- 1Jülich Centre for Neutron Science (JCNS) at Heinz Maier-Leibnitz Zentrum (MLZ), Forschungszentrum Jülich, Garching, Germany
- 2Heinz Maier-Leibnitz Zentrum (MLZ), Technische Universität München, Garching, Germany
- 3Jülich Centre for Neutron Science (JCNS) at Spallation Neutron Source (SNS), ORNL, Oak Ridge, TN, United States
- 4Jülich Centre for Neutron Science (JCNS-1), Forschungszentrum Jülich, Jülich, Germany
- 5Lehrstuhl für Funktionelle Materialien, Physik-Department, Technische Universität München, Garching, Germany
We present a structural and dynamical analysis of the influence of NaCl on multilayer stacks of phospholipids on a solid surface. To this end, multilayer stacks of phospholipids (L-α-phosphatidylcholine, abbreviated as SoyPC) are investigated with neutron reflectometry, grazing-incidence small-angle neutron scattering (GISANS) and grazing-incidence neutron-spin echo spectroscopy (GINSES). We show both that the NaCl influence on the structure is predominantly on water-head group interface and also, that the change in dynamics is restricted to an associated change in the inter-plane viscosity. Using this knowledge, it is possible to model the dynamical behavior of a phospholipid membrane in response to a salt concentration of the solvent using only a single parameter, namely the in-plane viscosity. The excellent agreement with our previously published model also strongly supports the existence of a thermally excited surface mode in phospholipid membranes for close-to-physiological conditions.
Introduction
Phospholipids are a major constituent of the plasma membranes in the vast majority of biological organisms. These membranes play a crucial role in a wide variety of important mechanisms and properties from drug delivery [1] to permeability in cellular membranes [2]. In the case of the latter, the interplay between dynamics and structure is of particular importance. The structure and dynamics of phospholipid membranes has been the subjects of a wide array of investigations ranging from light-scattering [2] and AFM studies [3] over X-ray scattering [4–10] to elastic [11–13]and inelastic neutron scattering [14–17]. An overview of the interplay between structure and dynamical behavior was published by Tanaka [18], and reviews focusing on neutron and X-ray scattering were published by Rheinstädter and Fragneto [19], and Salditt [8]. Moreover, an overview focused on grazing incidence methods on similar systems was given before [20].
In this study, we use elastic neutron scattering methods, reflectometry and grazing-incidence small-angle neutron scattering (GISANS), to evaluate the structure of a phospholipid membrane stack when NaCl is introduced to the solvent. NaCl addition introduces ions to the system and can be understood as an approach to getting closer to physiological conditions. It can be used to separate possible effects observed in unadulterated (i.e. in the absence of salt) in vitro experiments and examine their applicability to real-life systems. A previous investigation on the effects of various salts on the structure of lipid membranes found that the inter-lamellar spacing increases on addition of NaCl [21] However, in this work, we find that the overall dimensions of the membranes remain stable and there is little change in the bilayer thickness due to the salt concentration. We attribute this difference to a different approach of data evaluation. Hishida et al. used one single main peak position to determine the bilayer thickness. Using inverse Fourier transform we are able to show that an additional peak (Q = 0.05 Å−1), which appears for higher NaCl concentrations at lower Q-values, is actually connected to the changes at the phospholipid-water interface of the bilayer. The same is true for the peak splitting (Q = 0.09 Å−1). This more complicated reflectometry data probably cannot be adequately analyzed by using only a single peak position.
In an earlier work, focusing on the grazing-incidence small-angle neutron-spin echo spectroscopy (GINSES) technique [17], we demonstrated the existence of a hitherto unknown surface mode in a phospholipid membrane stack system which did not include any salt. Here we extend this investigation to systems with an NaCl-containing solvent, and examine the impact of the NaCl-ions on the membrane. This surface mode we postulate in our previous work is a long-range in-plane correlation, which is on the order of a few hundred Angstroms (Q∼0.01 A−1) with an energy transfer in the µeV range [16, 17, 22]. One of the questions that we try to answer in this study is, whether this phenomenon is only restricted to very pure phospholipid systems, or whether this is a general phenomenon in phospholipid membranes, also at closer to physiological conditions as described here.
The measurements presented here show that the effects of NaCl concerning structure is mostly concentrated on the interface between the phospholipid headgroups and the water layer, while not fundamentally impacting on the membrane stack nature of the samples.
Concerning the dynamical behavior such systems we show that the description put forward in our previous manuscript for the pure multilayer stack system also holds in case of NaCl introduction to the system. Only the intramembrane viscosity changes. This finding proves the existence of the previously shown surface mode being present in the phospholipid systems. Furthermore, it increases the predictive power of our model to systems, which are very close to those found under physiological conditions.
Materials and Methods
Materials
The materials used in the experiments reported here are L-α-phosphatidycholine, 95% (SoyPC) procured from Avanti Lipids, NaCl obtained from SigmaAldrich and D2O (99.8 atom-% D) from Deutero.
The sample preparation was identical in all cases, irrespective of the experiments being performed. A solution of 5 g SoyPC in 34 g of isopropanol (pA) was prepared. This was done at room-temperature (RT) and the mixture was shaken for at least 20 min. This always resulted in a clear solution with a slight yellow tinge.
In parallel, a silicon (Si) block with a neutron-resonator was prepared by cleaning it for at least 2 h in a 2% Hellanex solution. The resonating structure improved the scattering characteristics in for the GINSES measurements, without impeding those in the case of neutron reflectometry or GISANS [23]. The resonator is a layered structure of sputtered platinum and titanium, which provides a constructive interference for neutrons within an acceptable windows in terms of wavelength and incoming angle. This increases the probability of interaction of the neutrons with the sample. For the complete procedure, we followed the well-established protocol of our previous publications [13, 24].
In short, 12 ml SoyPC solution was solution cast onto the silicon block and dried overnight at a pressure of 250 mbar at room temperature to allow for ideal self-assembly conditions.
For the measurements, the prepared Si-blocks were fixed into a sample holder that provided a confined, water-tight space above the sample film on the surface. Finally, D2O containing the appropriate salt concentrations (as indicated in Table 1) were injected into the cell.
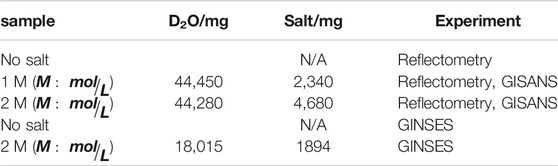
TABLE 1. Summary of samples used and their respective NaCl concentrations. If no salt was added to the sample, it was measured in pure D2O.
Sample Block and Geometry
A sketch of the sample block and the resulting geometry are given in Figure 1. It is important to note that the neutrons always impinge onto the phospholipid sample from the silicon side, at the solid/liquid interface. This provides an excellent orientation of the sample and a very smooth surface for the self-assembly of the phospholipid layer stack.
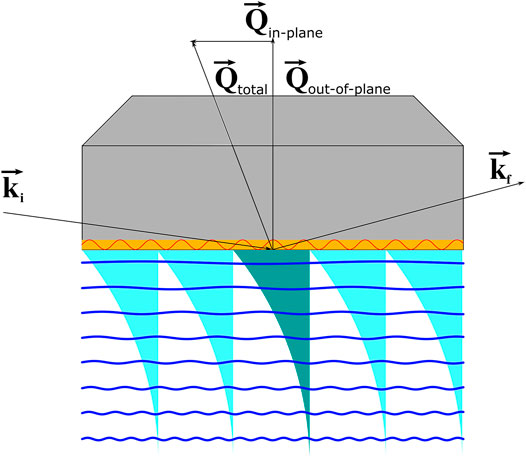
FIGURE 1. Sketch of the applied scattering geometry: For reflectometry the incoming wavevector ki and outgoing wavevector kf are identical. Hence, the total scattering vector Q is an out-of-plane vector perpendicular to the sample surface. For GISANS, a range of scattering angles are collected on the detector and therefore there are both in and out-of-plane components of the Q-vector, depending on the position on the detector. In case of the GINSES measurement, ki and kf are strictly different (incoming angle 0.2°, outgoing angle 8°) which leads to an in-plane component of Qin-plane = 0.0072 Å−1. However, since the active detector area of 10 cm width at 4 m sample detector distance is involved into data collection the uncertainty of outgoing angle is 0.25 mrad, which results in the uncertainty described in the text.
In terms of orientation a distinction must be made between the set-up for the GISANS and reflectometry experiments versus that for the GINSES experiment. The GISANS and reflectometry experiments were carried out on a single wavelength instrument, using either a reflectometry geometry, where the Q-vector is perfectly perpendicular to the sample surface or a GISANS geometry where the incoming angle is fixed and therefore a set of Q-values is probed, including lateral information. GINSES on the other hand was performed on a time-of-flight instrument with a wavelength spread of the incoming neutrons. Since the in-plane Q-vector is defined by the difference between the incoming and the outgoing angle of the neutrons, and the outgoing angle and Q-values are a function of each other, this in-plane Q-vector carries a systematical error of 2.5 ∙ 10−4 Å−1 due to the pixel size of the detector (3.75 cm pixels at 4 m sample detector distance). The impact of this uncertainty can be seen by the shaded areas in the GINSES data in Figure 2.
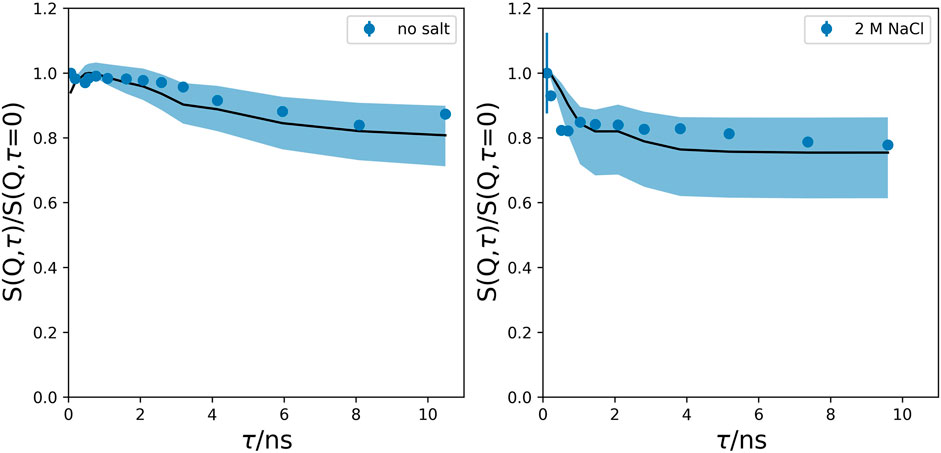
FIGURE 2. GINSES data for sample without (left panel) salt and a sample with a 2 M NaCl concentration in the solvent (right panel). The black lines represent the data either calculated from fits in our previous publication [17]. The shaded areas represent the confidence areas using the uncertainties for the in-plane Q. The extremely good correspondence, even without a fit (see text) corroborates our earlier and current use of the Romanov layer model and the existence of the surface mode shown in our previous publications. The very fast decay for short times in the case of a 2 M NaCl concentration we attribute to a facilitated sliding of the membrane layers against each other for short times.
GISANS and Reflectometry
GISANS and neutron reflectometry measurements were performed on the MARIA [25] reflectometer of JCNS at MLZ, FRM2, Garching, Germany. The temperature was controlled by a Julabo water thermostat. Temperature calibration was done by calibrating the Julabo temperature to a direct measurement of the temperature of the sample block. Incident angles for GISANS were always αi = 0.2°, with a neutron wavelength of λ = 5 Å.
GISANS and conversely grazing-incidence x-ray scattering (GISAXS) have been established since long as valuable tools for the investigation of surfaces and interfaces [20]. Due to the shallow incident angle (below or close to the critical angle of total external reflection) there is a large footprint of the beam, which allows that a large area average is made. In comparison to reflectometry, where the information may be extracted for similar average surface areas, GISANS and GISAXS analyze also non-specular intensities, which probe in-plane correlations, such as patches along the surface, to be investigated.
GINSES
GINSES measurements were performed at on the SNS-NSE [26] neutron spin-echo spectrometer of JCNS at SNS, ORNL, Oak Ridge (TN), USA. Temperature was controlled by a Julabo with a temperature sensor directly connected to the Si-sample block. Also here, the incident angle was set to αi = 0.2° as well. The exit angle was 8°, which leads to an in-plane scattering vector of
There are two aspects for using a grazing incidence geometry for neutron spin-echo spectroscopy (NSE): 1) Since incoming and outgoing angle are not identical, very small in-plane Q-values are accessible (see Figure 1), which are usually hidden to NSE, due to the rather large primary beam, and the detectors usually not being able to work under full beam conditions. The other reason 2) is to exploit the same advantages in surface scattering as GISANS and GISAXS, namely averaging over a large surface area and thereby enabling the investigation of films and interfaces. The authors gave an extensive overview of the technique in a previous publication [23].
Inverse FFT
The inverse FFT analysis used in the present data analysis is based on a self-written code by the authors using the approaches presented by Stroud and Li [27, 28]. While the resulting fits reproduce the data extremely well, due to boundary condition effects this method obtains most of the resulting neutron scattering length density (SLD) data directly from the area around the peaks. Accordingly, although the shape of the individual multilayer is well reproduced, no information about the layer-to-layer distance is included. This information is then obtained by the classical use of the peak position.
Results
The results are split in sections for GISANS/reflectometry and GINSES to make a distinction between structural and dynamic behavior, which is investigated with the different measurements. As an overall observation it can be stated that the stacked, lamellar nature of the phospholipid membranes, as was reported also in earlier investigations, is rather stable. The investigated salt concentrations of 1 and 2 M NaCl do not drive the system into a non-lamellar phase.
Reflectometry
Representative neutron reflectometry data is shown in Figure 3 and Figure 4. The most apparent feature is that for the 1 M salt concentration there is little distortion of the primary peak (Q ≈ 0.09 to 0.1 Å-1) of the lamellar order, while in case of the 2 M concentration, this peak is split into two peaks (Q ≈ 0.09 Å-1 and Q ≈ 0.12 Å-1). Moreover, an additional peak appears closer to the primary beam position in case of 2 M (Q ≈ 0.05 Å-1).
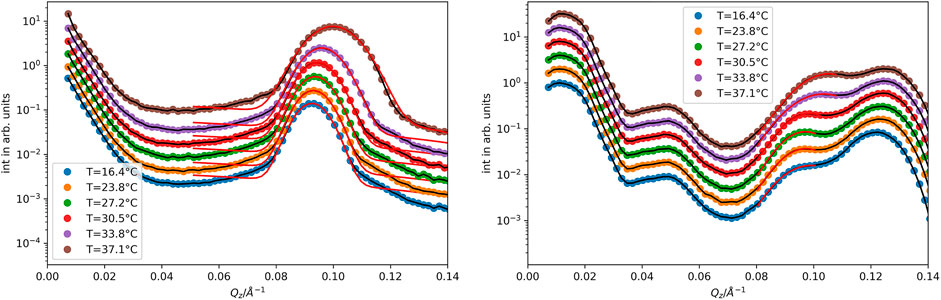
FIGURE 3. Reflectometry data of SoyPC samples with 1 M (left panel) and 2 M (right panel) NaCl in the D2O solvent. The black lines represent fits obtained by inverse FFT analysis, while the red lines represent Gaussian fits to determine the correct peak positions. The curves are shifted along the intensity axis for clarity of the representation.
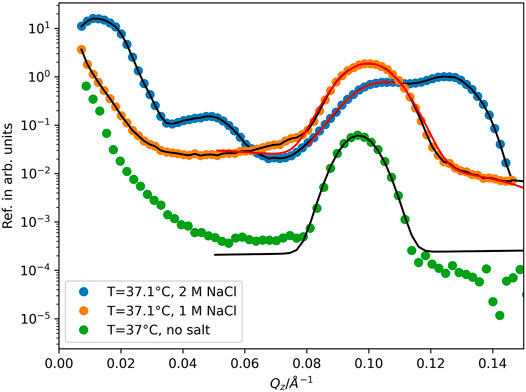
FIGURE 4. Reflectometry data for different NaCl concentrations at physiological temperatures. The curves are shifted along the intensity axis for clarity of the presentation.
In order to analyze the double layer thickness, we analyzed the peak positions of for the 1 M salt concentration and the peak position of the corresponding forward peak for the 2 M concentration. Both the peak positions and the resulting double layer thicknesses d (
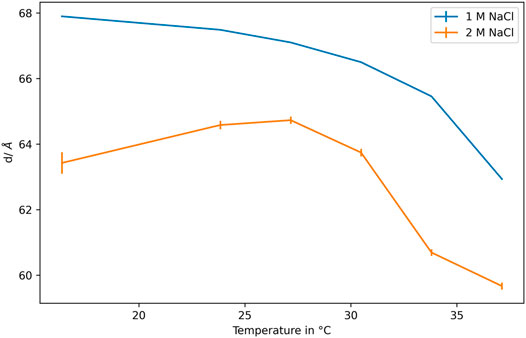
FIGURE 5. Double layer distances d from reflectometry data over temperature. The error bars are vanishingly small for the 1 M NaCl concentration.
The more complex structure at the 2 M salt concentration necessitated an inverse Fourier transform algorithm for analysis of the reflectivity data. Previous attempts to use the Parrat algorithm for the data analysis only worked for the condition 1 M, but were unsuccessful in finding a solution that was both algorithmically stable, in combination with the physical assumptions about the SLDs and thicknesses in the model, as well as describing the data fairly well. The resulting SLD profiles are shown in Figure 6. From those data it is apparent the thickness of the single lipid layer is constant with respect to temperature. The most pronounced difference is visible for the water/lipid interface, where the headgroup of the phospholipid is located. There a pronounced step is visible for the 2 M NaCl sample at low temperatures, while there is an additional undulation in case of high temperatures. The 1 M NaCl sample and the sample without salt do not exhibit this additional step, but have a single step between the SLD of the phospholipid and water region respectively.
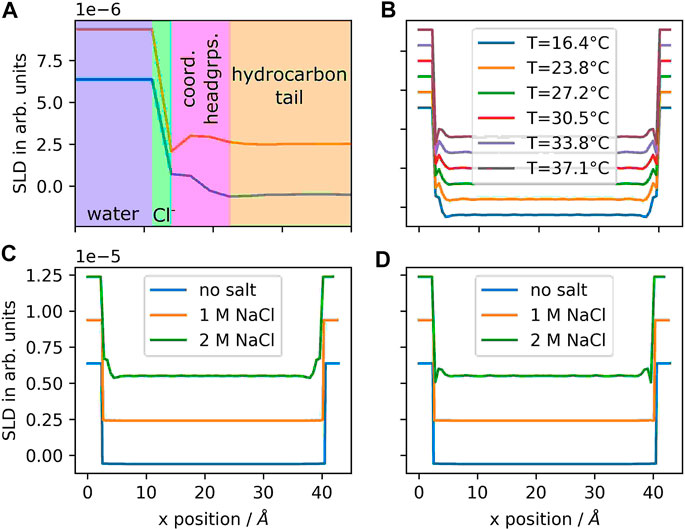
FIGURE 6. SLD profiles determined from reflectometry data via inverse FFT analysis; (A): 2 M, lowest and highest temperature zoom-in. The water area (D2O SLD = 6.3 10−6 Å−2) is shaded blue, the area where chlorine ions are enriched is shaded green, the area where sodium ions interact with the phospholipid head groups, causing a temperature dependent coordination is shaded magenta and the area of the hydrocarbon tail (C10H22 SLD = −0.49 ∙10−6 Å−2); (B): 2 M all temperatures, (C): all concentrations 1 and 2 M at 16.4°C, salt-free at 37°C; (D): all concentrations 1 and 2 M at 37.1°C, salt-free at 37°C. Upper limit in all cases is 6.3 10−6 Å−2 for D2O and −0.49 10−6 Å−2 for the pure hydrocarbon tails of the phospholipids. Curves are shifted against each other for better visibility.
GISANS
In addition to the reflectometry measurements probing structure information along the surface normal, GISANS measurements allow to probe in-plane features [29]. Such in-plane features could hint at an additional order or a phase transition in the in-plane direction. Representative GISANS data are shown in Figure 7.
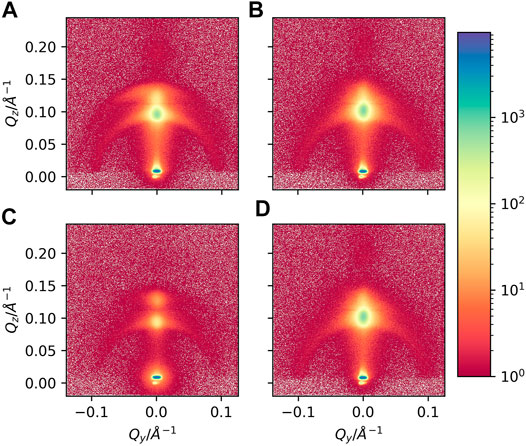
FIGURE 7. Representative GISANS images of samples with 1 M NaCl (A: 16.4°C and B: 37.1°C) and 2 M NaCl (C: 16.4°C and D: 37.1°C).
In the GISANS data, the only off-specular features are Debye-Scherrer rings. This observation clearly indicates, that apart from a slight disordering of the membrane stack in-plane, there is no structure formed upon the addition of NaCl salt. The additional secondary peak slightly above the primary lamellar peak is more pronounced at low temperatures. For 1 M salt concentration it is hard to be distinguished and only visible at low temperatures, while it is more pronounced and visible at all temperatures for the 2 M salt concentration. In addition, the secondary lamellar peak is visible. This secondary peak is extremely weak, but still an excellent guide to find the correct lamellar distance. For such a weak peak, splitting is impossible to see, so we use it only to identify the primary peak position, which otherwise is challenged by the additional splitting. This approach also helps to identify the correct peak position for the reflectivity data, which is apparently the one at lower Q-values. The distances extracted from vertical line cuts of the 2D GISANS data (Figure 8) confirm the distances obtained from the reflectometry data.
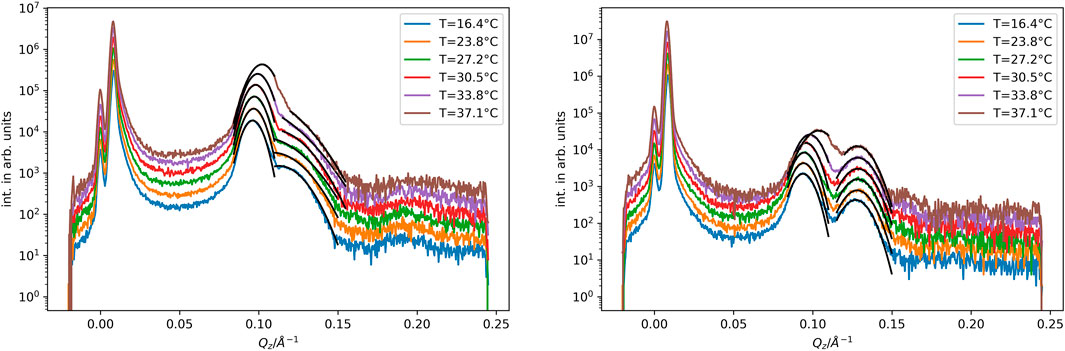
FIGURE 8. Vertical line cuts of the 2D GISANS data, taken at Qy = 0 for 1 M (left panel) and 2 M (right panel) NaCl concentration. Black lines are Gaussian fits to extract peak positions. Curves are shifted along the intensity axis for clarity of the presentation.
One difference between the reflectometry and vertical line cut data is the relative intensity of the secondary peak, which we attribute to the peak-splitting caused by SLD-variations close to the head-group of the phospholipids. Due to the different collimation conditions for reflectometry and GISANS the interplay between those two peaks is considerably more complex in the GISANS case, where an overlap of the two peaks occurs due to no collimation slits after the sample. This leads to a slight apparent shift of those peaks, as well as to a difference in relative intensity when compared with the peak at lower Q-values.
GINSES
In the GINSES measurements, we compare measurements of SoyPC membrane stacks without NaCl in the D2O solvent, and those with a 2 M NaCl concentration.
The respective data is shown in Figure 2. Both experiments were performed at physiological temperatures (37°C) and, apart from the presence of salt in the solvent, identical conditions with a scattering vector of Q = 0.106 Å-1 and an in-plane scattering vector of Qin-plane = 0.0072 Å-1. It is immediately apparent that the most prominent feature is the fast decay below τ = 1 ns for the sample with the 2 M salt concentration. We are able to fit the data with exactly the same parameters as in our previous publications for both samples [16, 17]. This evaluation was based on a theory by Romanov et at [30] and treated the membrane stack as a number of elastic layers close to a solid substrate, where wave modes govern the dynamic behavior of the membrane.
Romanov et al. solved the coupled equations of motion for infinitely thin membranes adjacent to a solid substrate, based on the assumption of a smectic liquid crystal, where thermal fluctuations (displacement forces), restoring forces and coupling between the membranes were considered. The coupling between the membranes was effected by elastic and viscous forces, which then related to the bulk viscosity and compressibility. NB, the bulk viscosity is different from the layer sliding viscosity η3, which was the layer sliding viscosity of the membranes along each other.
The only parameter that changed between the two fits is the in-plane viscosity η3. All other parameters were directly taken from our previous publication and are shown in Table 2. These parameters were not fitted, however if left free reverted to similar values. Thus, the difference in dynamic behavior can be attributed solely to a change in the viscosity of the system along the membrane surface. The value of η3 = 0.0023 Pa s is approximately a third of the value for the salt-free system.
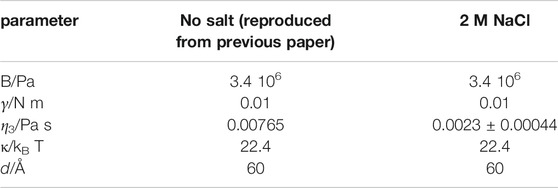
TABLE 2. Parameters used to calculate curves for the GINSES description. Only viscosity is fitted. Within the errorbars, the remaining parameters returned to the given values taken from the previous publication.
Discussion
We investigated the influence of NaCl salt concentration on the structure and dynamical behavior of phospholipid membrane systems (SoyPC) using neutron reflectometry, GISANS and GINSES.
Concerning the structure, the major impact of introducing NaCl into the system happens around the headgroup of the phospholipids. While for 1 M salt concentration the splitting of the primary lamellar peak is still difficult to be distinguished in the reflectometry data, in GISANS the splitting is more prominent, due to the different collimation conditions. We attribute this splitting to the onset of enrichment of ion-counter ion pairs along the head groups of the phospholipid.
Increasing the NaCl concentration to 2 M leads to a very prominent peak splitting and we find an enrichment of ion-counter ion pairs at the interface between the phospholipid and the D2O. This finding is corroborated by the temperature behavior of the SLD-undulation at the interface. The much smaller ions are a lot more mobile at higher temperatures, leading to a more distinctive ion-counter ion pair at higher temperatures causing a sharper contrast than at lower temperatures. A similar behavior has been observed in MD-simulations, where the sodium ions were mostly located at the head group of the phospholipid and the chlorine ions were mostly located in water directly adjacent to the phospholipid layer and did not change the overall structure of the membrane [31, 32]. The increase of the layer thickness upon addition of NaCl to PC multilayers, as reported in another study, cannot be confirmed by our data [21]. We assume that the change in thickness was attributed to the choice made which of the two split peaks was chosen for a simple single peak to double layer thickness correlation in the previous work. In the present work, however, we show that this splitting can be attributed to the difference in structure of the interface, due to the NaCl ion-counter ion pairs at the head groups of the phospholipids, and that it is not necessary to include an additional assumption about double layer distance to interpret the data, which is continuously evolving with temperature in a manner consistent with literature [33–35]. The change in thickness as can be expected from the phase changes (essentially a phase transition of the tail groups from liquid crystalline to liquid) is as pronounced (approx. 4 Å in each instance) as is the effect of the NaCl concentration.
We attribute the more pronounced difference between low and high temperature concerning the structure close to the head group-water interface, which is nearly negligible for 1 M NaCl concentration and only visible for the 2 M NaCl concentration to the more pronounced coordination as reported by Böckmann et al. [31]. Not only does this make a the more pronounced wriggling (instead of the nearly box-like profile for 1 M NaCl) at 2 M NaCl better understandable, but also the temperature sensitivity, since the coordination as such is a transient state and therefore more sensitive to thermal excitation.
The GINSES results corroborate the findings from our previous publication, in that without additional fitting the case of the salt-free sample can be reproduced within the experimental uncertainty. In addition, by only adjusting the in-plane viscosity η3 to a lower value, the measurements of a sample with a 2 M NaCl concentration are reproduced very well. This compares to bulk values from literature, where the viscosity of pure water also changes upon the addition of NaCl from 1.002 Pa s to 1.250 Pa s for a 2 M salt concentration [36]. Thus, it can be seen, that even though the viscosity for bulk water increases, the interface effect seems to dominate, such that the sliding of the membranes against each other is facilitated by the ion-ion interaction and the in-plane viscosity decreases. In addition, a higher coordination as reported by Böckmann et al. [31] for higher NaCl concentrations improves the in-plane ordering, which might facilitate the sliding of two membranes against one another. Since the bulk viscosity of water is changing a lot weaker than the in-plane viscosity, and in the opposite direction, we assume this a structural effect due to the influence of the ordering of the membranes.
This allows also to understand the interplay between the dynamics and the structure of the sample. Since the NaCl ion-counter ion pairs are located at the water-phospholipid interface they can also be expected to have a profound impact of the viscosity of these layers against each other. Essentially, they decrease the resistance of the water layer against sliding along the phospholipid layer.
Due to experimental limitations (each GINSES curve takes 3–4 days without setup) we are not able to test this behavior as a function of temperature, but from the present data it seems reasonable to assume that the location and interpenetration of the phospholipid headgroups and the ion-counter ion pairs change their mobility in the surface as they also change their spatial arrangement.
Summarizing, this study shows both the influence on the structure of phospholipid membranes upon the addition of NaCl salt. The principal effect of salt addition is the incorporation of ions close to the headgroup-water interface, which is to be expected, since there they find the highest charge density in the overall system. This is in line with the very short ranged dipolar field of the zwitterionic head group that is barely shielded by NaCl ions on long ranges but has short range compensations [37] compared to isolated ions in the Debye-Hückel [38] theory (κ−1 = 2.2 Å). So the only effect of the NaCl ions is on the viscosity of the water and only weakly changes the dipole field effect on the bending rigidity in contrast to simply charged head groups [39, 40]. This can be understood as the small-scale analogue of rough surfaces sliding against each other. As soon as the roughness of the surface is overcome by a lubricant, even if this effect is small compared to the overall thickness, there is a profound impact on the overall system.
This behavior has also been observed in MD simulations [31]. Such a change in the structure, and the location of the ions, then lead to a distinctly different dynamic behavior. The previously postulated dynamic surface mode is still present and can be reproduced in our newer measurements. Moreover, by changing only the in-plane viscosity and keeping all other parameters constant, the behavior of the membrane can be correctly described. This finding strongly supports both the existence of the surface mode and their description and treatment using the Romanov theory.
It is interesting to speculate, what impact this surface mode has on the in vivo behavior of biological membranes. Since this surface mode seems to be universally present and the Romanov model also develops predictive powers. The extent to which such modes play a role in the functioning of living organisms is intriguing and warrants and requires substantial further research.
Data Availability Statement
The original contributions presented in the study are included in the article, further inquiries can be directed to the corresponding author.
Author Contributions
SJ planned and conducted the experiments, performed data analysis and was primary author of the manuscript. OH, HF, AK, and PZ planned and conducted the experiments, participated in data analysis and the writing of the manuscript. DH, SF and PM-B participated in data analysis and the writing of the manuscript.
Funding
This research was carried out on internal funding of the Jülich Center for Neutron Science institute. PM-B acknowledges funding by German Research Foundation (MU 1487/).
Conflict of Interest
The authors declare that the research was conducted in the absence of any commercial or financial relationships that could be construed as a potential conflict of interest.
Acknowledgments
The authors acknowledge being awarded beamtime by the JCNS on the MARIA and SNS-NSE instruments.
References
1. Cevc G. Drug Delivery across the Skin. Expert Opin Investig Drugs (1997) 6(12):1887–937. doi:10.1517/13543784.6.12.1887
2. Touitou E, Dayan N, Bergelson L, Godin B, Eliaz M. Ethosomes - Novel Vesicular Carriers for Enhanced Delivery: Characterization and Skin Penetration Properties. J Controlled Release (2000) 65(3):403–18. doi:10.1016/s0168-3659(99)00222-9
3. Butt H-J, Cappella B, Kappl M. Force Measurements with the Atomic Force Microscope: Technique, Interpretation and Applications. Surf Sci Rep (2005) 59(1–6):1–152. doi:10.1016/j.surfrep.2005.08.003
4. Constantin D, Brotons G, Salditt T, Freyssingeas E, Madsen A. Dynamics of Bulk Fluctuations in a Lamellar Phase Studied by Coherent X-Ray Scattering. Phys Rev E (2006) 74(3):31706. doi:10.1103/physreve.74.031706
5. Pabst G, Katsaras J, Raghunathan VA, Rappolt M. Structure and Interactions in the Anomalous Swelling Regime of Phospholipid Bilayers†. Langmuir (2003) 19(5):1716–22. doi:10.1021/la026052e
6. Safinya CR, Roux D, Smith GS, Sinha SK, Dimon P, Clark NA, et al. Steric Interactions in a Model Multimembrane System: a Synchrotron X-Ray Study. Phys Rev Lett (1986) 57(21):2718–21. doi:10.1103/physrevlett.57.2718
7. Safinya CR, Sirota EB, Roux D, Smith GS. Universality in Interacting Membranes: the Effect of Cosurfactants on the Interfacial Rigidity. Phys Rev Lett (1989) 62(10):1134–7. doi:10.1103/physrevlett.62.1134
8. Salditt T. Thermal Fluctuations and Stability of Solid-Supported Lipid Membranes. J Phys Condens Matter (2005) 17(6):R287–R314. doi:10.1088/0953-8984/17/6/r02
9. Malaquin L, Charitat T, Daillant J. Supported Bilayers: Combined Specular and Diffuse X-Ray Scattering. Eur Phys J E (2010) 31(3):285–301. doi:10.1140/epje/i2010-10578-2
10. Reusch T, Mai D, Osterhoff M, Khakhulin D, Wulff M, Salditt T. Nonequilibrium Collective Dynamics in Photoexcited Lipid Multilayers by Time Resolved Diffuse X-Ray Scattering. Phys Rev Lett (2013) 111(26):268101. doi:10.1103/physrevlett.111.268101
11. Schneck E, Rehfeldt F, Oliveira RG, Gege C, Demé B, Tanaka M. Modulation of Intermembrane Interaction and Bending Rigidity of Biomembrane Models via Carbohydrates Investigated by Specular and Off-Specular Neutron Scattering. Phys Rev E (2008) 78(6):61924. doi:10.1103/physreve.78.061924
12. Jaksch S, Koutsioubas A, Mattauch S, Holderer O, Frielinghaus H. Measurements of Dynamic Contributions to Coherent Neutron Scattering. Colloids Inter (2018) 2(3):31. doi:10.3390/colloids2030031
13. Jaksch S, Lipfert F, Koutsioubas A, Mattauch S, Holderer O, Ivanova O, et al. Influence of Ibuprofen on Phospholipid Membranes. Phys Rev E (2015) 91(2):9. doi:10.1103/physreve.91.022716
14. Armstrong CL, Toppozini L, Dies H, Faraone A, Nagao M, Rheinstädter MC. Incoherent Neutron Spin-Echo Spectroscopy as an Option to Study Long-Range Lipid Diffusion. ISRN Biophys (2013) 2013:439758. doi:10.1155/2013/439758
15. Armstrong CL, Häußler W, Seydel T, Katsaras J, Rheinstädter MC. Nanosecond Lipid Dynamics in Membranes Containing Cholesterol. Soft matter (2014) 10(15):2600–11. doi:10.1039/c3sm51757h
16. Holderer O, Jaksch S, Zolnierczuk P, Ohl M, Frielinghaus H. Phospholipid Membrane Dynamics at the Solid–Liquid Interface Studied with Grazing Incidence Neutron Spin Echo Spectroscopy. J Phys Conf Ser (2019) 1316:012001. doi:10.1088/1742-6596/1316/1/012001
17. Jaksch S, Holderer O, Gvaramia M, Ohl M, Monkenbusch M, Frielinghaus H. Nanoscale Rheology at Solid-Complex Fluid Interfaces. Scientific Rep (2017) 7(1):1–7. doi:10.1038/s41598-017-04294-4
18. Tanaka M. Physics of Interactions at Biological and Biomaterial Interfaces. Curr Opin Colloid Interf Sci (2013) 18(5):432–9. doi:10.1016/j.cocis.2013.07.002
19. Fragneto G, Rheinstädter M. Structural and Dynamical Studies from Bio-Mimetic Systems: an Overview. Comptes Rendus Physique (2007) 8(7–8):865–83. doi:10.1016/j.crhy.2007.09.003
20. Jaksch S, Gutberlet T, Müller-Buschbaum P. Grazing-incidence Scattering-Status and Perspectives in Soft Matter and Biophysics. Curr Opin Colloid Interf Sci (2019) 42:73–86. doi:10.1016/j.cocis.2019.04.001
21. Hishida M, Yamamura Y, Saito K. Salt Effects on Lamellar Repeat Distance Depending on Head Groups of Neutrally Charged Lipids. Langmuir (2014) 30(35):10583–9. doi:10.1021/la502576x
22. Jaksch S, Koutsioubas A, Mattauch S, Holderer O, Frielinghaus H. Long-range Excitations in Phospholipid Membranes. Chem Phys Lipids (2019) 225:104788. doi:10.1016/j.chemphyslip.2019.104788
23. Frielinghaus H, Gvaramia M, Mangiapia G, Jaksch S, Ganeva M, Koutsioubas A, et al. New Tools for Grazing Incidence Neutron Scattering Experiments Open Perspectives to Study Nano-Scale Tribology Mechanisms. Nucl. Instrum. Methods Phys. Res. A (2017) 871:72–6. doi:10.1016/j.nima.2017.07.064
24. Jaksch S, Holderer O, Gvaramia M, Ohl M, Monkenbusch M, Frielinghaus H. Nanoscale Rheology at Solid-Complex Fluid Interfaces. Scientific Rep (2017) 7(1):4417. doi:10.1038/s41598-017-04294-4
25. Mattauch S, Koutsioubas A, Rücker U, Korolkov D, Fracassi V, Daemen J, et al. The High-Intensity Reflectometer of the Jülich Centre for Neutron Science: MARIA. J Appl Cryst (2018) 51(3):646–54. doi:10.1107/s1600576718006994
26. Ohl M, Monkenbusch M, Arend N, Kozielewski T, Vehres G, Tiemann C, et al. The Spin-Echo Spectrometer at the Spallation Neutron Source (SNS). Nucl Instr Methods Phys Res Section A: Acc Spectrometers, Detectors Associated Equipment (2012) 696:85–99. doi:10.1016/j.nima.2012.08.059
27. Stroud RM, Agard DA. Structure Determination of Asymmetric Membrane Profiles Using an Iterative Fourier Method. Biophysical J (1979) 25(3):495–512. doi:10.1016/s0006-3495(79)85319-9
28. Li M, Möller MO, Landwehr G. Fitting of X‐ray or Neutron Specular Reflectivity of Multilayers by Fourier Analysis. J Appl Phys (1996) 80(5):2788–90. doi:10.1063/1.363196
29. Müller-Buschbaum P, Gutmann JS, Cubitt R, Stamm M. Probing the In-Plane Composition of Thin Polymer Films with Grazing-Incidence Small-Angle Neutron Scattering and Atomic Force Microscopy. Colloid Polym Sci (1999) 277(12):1193–9. doi:10.1007/s003960050509
30. Romanov VP, Ul'yanov SV. Dynamic and Correlation Properties of Solid Supported Smectic-A Films. Phys Rev E (2002) 66(6):9. doi:10.1103/physreve.66.061701
31. Böckmann RA, Hac A, Heimburg T, Grubmüller H. Effect of Sodium Chloride on a Lipid Bilayer. Biophysical J (2003) 85(3):1647–55. doi:10.1016/s0006-3495(03)74594-9
32. Valley CC, Perlmutter JD, Braun AR, Sachs JN. NaCl Interactions with Phosphatidylcholine Bilayers Do Not Alter Membrane Structure but Induce Long-Range Ordering of Ions and Water. J Membr Biol (2011) 244(1):35–42. doi:10.1007/s00232-011-9395-1
33. Cornell BA, Separovic F. Membrane Thickness and Acyl Chain Length. Biochim Biophys Acta (BBA) - Biomembranes (1983) 733(1):189–93. doi:10.1016/0005-2736(83)90106-2
34. Jaksch S, Lipfert F, Koutsioubas A, Mattauch S, Holderer O, Ivanova O, et al. Influence of Ibuprofen on Phospholipid Membranes. Phys Rev E (2015) 91(2):022716. doi:10.1103/physreve.91.022716
35. Lvov J, Mogilevskij L, Fejgin L, Györgyi S, Ronto G, Thompson K, et al. Structural Parameters of Phosphatidylcholine Bilayer Membranes. Mol crystals liquid crystals (1986) 133(1-2):65–73. doi:10.1080/00268948608079561
36. Sharqawy MH, Lienhard JH, Zubair SM. Thermophysical Properties of Seawater: a Review of Existing Correlations and Data. Desalination Water Treat (2010) 16(1-3):354–80. doi:10.5004/dwt.2010.1079
37. Ramshaw JD. Debye-Hückel Theory for Rigid‐dipole Fluids. J Chem Phys (1976) 64(9):3666–9. doi:10.1063/1.432730
38. Debye Pv., Hückel E. Zur Theorie der Elektrolyte: I. Gefrierpunktserniedrigung und verwandte Erscheinungen; II. Das Grenzgesetz für die elektrische Leitfähigkeit (On the theory of electrolytes: I. Lowering of the freezing point and related phenomena; II. The limiting laws for the electrical conductivity). Phys Z (1923) 24:185.
39. Lekkerkerker HNW, Kegel WK, Overbeek JTG. Phase Behavior of Ionic Microemulsions. Berichte der Bunsengesellschaft für physikalische Chem (1996) 100(3):206–17. doi:10.1002/bbpc.19961000305
Keywords: phospholipids, salt, dynamics, structure, GISANS, neutron reflectometry, neutron spin-echo, GINSES
Citation: Jaksch S, Holderer O, Frielinghaus H, Koutsioubas A, Zolnierczuk P, Hayward DW, Förster S and Müller-Buschbaum P (2021) Influence of NaCl on the Structure and Dynamics of Phospholipid Layers. Front. Phys. 9:628219. doi: 10.3389/fphy.2021.628219
Received: 11 November 2020; Accepted: 30 April 2021;
Published: 14 May 2021.
Edited by:
Doo Soo Chung, Seoul National University, South KoreaReviewed by:
Victoria Garcia Sakai, ISIS Facility, United KingdomSadafara Anand Pillai, P P Savani University, India
Copyright © 2021 Jaksch, Holderer, Frielinghaus, Koutsioubas, Zolnierczuk, Hayward, Förster and Müller-Buschbaum. This is an open-access article distributed under the terms of the Creative Commons Attribution License (CC BY). The use, distribution or reproduction in other forums is permitted, provided the original author(s) and the copyright owner(s) are credited and that the original publication in this journal is cited, in accordance with accepted academic practice. No use, distribution or reproduction is permitted which does not comply with these terms.
*Correspondence: Sebastian Jaksch, cy5qYWtzY2hAZnotanVlbGljaC5kZQ==