvengadam, Konkuk University, South KoreaVladimir Ivanov, Institute of Theoretical and Experimental Biophysics (RAS), Russia
Copyright © 2021 Gudkov, Burmistrov, Serov, Rebezov, Semenova and Lisitsyn. This is an open-access article distributed under the terms of the Creative Commons Attribution License (CC BY). The use, distribution or reproduction in other forums is permitted, provided the original author(s) and the copyright owner(s) are credited and that the original publication in this journal is cited, in accordance with accepted academic practice. No use, distribution or reproduction is permitted which does not comply with these terms.
*Correspondence: Sergey V. Gudkov, c19tYWthcml5QHJhbWJsZXIucnU=