- 1Institut für Neurowissenschaften und Medizin, INM-5: Nuklearchemie, Forschungszentrum Jülich, Jülich, Germany
- 2Department of Physics, Government College University Lahore, Lahore, Pakistan
Nuclear data are important for production and medical application of a radionuclide. This brief review concentrates on nuclear reaction cross-section data. The availability of standardized nuclear data for accelerator-based production of medical radionuclides is outlined. Some new directions in radionuclide applications, for example, theranostic approach, bimodal imaging, and radionuclide targeted therapy, are considered and the status of relevant nuclear data is discussed. The current trends in nuclear data research using accelerators are elaborated. The increasing significance of intermediate energy accelerators in production of therapeutic radionuclides is emphasized.
Introduction
Radionuclides find application in medicine, both in diagnosis and internal radiotherapy. Diagnostic investigations are carried out using short-lived radionuclides which emit either a single or major γ-ray of energy between 100 and 250 keV or a positron, the former in single photon emission computed tomography (SPECT) and latter in positron emission tomography (PET). In internal radionuclide therapy, on the other hand, radionuclides emitting low-range highly ionizing radiation, that is, α- or β–-particles, conversion, and/or Auger electrons are used. A detailed knowledge of decay properties of a radionuclide is, therefore, essential to decide about its application. Furthermore, the radionuclidic purity and specific activity (defined as the radioactivity per unit mass of the material) play an important role in its medical application. Radionuclidic purity reduces the radiation dose due to impurities and higher specific activity ensures that the biological equilibrium is not disturbed and imaging is carried out at real molecular level. Those two parameters are controlled by knowledge of nuclear reaction cross sections, especially in production of radionuclides via charged particle-induced reactions [1, 2]. The level and type of radionuclidic impurity varies with the chosen reaction route and the projectile energy range effective in the target.
The positron emitters are generally produced at small-sized cyclotrons, the number of which worldwide is now increasing to about 1200 [3]. Attempts are presently also underway to produce several therapeutic radionuclides at cyclotrons/accelerators, using either the charged particle beam or secondary radiation, that is, neutrons and photons, generated at accelerators (for recent reviews, cf [4, 5]). Nuclear data play a continuing role in the development of radionuclides for applications. In this brief review, the availability of standardized data for production of medical radionuclides using accelerators is outlined, some new directions in radionuclide applications are discussed, and the current trends in nuclear data research are described.
Availability and Status of Standardized Data
Experimental data are compiled in the international file EXFOR, managed by the IAEA. Radionuclides to be used for a suitable modality of the medical procedure demand well-established data. The decay data of medical radionuclides are compiled and constantly updated in the MIRD file of the Society of Nuclear Medicine (SNM) of United States. Decay data of all radionuclides are available in the Evaluated Nuclear Structure and Decay Data File (ENSDF) and neutron-induced reaction cross-section data in the Evaluated Nuclear Data File (ENDF/B-VIII.0). In contrast, the evaluation methodology for charged particle-induced reaction cross sections started developing only about 2 decades ago [2] under the umbrella of IAEA in the form of coordinated research projects (CRPs), with major contributions from Jülich, Debrecen, Brussels, and Obninsk. To date three CRPs have been completed. The first two were related to radionuclides commonly used in diagnosis (IAEA-TECDOC-1211, 2001) and internal therapy (IAEA-Technical Report-473, 2011). In the third CRP, some updates were done but also the production data of some novel radionuclides were standardized [6–8]. The initial work was rather empirical. Later, however, strong application of nuclear models was built in. All modern calculation codes are based on the statistical model, taking into account angular momentum, nuclear structure, and level density effects, and also incorporate pre-compound emission contributions. Each code reproduces the experimental data to a certain degree of success. The nuclear model calculations performed using the codes EMPIRE 3.2 and TALYS 1.95 are generally very successful in reproducing the experimental data up to about 50 MeV, if only a few nucleons are emitted. Deviations are observed when emission of many nucleons and complex particles is involved. The adjustable parameters of the nuclear model codes are fitted within their prescribed limits to reproduce the experimental data in the process of validation and evaluation. All evaluated data are available on the website of the IAEA [9]. Using theory-aided evaluation, some work has been also carried out outside the abovementioned CRPs (see for example [10–14]). The evaluation efforts have provided reliable standardized data for accelerator-based radionuclides routinely used in patient care (PET/SPECT imaging and internal radiotherapy), produced either directly or obtained through positron-emitting generator systems 68Ge/68Ga and 82Sr/82Rb. Furthermore, data for a few novel and less commonly used radionuclides, for example, 64Cu, 89Zr, 124I, 103Pd, and 211At, have also been standardized. Standardized data are also available for the 100Mo(p,2n)99mTc reaction [10]. This is a very promising route for accelerator-based production of the most commonly used SPECT radionuclide 99mTc, which is generally available via the fission-produced 99Mo/99mTc generator system. However, further extensive work is needed to settle the question of impurities in cyclotron production of this radionuclide. Similarly, for emerging radionuclides more experimental and evaluation work is necessary (see below).
New Directions in Radionuclide Applications and Relevant Nuclear Data
For investigating slow metabolic processes, about 25 longer lived metallic positron emitters, termed as “nonstandard” positron emitters, have been developed [15]. Similarly, several metallic radionuclides emitting low-energy corpuscular radiation are in development for internal therapy (cf [1, 16]). In parallel, considerable chemical research has led to enhanced possibilities of labeling monoclonal antibodies (mAbs) and other versatile organo-metallic chemical complexes with radionuclides for targeted therapy (for reviews cf [17, 18]). Based on those advances, applications of radionuclides are enhancing today in the following directions:
i) Radiolabeled monoclonal antibodies
ii) Peptide receptor radiotherapy
iii) Small molecules
iv) Theranostic approach
v) Bimodal imaging
vi) Radioactive nanoparticles
Radiolabeled monoclonal antibodies: Monoclonal antibodies (mAbs) labeled with radionuclides are used in diagnosis and therapy of tumors. The application of mAbs labeled with positron emitters is called immunoPET (cf. [19]). To date, the most widely used radionuclide for this purpose is 89Zr, but also 64Cu has found application in preclinical studies. For immunotherapy, 90Y, 111In, and 225Ac are potential candidates, for example, [90Y]ibritumomab tiuxetan (Zevalin).
Peptide receptor radiotherapy (PRRT) makes use of peptide-based radiopharmaceuticals which can target different receptor systems like somatostatin receptors (SSTR), integrins, chemokine receptors, or the prostate-specific membrane antigen (PSMA). The most common approach uses octreotide derivatives labeled with 90Y or 177Lu to treat neuroendocrine tumors effectively, that is, [90Y]Y-DOTATOC and [177Lu]Lu-DOTATATE. Also [225Ac]Ac-DOTATATE is under study. A further important molecular target is the chemokine receptor-4 CXCR-4. This can be targeted with Pentixafor and studies with 177Lu-labeled Pentixafor in cancer patients are underway.
Small molecules: Besides radioiodinated pharmaceuticals, different radiometal-labeled small molecules have been developed to treat oncological diseases, for example, PSMA-617, a urea-based derivative with excellent affinity to PSMA overexpressing prostate cancer tumor cells. After binding to the target, the molecule is internalized and facilitates eradication of the tumor cells. Therefore [225Ac]Ac-PSMA-617 and [177Lu]Lu-PSMA-617 are under different clinical trials.
Theranostic approach entails a combination of diagnosis (molecular imaging) and internal radionuclide therapy (molecular targeted treatment). It makes use of two radionuclides of the same element in the same chemical form, a positron emitter which allows quantitative diagnosis via PET and a therapeutic nuclide. Originally, the pair 86Y/90Y was developed (cf. [20]), but today several other theranostic pairs are also known, for example, 44gSc/47Sc, 64Cu/67Cu, 124I/131I, and 152Tb/161Tb (for review cf [4]). Two other concepts also exist: 1) use of an analog pair of trivalent metallic radionuclides, that is, a positron emitter (44gSc or 68Ga) and a β−- (177Lu) or an α-emitter (225Ac). 2) use of a single radionuclide, emitting a β−- or α-particle as well as a low-energy γ-ray, which could be utilized for SPECT measurement to deliver data for dosimetry. Examples are 47Sc, 67Cu, 177Lu, and 186Re for β−-therapy and 211At, 223Ra, 225Ac, 213Bi, 212Pb/212Bi generator, and 149Tb for targeted alpha therapy (TAT). Several other alpha-particle emitters are also under consideration (cf [21]).
Bimodal imaging involves a combination of two organ-imaging techniques, for example, PET and magnetic resonance imaging (MRI) (for review cf [22]). From the viewpoint of PET, the major focus is on the elements Mn and Gd which are important contrast agents in MRI. The positron-emitting radionuclide 52gMn is of great current interest (cf [23, 24]). For Gd, no positron-emitting radionuclide is available and the use of 68Ga-labeled Gd(III) complexes has been proposed.
Radioactive nanoparticles in medicine constitute a long-term perspective, provided the stability and toxicity problems are overcome. In animal and preclinical studies, considerable success has been reported (for review cf [25, 26]) but application in humans has yet to be demonstrated. The radionuclides 64Cu and 68Ga are widely used positron emitters for surface labeling of nanoparticles. The longer lived 52gMn and 89Zr are also of great interest. For therapy, 186Re and 225Ac are considered to be very useful.
In the abovementioned new applications seven positron emitters, namely 44gSc (T1/2 = 3.9 h), 52gMn (T1/2 = 5.6 d), 64Cu (T1/2 = 12.7 h), 68Ga (T1/2 = 1.13 h), 86Y (T1/2 = 14.7 h), 89Zr (T1/2 = 78.4 h), and 124I (T1/2 = 4.18 d) are in great demand. Similarly, eight therapeutic radionuclides, namely 47Sc (T1/2 = 3.35 d), 67Cu (T1/2 = 2.58 d), 90Y (T1/2 = 2.7 d), 117mSn (T1/2 = 13.6 d), 177Lu (T1/2 = 6.65 d), 186Re (T1/2 = 3.78 d), 223Ra (T1/2 = 11.4 d), and 225Ac (T1/2 = 10.0 d) are of great interest. For each radionuclide, the stringent criteria of purity and specific activity must be met.
The production of the listed positron emitters is generally carried out via the low-energy (p,n) reaction on a highly enriched solid target isotope. In the case of 68Ga, the generator route is more commonly used. The status of the available cross-section data of the abovementioned positron emitters was reviewed and found to be generally good (cf. [1, 15]), except for 86Y where considerable discrepancy existed.
In contrast to positron emitters, the therapeutic radionuclides 90Y and 177Lu are routinely produced using nuclear reactors. For the six other therapeutic radionuclides, it was shown (cf. [8, 16]) that the reactor production methods are not sufficient. For 47Sc and 67Cu, the required radionuclidic and chemical purity is not achieved and for 186Re and 117mSn, the specific activity is too low. The supply of 225Ac and 223Ra via reactor route is limited. Efforts are therefore underway to produce those radionuclides at accelerators, 186Re at a small cyclotron and others at intermediate energy accelerators.
Current Nuclear Data Activities
Nuclear modeling and theory-aided evaluations are continuing. The emphasis is, however, on new experimental studies; some of the most recent examples are given below.
Low-energy charged particle beam: Low-energy medical cyclotrons (EP ≤ 18 MeV; Ed ≤ 9 MeV) are mainly utilized for the production of the standard positron emitters (11C, 13N, 15O, and 18F) using gas and liquid targets. Since solid targetry is not available, a new methodology for the production of some nonstandard positron emitters via the (p,n) reaction at those cyclotrons has emerged. It makes use of “solution targets”, especially for 44gSc, 64Cu, 68Ga, 86Y, 89Zr, and 94mTc (for review cf. [27]). The product yield is low but sufficient for local use. This approach has, however, put an extra demand on chemical purification of the product as well as on the accuracy of the cross-section data near the threshold of the reaction (cf. [28, 29]). To improve the cross-section data of the 86Sr(p,n)86Y reaction, an accurate measurement was very recently completed [30]. The new results agree with the TALYS calculation and the discrepancy has been solved.
Intermediate energy charged particle beam: In the energy range up to 30 MeV, several routinely used radionuclides are conveniently produced, especially the SPECT radionuclides 67Ga, 111In, 123I, and 201Tl. At a higher proton energy up to 100 MeV, several radionuclides could be produced via (p,x) reactions, where x stands for multiparticle emission. Such accelerator facilities are now available at several places, for example Nantes (France), Legnaro (Italy), Moscow (Russia), Cape Town (South Africa), Brookhaven (United States), and Los Alamos (United States). A recent example is the measurement of the excitation function of the 75As (p,4n)72Se reaction [31] for the production of 72Se which is useful for preparing the β+-emitting generator system 72Se/72As.
The intermediate energy range, however, is now being utilized more extensively for the production of therapeutic radionuclides. A recent cross-section measurement on the reaction 70Zn(p,α+2p2n)67Cu [32] is very interesting. Although the experimental data are not reproduced by the model calculation (due to complexity of reaction and energy range), they are of great technical value. In Figure 1 the yields of 67Cu are shown. They were calculated from the excitation functions of relevant charged-particle–induced reactions using the standard activation equation [1]. It is an updated version of the diagram given earlier [16]. In order to minimize the impurities of 64Cu (radioactive) and 65Cu (stable, decreasing the specific activity of 67Cu), the energy range Ep = 80 → 30 MeV was found to be very suitable for the production of 67Cu via the 68Zn(p,2p) process. From the new results, it is concluded that protons of energy up to about 80 MeV are very suitable for the production of 67Cu not only when isotopically enriched 68Zn is used as target material [16, 33] but also if enriched 70Zn would be the target, provided good radionuclidic purity is achieved. For production of the β−-emitter 47Sc (for review see [4]), neither the intermediate energy reaction 48Ti(p,2p)47Sc investigated earlier nor the 51V(p,αp)47Sc process studied recently [34] appears to be successful because of the high level of the radionuclidic impurity 46Sc. With regard to the conversion electron emitter 117mSn, the production route 116Cd(α,3n)117mSn was established [11]. Yet, a new measurement suggests that the nuclear process natSb(p,xn)117mSn over the proton energy range of 30–90 MeV could also be potentially useful [35]. Regarding the α-particle emitters 225Ac and 223Ra, the present emphasis is on their production through proton-induced reactions on 232Th, either directly or via the indirect routes
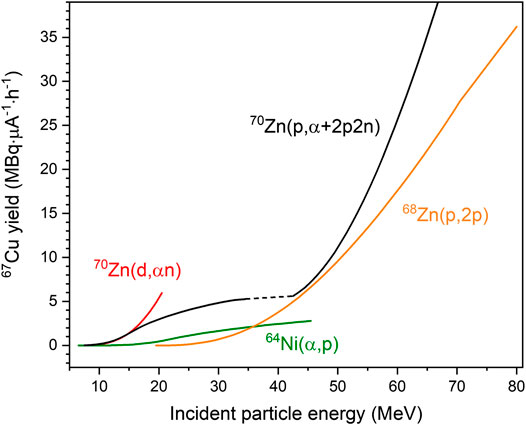
FIGURE 1. Integral yield of 67Cu, calculated from the excitation function of each reaction for an irradiation time of 1 h, shown as a function of incident particle energy. The dashed part of the curve for the 70Zn(p,x)67Cu process is an extrapolation because no data exist.
It should be mentioned that besides protons, intermediate energy deuterons and alpha-particles are also potentially useful for production of a few special radionuclides, for example, 103Pd and 186Re using deuterons and 211At using alpha-particles. Furthermore, in recent years it has been demonstrated that the high-spin isomers of a few radionuclides, for example, 117mSn and 193mPt, can be advantageously produced using alpha-particles [36]. They are useful in therapy because they emit low-energy conversion and Auger electrons. In general, however, the use of protons is preferred due to their easier availability and the resulting higher yields of the products.
High-energy charged-particle beam: The spallation process with high-energy protons (Ep > 500 MeV) combined with on-line mass separation was utilized at CERN in cooperation with the Paul Scherrer Institute (PSI) to produce some exotic radionuclides in the region of rare earths, especially 149Tb (T1/2 = 4.1 h) and 152Tb (T1/2 = 17.5 h). The former is a unique low-energy α-particle emitter suited for TAT. The latter is a theranostic PET partner. A new measurement gives cross sections for the formation of several terbium radioisotopes in the spallation of tantalum as a function of proton energy [37]. For more general use of the two radionuclides, however, development of alternative production methods, preferably using intermediate energy protons, are called for. Preliminary cross-section measurements on the reactions 155Gd(p,4n)152Tb and 152Gd(p,4n)149Tb are promising [38].
Use of photons and fast neutrons: The use of electron linear accelerator (LINAC) to deliver high-energy photons for the production of the therapeutic radionuclides 47Sc and 67Cu via the 48Ti (γ,p)47Sc and 68Zn (γ,p)67Cu reactions, respectively, is presently under investigation. The excitation functions are known (cf. IAEA-TECDOC-1178, 2000) but further improvement in the data is needed. The production for preclinical tests has been reported [39, 40]. In practice, however, GBq amounts of 67Cu have been produced whereas the methodology for 47Sc production is still developing. Some on-going nuclear data work deals with determination of spectrum-averaged cross sections and production yields [41, 42]. The production of 225Ac via the
The use of accelerator-generated fast neutrons is also under investigation. In particular, a 30 or 40 MeVd(Be) or d(C) breakup neutron source is considered to be suitable for the production of a few radionuclides via the (n,p) or (n,np) reaction [43]. The estimated integral cross sections amount to a few mb [5]. The spallation neutrons also appear to be interesting for the formation of a few radionuclides [44]. However, extensive further nuclear data work is called for.
Concluding Remarks
Nuclear data play an important role in the production and medical application of accelerator-based radionuclides. The data for routine production of radionuclides for patient care have been standardized. For development of novel radionuclides, however, continuing data research is needed. The present thrust in medical application of radionuclides is directed toward PET studies using metallic positron emitters as well as toward targeted radionuclide therapy, preferably applying the theranostic approach. With tremendous developments in antibody labeling and organo-metallic complex formation chemistry, a big impetus has come to the field of theranostics. This is leading to an enhanced interest in accelerator-based production of radionuclides. The nonstandard positron emitters are produced at small-sized cyclotrons and now development of production methodologies of many therapeutic radionuclides is shifting from nuclear reactors to intermediate energy accelerators. Another strategy is to utilize hard photons from powerful LINACs or fast neutrons from intermediate energy accelerators. With enhancing interest in versatile accelerators to produce novel medical radionuclides, the need of relevant nuclear data research is continuing.
Author Contributions
SQ developed the concept. MH elaborated the section on standardization of data. IS reviewed experimental data and calculated radionuclide yields. BN advised on new directions in medical applications. All authors contributed to the writing of the article and approved the submitted version.
Conflict of Interest
The authors declare that the research was conducted in the absence of any commercial or financial relationships that could be construed as a potential conflict of interest.
References
1. Qaim SM Nuclear data for production and medical application of radionuclides: present status and future needs. Nucl Med Biol (2017) 44:31–49. doi:10.1016/j.nucmedbio.2016.08.016
2. Qaim SM Medical radionuclide production: science and technology. Berlin/Boston: Walter de Gruyter (2019) 978-3-11-060156-5..
3. Synowiecki MA, Perk LR, Nijsen JFW Production of novel diagnostic radionuclides in small medical cyclotrons. EJNMMI Radiopharmacy Chem (2018) 3(3):1–25. doi:10.1186/s41181-018-0038-z
4. Qaim SM, Scholten B, Neumaier B New developments in the production of theranostic pairs of radionuclides. J Radioanal Nucl Chem (2018) 318:1493–509. doi:10.1007/s10967-018-6238-x
5. Qaim SM Theranostic radionuclides: recent advances in production methodologies. J Radioanal Nucl Chem (2019) 322:1257–66. doi:10.1007/s10967-019-06797-y
6. Tárkányi FT, Ignatyuk AV, Hermanne A, Capote R, Carlson BV, Engle JW, et al. Recommended nuclear data for medical radioisotope production: diagnostic gamma emitters. J Radioanal Nucl Chem (2019) 319:487–531. doi:10.1007/s10967-018-6142-4
7. Tárkányi FT, Ignatyuk AV, Hermanne A, Capote R, Carlson BV, Engle JW, et al. Recommended nuclear data for medical radioisotope production: diagnostic positron emitters. J Radioanal Nucl Chem (2019) 319:533–666. doi:10.1007/s10967-018-6380-5
8. Engle JW, Ignatyuk AV, Capote R, Carlson BV, Hermanne A, Kellett MA, et al. Recommended nuclear data for the production of selected therapeutic radionuclides. Nucl Data Sheets (2019) 155:56–74. doi:10.1016/j.nds.2019.01.003
9.IAEA. Nuclear data section (2020) Available from: https://www-nds.iaea.org/relnsd/vcharthtml/MEDVChart.html (Accessed December 1, 2020).
10. Qaim SM, Sudár S, Scholten B, Koning AJ, Coenen HH Evaluation of excitation functions of 100Mo(p,d+pn)99Mo and 100Mo (p,2n)99mTc reactions: estimation of long-lived Tc-impurity and its implication on the specific activity of cyclotron-produced 99mTc. Appl Radiat Isot (2014) 85:101–13. doi:10.1016/j.apradiso.2013.10.004
11. Aslam MN, Zubia K, Qaim SM Nuclear model analysis of excitation functions of α-particle induced reactions on in and Cd up to 60 MeV with relevance to the production of high specific activity 117m Sn. Appl Radiat Isot (2018) 132:181–8. doi:10.1016/j.apradiso.2017.12.002
12. Ali SKI, Khandaker MU, Kassim HA Evaluation of production cross-sections for 186 Re theranostic radionuclide via charged-particle induced reactions on tungsten. Appl Radiat Isot (2018) 135:239–50. doi:10.1016/j.apradiso.2018.01.035
13. Ali W, Tashfeen M, Hussain M Evaluation of nuclear reaction cross sections via proton induced reactions on 55Mn for the production of 52Fe: a potential candidate for theranostic applications. Appl Radiat Isot (2019) 144:124–9. doi:10.1016/j.apradiso.2018.11.016
14. Amjed N, Wajid AM, Ahmad N, Ishaq M, Aslam MN, Hussain M, et al. Evaluation of nuclear reaction cross sections for optimization of production of the important non-standard positron emitting radionuclide 89Zr using proton and deuteron induced reactions on 89Y target. Appl Radiat Isot (2020) 165:109338. doi:10.1016/j.apradiso.2020.109338
15. Qaim SM, Scholten B, Spahn I, Neumaier B Positron-emitting radionuclides for applications, with special emphasis on their production methodologies for medical use. Radiochim Acta (2019) 107:1011–26. doi:10.1515/ract-2019-3154
16. Qaim SM, Spahn I Development of novel radionuclides for medical applications. J Label Compd Radiopharm (2018) 61:126–40. doi:10.1002/jlcr.3578
17. Aluicio-Sarduy E, Ellison PA, Barnhart TE, Cai W, Nickles RJ, Engle JW PET radiometals for antibody labeling. J Label Compd Radiopharm (2018) 61:636–51. doi:10.1002/jlcr.3607
18. Kostelnik TI, Orvig C Radioactive main group and rare earth metals for imaging and therapy. Chem Rev (2019) 119:902–56. doi:10.1021/acs.chemrev.8b00294
19. Wei W, Rosenkrans ZT, Liu J, Huang G, Luo Q-Y, Cai W ImmunoPET: concept, design, and applications. Chem Rev (2020) 120(8):3787–851. doi:10.1021/acs.chemrev.9b00738
20. Rösch F, Herzog H, Qaim S The beginning and development of the theranostic approach in nuclear medicine, as exemplified by the radionuclide pair 86Y and 90Y. Pharmaceuticals (2017) 10:56. doi:10.3390/ph10020056
21. Ferrier MG, Radchenko V, Wilbur DS Radiochemical aspects of alpha emitting radionuclides for medical application. Radiochim Acta (2019) 107:1065–85. doi:10.1515/ract-2019-0005
22. Picchio M, Pampaloni MH Current status and future perspectives of PET/MRI hybrid imaging. Clin Transl Imaging (2017) 5:79–81. doi:10.1007/s40336-016-0215-6
23. Lewis CM, Graves SA, Hernandez R, Valdovinos HF, BarnhartCai TEWB, Cai W, et al. 52Mn production for PET/MRI tracking of human stem cells expressing divalent metal transporter 1 (DMT1). Theranostics (2015) 5:227–39. doi:10.7150/thno.10185
24. Brandt MR, Vanasschen C, Ermert J, Coenen HH, Neumaier B 52g/55Mn-Labelled CDTA-based trimeric complexes as novel bimodal PET/MR probes with high relaxivity. Dalton Trans (2019) 48:3003–8. doi:10.1039/c8dt04996c
25. Farzin L, Sheibani S, Moassesi ME, Shamsipur M An overview of nanoscale radionuclides and radiolabeled nanomaterials commonly used for nuclear molecular imaging and therapeutic functions. J Biomed Mater Res (2019) 107:251–85. doi:10.1002/jbm.a.36550
26. Ge J, Zhang Q, Zeng J, Gu Z, Gao M Radiolabeling nanomaterials for multimodality imaging: new insights into nuclear medicine and cancer diagnosis. Biomaterials (2020) 228:119553. doi:10.1016/j.biomaterials.2019.119553
27. Pandey MK, DeGrado TR Cyclotron production of PET radiometals in liquid targets: aspects and prospects. Curr Radiopharm (2020) 13:1–15. doi:10.2174/1874471013999200820165734
28. Uddin MS, Chakraborty AK, Spellerberg S, Shariff MA, Das S, Rashid MA, et al. Experimental determination of proton induced reaction cross sections on natNi near threshold energy. Radiochim Acta (2016) 104:305–14. doi:10.1515/ract-2015-2527
29. Carzaniga TS, Auger M, Braccini S, Bunka M, Ereditato A, Nesteruk KP, et al. Measurement of 43Sc and 44Sc production cross-section with an 18 MeV medical PET cyclotron. Appl Radiat Isot (2017) 129:96–102. doi:10.1016/j.apradiso.2017.08.013
30. Uddin MS, Scholten B, Basunia MS, Sudár S, Spellerberg S, Voyles AS, et al. Accurate determination of production data of the non-standard positron emitter 86Y via the 86Sr(p,n)-reaction. Radiochim Acta (2020) 108:747–56.
31. DeGraffenreid AJ, Medvedev DG, Phelps TE, Gott MD, Smith SV, Jurisson SS, et al. Cross-section measurements and production of 72Se with medium to high energy protons using arsenic containing targets. Radiochim Acta (2019) 107:279–87. doi:10.1515/ract-2018-2931
32. Pupillo G, Mou L, Martini P, Pasquali M, Boschi A, Cicoria G, et al. Production of 67Cu by enriched 70Zn targets: first measurements of formation cross sections of 67Cu, 64Cu, 67Ga, 66Ga, 69mZn and 65Zn in interactions of 70Zn with protons above 45 MeV. Radiochim Acta (2020) 108:593–602. doi:10.1515/ract-2019-3199
33. Pupillo G, Sounalet T, Michel N, Mou L, Esposito J, Haddad F New production cross sections for the theranostic radionuclide 67Cu. Nucl Instr Methods Phys Res Section B: Beam Interactions Mater Atoms (2018) 415:41–7. doi:10.1016/j.nimb.2017.10.022
34. Pupillo G, Mou L, Boschi A, Calzaferri S, Canton L, Cisternino S, et al. Production of 47Sc with natural vanadium targets: results of the PASTA project. J Radioanal Nucl Chem (2019) 322:1711–8. doi:10.1007/s10967-019-06844-8
35. Ermolaev SV, Zhuikov BL, Kokhanyuk VM, Matushko VL, Srivastava SC Cross sections and production yields of 117mSn and other radionuclides generated in natural and enriched antimony with protons up to 145 MeV. Radiochim Acta (2020) 108:327–51. doi:10.1515/ract-2019-3158
36. Qaim SM, Spahn I, Scholten B, Neumaier B Uses of alpha particles, especially in nuclear reaction studies and medical radionuclide production. Radiochim Acta (2016) 104:601–24. doi:10.1515/ract-2015-2566
37. Verhoeven H, Cocolios TE, Dockx K, Farooq-Smith GJ, Felden O, Formento-Cavaier R, et al. Measurement of spallation cross sections for the production of terbium radioisotopes for medical applications from tantalum targets. Nucl Instr Methods Phys Res Section B: Beam Interactions Mater Atoms (2020) 463:327–9. doi:10.1016/j.nimb.2019.04.071
38. Steyn GF, Vermeulen C, Szelecsényi F, Kovács Z, Hohn A, van der Meulen NP, et al. Cross sections of proton-induced reactions on 152Gd, 155Gd and 159Tb with emphasis on the production of selected Tb radionuclides. Nucl Instr Methods Phys Res Section B: Beam Interactions Mater Atoms (2014) 319:128–40. doi:10.1016/j.nimb.2013.11.013
39. Starovoitova VN, ColeCole PLPL, Grimm TL Accelerator-based photoproduction of promising beta-emitters 67Cu and 47Sc. J Radioanal Nucl Chem (2015) 305:127–32. doi:10.1007/s10967-015-4039-z
40. Rotsch DA, Brown MA, Nolen JA, Brossard T, Henning WF, Chemerisov SD, et al. Electron linear accelerator production and purification of scandium-47 from titanium dioxide targets. Appl Radiat Isot (2018) 131:77–82. doi:10.1016/j.apradiso.2017.11.007
41. Aliev RA, Belyshev SS, Kuznetsov AA, Dzhilavyan LZ, Khankin VV, Aleshin GY, et al. Photonuclear production and radiochemical separation of medically relevant radionuclides: 67Cu. J Radioanal Nucl Chem (2019) 321:125–32. doi:10.1007/s10967-019-06576-9
42. Inagaki M, Sekimoto S, Tanaka W, Tadokoro T, Ueno Y, Kani Y, et al. Production of 47Sc, 67Cu, 68Ga, 105Rh, 177Lu, and 188Re using electron linear accelerator. J Radioanal Nucl Chem (2019) 322:1703–9. doi:10.1007/s10967-019-06904-z
43. Sugo Y, Hashimoto K, Kawabata M, Saeki H, Sato S, Tsukada K, et al. Application of 67Cu produced by 68Zn(n,n′p+d)67Cu to biodistribution study in tumor-bearing mice. J Phys Soc Jpn (2017) 86:023201. doi:10.7566/jpsj.86.023201
Keywords: accelerator-produced radionuclides, decay properties, reaction cross sections, positron emitters, therapeutic radionuclides, theranostic approach, radionuclide targeted therapy
Citation: Qaim SM, Hussain M, Spahn I and Neumaier B (2021) Continuing Nuclear Data Research for Production of Accelerator-Based Novel Radionuclides for Medical Use: A Mini-Review. Front. Phys. 9:639290. doi: 10.3389/fphy.2021.639290
Received: 08 December 2020; Accepted: 08 January 2021;
Published: 27 April 2021.
Edited by:
Zhen Cheng, Stanford University, United StatesReviewed by:
Ferid Haddad, Université de Nantes, FranceGaia Pupillo, Legnaro National Laboratories (INFN), Italy
Copyright © 2021 Qaim, Hussain, Spahn and Neumaier. This is an open-access article distributed under the terms of the Creative Commons Attribution License (CC BY). The use, distribution or reproduction in other forums is permitted, provided the original author(s) and the copyright owner(s) are credited and that the original publication in this journal is cited, in accordance with accepted academic practice. No use, distribution or reproduction is permitted which does not comply with these terms.
*Correspondence: Syed M. Qaim, cy5tLnFhaW1AZnotanVlbGljaC5kZQ==
†These authors have contributed equally to this work