- 1The Patrick G. Johnston Centre for Cancer Research, Queens University Belfast, Belfast, Northern Ireland
- 2Centre for Plasma Physics, School of Mathematics and Physics, Queen’s University Belfast, Belfast, Northern Ireland
- 3Central Laser Facility, Rutherford Appleton Laboratory, Science and Technology Facilities Council, Didcot, Oxford, United Kingdom
- 4Institute of Physics ASCR, v.v.i. (FZU), ELI-Beamlines Project, Prague, Czech Republic
The use of particle accelerators in radiotherapy has significantly changed the therapeutic outcomes for many types of solid tumours. In particular, protons are well known for sparing normal tissues and increasing the overall therapeutic index. Recent studies show that normal tissue sparing can be further enhanced through proton delivery at 100 Gy/s and above, in the so-called FLASH regime. This has generated very significant interest in assessing the biological effects of proton pulses delivered at very high dose rates. Laser-accelerated proton beams have unique temporal emission properties, which can be exploited to deliver Gy level doses in single or multiple pulses at dose rates exceeding by many orders of magnitude those currently used in FLASH approaches. An extensive investigation of the radiobiology of laser-driven protons is therefore not only necessary for future clinical application, but also offers the opportunity of accessing yet untested regimes of radiobiology. This paper provides an updated review of the recent progress achieved in ultra-high dose rate radiobiology experiments employing laser-driven protons, including a brief discussion of the relevant methodology and dosimetry approaches.
Introduction
Radiotherapy is delivered to over 50% of cancer patients with curative intent for solid localized tumours [1]. Most radiotherapy facilities across the world still rely on high energy photon or electron sources [1]. With recent technological advances, modalities such as Intensity Modulated Radiotherapy (IMRT), Stereotactic Body Radiotherapy (SBRT) or Volumetric Modulated Arc Therapy (VMAT) can conform doses to tumours more precisely than possible a few decades ago sparing the normal tissue to a larger extent. However, the risk of exposure of the surrounding normal tissues remains a concern for patient outcomes [2], with the potential for late tissue damage and escalating the risk of initiating secondary cancers in a patient’s lifetime, especially for paediatric patients [3]. Proton therapy has been proposed as the most effective treatment of solid tumours in critical locations including the brain, medulloblastoma and other central nervous system tumours [4, 5]. The dose deposition profile of protons in the form of a “Bragg peak” imparts a unique normal tissue sparing ability [6] while depositing maximal dose within tumours [4, 7]. Due to this higher normal tissue sparing effect relative to any of the photon based modalities, proton therapy has drawn an increasing interest with globally over 150 proton therapy centres being operational and under construction or at the planning stage [8]. Several clinical trials have confirmed the benefit of proton therapy in patients with localized solid tumours giving enhanced quality of life and better tumour control [9–11].
While proton therapy is clearly envisaged to significantly benefit patients, the underlying operational and construction costs pose a significant obstacle for widespread access to this form of treatment [12–14]. Alternative strategies for ion acceleration to clinically relevant energies with a smaller footprint technology and at reduced costs have been pursued for a long time and in this direction high power lasers have been suggested as a potentially transformative technology (see [15–17] and references within). Thanks to the Chirped Pulse Amplification (CPA) technique, it is now possible to amplify ultra-short laser pulses to the Petawatt level, an approach which led to the award of 2018 Physics Nobel Prize to Strickland and Mourou [18]. Based on the application of CPA, several investigators have demonstrated the generation of high-energy laser-accelerated ions and developed an understanding of their physical properties as well as their upscaling through different approaches potentially enabling radiological and radiobiological application [15, 17, 19, 20]. The unique properties of laser-driven protons [15, 17] include ultra-high field acceleration gradient, high brightness with ∼1012 ions in picosecond-scale bunches, high laminarity, ultra-low emittance and scalable energy cut-off, ultra-short pulse duration, energy-dependent collimation which increases with proton energy. Furthermore, laser acceleration potentially allows controlling the accelerated ion species by changing the target material, as well as producing multispecies ion beams, a capability that could be valuable for mixed field irradiations, currently not possible even with the more advanced RF accelerators.
The vision of laser-driven ion acceleration for hadrontherapy was first proposed by Bulanov et al. [21] and further supported by Fourkal et al. and Malka et al. [22, 23]. This stimulated significant interest in the biomedical application of laser-accelerated ions, and has led to the demonstration of methods for handling and irradiating cell culture models in order to develop a radiobiological understanding of ion irradiations at the ultra-high dose rates deliverable with this acceleration technique (up to ∼109 Gray per second (Gy/s)).
The impact of dose rate on the biological effects and clinical response of radiation exposure has been studied for many years and several clinical modalities such as hypo- and hyper-fractionation have emerged based on the dose rate concept [24, 25]. Hall and Brenner reviewed the clinical importance of dose rate effects about 30 years ago, where a dose rate of 1011–1013 cGy/min was specifically defined as ultra-high dose rate [26] and a dose rate of 0.1–0.2 Gy/s was considered as a radiotherapy relevant dose rate or, in other words, a “conventional” dose rate. Since then, these conventional dose rates have been widely used in various modalities of external beam radiotherapy (reviewed by Ling et al. in Ref. 25).
Recently, numerous investigators have used dose rates significantly higher than the conventional ones, often in the context of FLASH radiotherapy where the dose rate usually ranges between 40 and 1000 Gy/s [27–30]. The term “ultra-high dose rate” is often used to indicate these “FLASH” dose rates, which is confusing and can lead to a misinterpretation of ultra-high dose-rate radiobiology. It is important to understand the differences between these various regimes, as the biological effects at each dose rate may involve a different mechanism of action. Recently, Vozenin et al. [31] have emphasized the importance of properly reporting beam parameters for the characterization of the biological effects of FLASH irradiations. They also point out that not all irradiations at ultra-high dose rate may lead to FLASH effects and thus to understand the differences between the two regimes is important. FLASH dose rate effects have been shown to involve the differential response of normal and cancer cells, in the removal and decay of hydroperoxide and other free radicals [32] and oxygen saturation during the irradiation mainly observed in-vivo [33]. This results in significant sparing of the normal cells and thus provides a larger therapeutic window compared to conventional dose rate irradiation [34]. Durante et al. [35] introduced a Dose Rate Effectiveness Factor (DREF), and defined the radiobiological effectiveness of exposures ranging from low to Flash dose rates. However the behaviour of DREF at ultra-high dose rates of 109–1010 Gy/s has not yet been clearly defined, as indicated in Figure 1. In order to capitalize on any future radiobiological applications of laser-driven protons it is imperative to properly understand the effects arising at such dose rates. This manuscript aims to present an update on recent radiobiological research in this area with an overview of the methodology, mechanisms, dosimetry approaches and radiobiological assays employed to study the radiobiology of laser-accelerated protons.
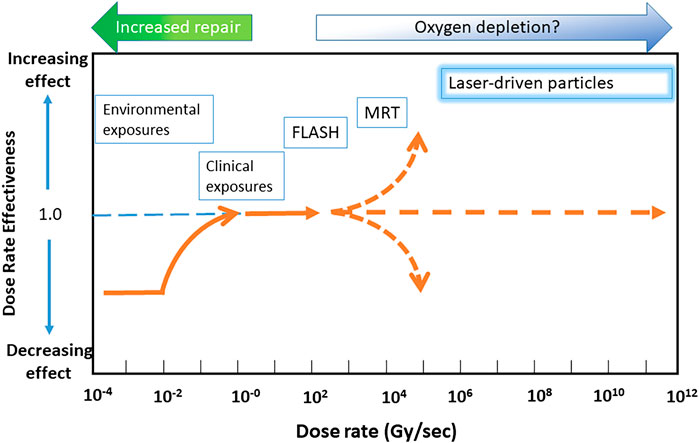
FIGURE 1. Representation of dose rate effectiveness as a function of dose rate. Y-axis shows the dose rate effectiveness and X–axis shows the dose rate. Various radiotherapy modalities are displayed according to their approximate DREF values and dose rates. Abbreviations: MRT, Microbeam Radiotherapy.
Laser-Driven Proton Acceleration
Research on the acceleration of ions by ultra-intense laser pulses has been pursued over the last 2 decades, and has driven continual progress in delivering higher laser intensities on target, creating new target types and improving diagnostic systems underpinning new scientific results.
Research on the acceleration of ions by ultra-intense laser pulses is mostly based on interactions with solid targets. In a typical experimental setting, as shown in Figure 2, an intense laser pulse, typically at intensities above 1019 W/cm2, interacts with a thin foil with thickness in the order of µms to 10s of µm and, during this interaction, a significant amount of laser energy is transferred to a population of relativistic electrons. These energetic electrons propagate through the target and create a strong sheath electric field at its rear surface, leading to the acceleration of protons originally present as impurities on the target surfaces. This Target Normal Sheath Acceleration (TNSA) process [36], is currently the most explored and robust mechanism of laser-driven proton acceleration [15, 17, 20, 37, 38]. Nevertheless, further improvements in cut-off energy, spectral and angular properties and repetition rate are required for effective application of laser-accelerated beams. For instance, applications in cancer therapy would require the delivery of high-energy protons (60–250 MeV) with a narrow energy spread and sufficient particle flux at appropriate distances from the interaction targets, so that any extraneous radiation produced during the intense laser interaction can be shielded adequately.
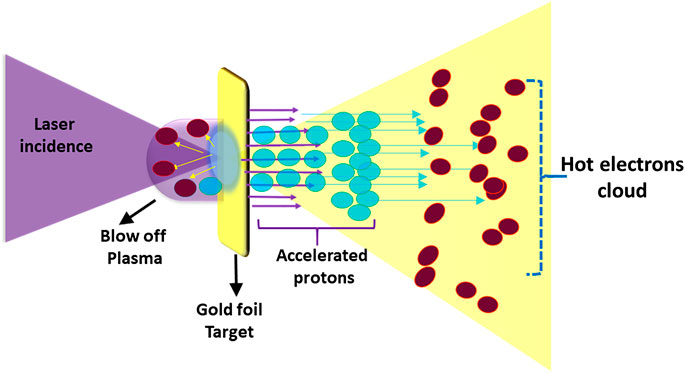
FIGURE 2. Target Normal Sheath Acceleration mechanism of laser driven ions acceleration. The laser is focused onto in (5–10 μm) foil targets (e.g., gold as in the example shown) generating a beam of hot electrons which propagate through the target, and set-up a large sheath field at its rear. This field can reach up to a few Tera Volts per metre (TV/m) ionizing the atoms present at the target surface (inlcuding contaminants). The ions are then accelerated in the sheath field along the target normal direction.
While control of TNSA beams has been a topic of active research over the last decade, significant attention has also been devoted to the exploration of a number of new acceleration mechanisms, including, for example, radiation pressure acceleration (RPA), which can in principle, produce beams with smaller divergence and narrow energy spread, particularly in the Light-Sail (LS) regime [15, 39]. RPA approaches are particularly suited to the acceleration of bulk ions in the irradiated target (e.g., carbon ions as opposed to contaminant protons [40]). There has recently been significant progress in increasing the maximum proton energies delivered through laser-driven processes, with reports of the acceleration of near 100 MeV protons by hybrid acceleration schemes [41] where acceleration is initiated by the TNSA mechanism followed by RPA until the target becomes transparent, with a further energy boost provided in a relativistic transparency regime.
Main Centres Involved in the Radiobiology Study of Laser-Driven Proton
Motivated by its potential applications in research, technology and medicine, laser-driven proton acceleration research has gained significant support from funding agencies and academia in many countries. A number of laboratories across Europe have a specific interest and focus on the radiobiological and clinical applications of laser-driven proton. In Germany, Ludwig Maximilian University (LMU) Munich (currently starting activities at the new Centre for Advanced Laser Applications, CALA) and Helmholtz-Zentrum Dresden- Rossendorf (HZDR) have been very actively involved in radiobiological applications of laser-driven protons and several publications have resulted from the work performed at these facilities (discussed later). Investigators in France, are also actively engaged in radiobiology research with laser-driven beams, particularly at Laboratoire d’Optique (LOA), Palaiseau [42, 43]. A focus for future activities in this area across Europe will be at the facilities of the Extreme Light Infrastructure (ELI), particularly at ELI Beamlines (Czech Republic), where the Extreme Light Infrastructure Multidisciplinary Applications of Laser-Ion Acceleration (ELIMAIA) beam lines in Prague are being commissioned [44]. ELI Nuclear Physics (ELI NP) Romania, is also planning an involvement in laser-driven ion radiobiology research [45]. In the United Kingdom, the main facilities used for this research are located at the Central Laser Facility of the Rutherford Appleton Laboratory (RAL) (GEMINI and VULCAN lasers), the University of Strathclyde (SCAPA) and Queen’s University Belfast (TARANIS), with activities in this area carried out so far within the EPSRC-funded A-SAIL consortium. In Asia, pioneering activities in this area were carried out in Japan at APRC-JAEA (now QST) [46, 47], while a proton beamline for radiobiology applications (CLAPA) has recently been developed at Peking University [48].
Typical Set Up for Laser-Driven Proton Irradiation
An example of an arrangement for radiobiology experiments employing laser-driven protons is shown in Figures 3A–C (based on the set-up employed for several experiments on the VULCAN and ASTRA GEMINI laser systems [49, 50]). Amplified laser light enters the evacuated interaction chamber and, after reflection from a plasma mirror (used for contrast enhancement), the laser is focused within a spot of a few microns onto a thin target, as shown in Figure 3A. The ions accelerated from the target are spatially selected by a narrow slit (of a few hundred μm) mounted in front of a ∼1 T magnet (Figure 3B), which deflects the positively charged protons and other ions at an angle from the target normal separating them from other emitted radiation (electrons, X-rays). The deflected ions finally pass through a 50-200 μm-thick Kapton exit window (Figure 3C) and irradiate the cell sample positioned immediately after the window inside a sample holder. The sample holder is often a stainless steel dish covered with a thin Mylar foil (0.9–4 µm) which allows the ions to pass through with minimal beam attenuation, even at moderate energies.
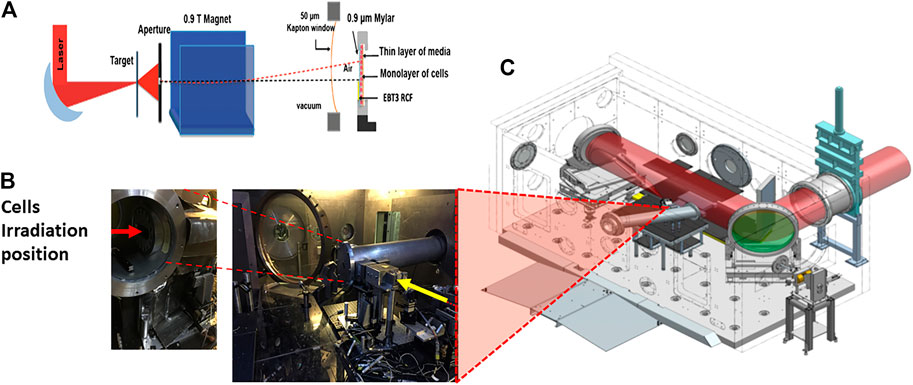
FIGURE 3. Experimental set up demonstrating laser driven proton acceleration. (A) Schematic of the arrangement of the target, aperture, magnet, beam exit and cells. (B) Actual image showing the laser target interaction point setup along with the beam exit and sample irradiation position. (C) Overview of the Laser Interaction chamber of the Vulcan Target Area Pettawatt Laser of the Central Laser Facility at the Rutherford Appleton laboratory, Didcot, Oxford, England.
Diagnostics and Dosimetry
The monitoring of laser-accelerated ion parameters, such as beam composition, energy spectrum and dose distribution at the irradiation point is fundamental to achieving a controlled and accurate cell irradiation. Bolton et al. have provided an extensive update on the instrumentation for diagnosis and control of laser-accelerated proton beams in Ref. 51. The beam characteristics are typically diagnosed using a range of techniques and detectors such as Thomson Parabola Spectrometers (TPS), Radiochromic Films (RCF) and Time of Flight (TOF) detectors [51]. The TPS and the RCF are usually placed along the target-normal direction to measure the higher-energy component and used for the full beam characterization in advance of the cell irradiations. In contrast, TOF detectors (typically diamond and silicon carbide) [52, 53] can be placed at various angles both in the forward and backward directions to monitor the particle flux and energy on-line during the irradiation, and detect any shot-to-shot variations [54]. A crucial task in view of meaningful radiobiological investigations is a precise measurement of the dose delivered to the cells. For conventional clinical beams, since the 2000s, a protocol for proton/ion dose measurement has been established where ionization chambers and calorimeters are defined as the absolute reference dosimeters [55]. In contrast, novel features of laser-driven protons such as ultra-high dose-rate, short pulse duration, as well as the presence of large electromagnetic pulses [56] generated by the laser-target interaction, require the development of new approaches and protocols for dose measurements [57].
The use of ionization chambers with pulsed beams at high dose rates is complicated due to an increase in ion recombination. Several authors have measured correction factors for Markus or Roos chambers developing new calibration procedures and models for both electrons and protons under different conditions relevant to FLASH radiotherapy [58–60]. They mainly used radiofrequency cavity (RF) accelerated protons up to 26 Gy/min and electrons up to 107 Gy/s. Extending the use of ionization chambers as absolute dosimeters for laser-driven protons at dose-rates near 109 Gy/s and at therapeutic doses (1–10 Gy) delivered per pulse, as typical in single-shot radiobiology experiments, is however a significant challenge, as it requires a large correction factor for the ion collection efficiency, which may affect the reliability of the dose measurement, as pointed out by McManus et al. [59].
The use of Faraday cups as suitable online dosimeters for laser-driven protons has also been proposed, e.g., by Richter et al. [61], who developed an integrated dosimetry and cell irradiation system (IDOCIS) that had about 13% dose uncertainty based on the quadratic summation of various contributing factors. An absolute dosimetry approach based on the use of Faraday cups is also being pursued on the ELIMED beamline at ELI Beamlines [62, 63].
In the attempt to further reduce dose uncertainties with laser-driven protons, an alternative approach employing graphite calorimeters as absolute dosimeters for laser-driven protons is under investigation at the National Physical Laboratory (NPL) in the framework of the European- Joint Research Project “UHDPulse” [57], aimed at establishing protocols and procedures for the dosimetry of ultra-high-pulse electron and proton beams [57, 64]. The measurement of the temperature increase in the calorimeter core upon irradiation is a direct measurement of the energy and dose deposited [65]. A first proof-of-principle experiment employing the high-energy laser-driven protons accelerated with the Vulcan PW laser at Rutherford Appleton Laboratory (RAL) has been reported in Ref. 66.
The use of passive detectors such as radiochromic films (Gafchromic EBT2 or EBT3), previously calibrated with beams generated by RF accelerators is a simple and widespread technique for dose measurements in laser-based radiobiology experiments [67, 68]. A single layer of the film can be used for 2D (transverse) monitoring of the proton dose, while stacks of multiple layers can be used for 3D dose mapping and reconstruction of the local proton spectrum by using deconvolution procedures. In a set-up as shown in Figure 3A, depending on the energy of the protons and cell dish arrangement, the RCF films can be placed either behind or in front of the cell dish. On a single-shot radiobiology experiment, this provides shot-to-shot monitoring of the dose, although this is available only after scanning of the film, using a suitably calibrated scanner. Recently RCF dosimetry (employing customized, unlaminated EBT3 films) has also been demonstrated with laser-accelerated carbon ions [50].
Real time dose monitoring during an irradiation is also crucial for an accurate characterization of the radiobiological effects of ultra-high dose rate irradiations. In radiobiology experiments at conventional accelerators, large area transmission ionization chambers are typically calibrated against the absolute dosimeter and then used as current monitors during the irradiation. In a laser-driven context, this is particularly important in multi-shot radiobiological experiments (as well as in future in-vivo experiments) to control the dose delivered in every single laser shot. Commercially available standard transmission ionization chambers have been used to monitor dose stability and reproducibility, e.g., on experiments on the Draco laser facility at HZDR, where standard transmission chambers cross-calibrated against radiochromic film at the cell irradiation position have been employed effectively (as described in Refs. 69 and 70). Modified transmission ionization chambers based on a double-gap structure [63] have been developed for dose monitoring along the ELIMED beam line, where the second gap is used to quantify, on each shot, the ion recombination occurring in the first gap at high dose-rates and to correct the collection efficiency.
Other approaches based on modified ionization chambers, such as the DOSION beam monitoring equipment [71], have been applied successfully in FLASH regimes [57], and may have scope for application with laser-driven sources. Several real-time approaches employing volumetric scintillation detectors [72], fibre optic dosimeters [73, 74], radioluminescence and Cherenkov emission based dosimeters have also been used for conventional dose rate proton beams as well as FLASH protons. In a laser-driven context, the use of scintillator blocks for approximated online monitoring of the lateral and depth dose distribution of the proton beam has been reported in Ref. 70, while approaches based on optical fibre arrays have not yet been applied to laser-driven protons. Finally, an approach for depth dose reconstruction employing acoustic traces generated by laser-driven protons stopping in a water phantom has been demonstrated and reported in Ref. 75.
Radiobiological Approaches
Radiation effects were first observed as a function of dose rate in the 1950s by Brasch et al. [76]. In 1969, Prempree et al. [77] studied the effects on the repair time of chromosome breaks induced by X-rays delivered at a dose rate of 4.5 × 108 Gy/s. Later, Berry in 1972–73 compared the effects of radiation dose rate from protracted, continuous irradiation to ultra-high dose rates of 109 Gy/s from pulsed accelerators [78, 79], noticed some non-predictable effects and discussed the impact of these dose rates on responses under hypoxia. Weiss et al. [80] demonstrated the depletion of oxygen in mammalian cells after ultra-high dose rate exposure. In all these studies, pulsed electron beams were mainly used and the pulse duration was of the order of a microsecond to a nanosecond. It was another two to 3 decades before studies were possible with laser-driven protons at comparable dose rates. During the early years of laser-driven proton radiobiology research, most experiments relied on multi-shot dose delivery, where several shots were required to deliver Gy level doses, and detect biological changes [81, 82]. Yogo et al. [81] delivered 200 shots to deposit a dose of 20 Gy in A549 lung adenocarcinoma cells that translates to an effective dose rate of 0.1 Gy/s with a single bunch dose rate of 1 × 107 Gy/s. Similarly, Kraft et al. [82] irradiated SKX squamous carcinoma cells using 12, 20, and 29 pulses, respectively, for low (1.5 Gy), medium (2.7 Gy) and high (4.1 Gy) doses of laser driven protons, demonstrating the feasibility of irradiating human cells with laser driven ions. These studies all employed femtosecond Titanium: Sapphire systems with limited energy per pulse, but were able to operate at sufficiently high repetition rates to make these multiple shot irradiations feasible.
A different approach, used by other groups, has been that of delivering Gy level doses in a single short burst of ∼ns duration, therefore reaching dose rates of ∼109 Gy/s. This has been possible on higher energy systems, typically delivering 10s–100s J per pulse [49, 83] or by focusing tightly the protons into a small spot [84]. Arguably, the approach employing single-shot irradiation, where the dose is not averaged over time, could in principle be better suited to test any biological effect related to the ultra-high dose rate.
The radiobiological assays that were applied in the above studies and also used more recently in laser-driven proton radiobiology research are briefly summarised in Figure 4, which includes endpoints for both the lethal and sub-lethal effects of radiation described in the following sub-sections.
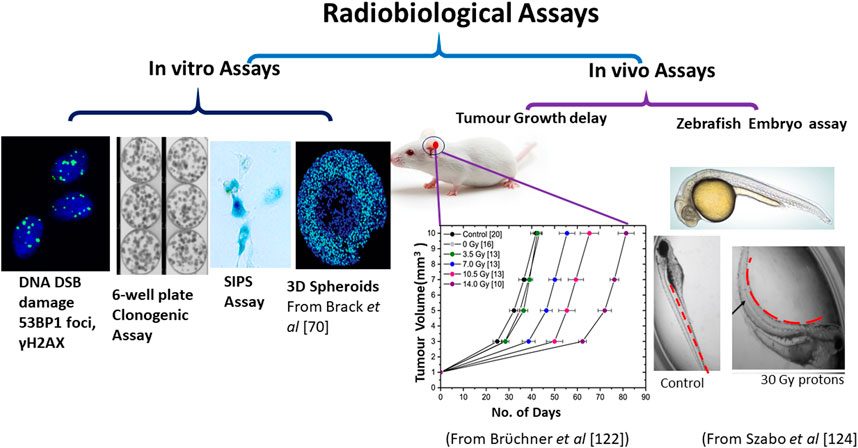
FIGURE 4. Main radiobiological models used with laser-driven ions. The models are classified as in-vitro or cellular models and in vivo or animal models. The in-vitro models mainly used the DNA DSB damage assay using γ-H2AX or 53BP1 foci formation (in both 2D monolayers and 3D spheroids), stress induced pre-mature senescence (SIPS) and clonogenic assay. The in-vivo or animal model studies mainly used the tumour growth delay assay (mice ear tumour) as shown by Brüchner et al. [122] or organ deformation assays in zebrafish model as shown by Szabó et al. [124].
Cell Based In-Vitro Studies
DNA Double Strand Break (DSB) Damage
The first experiments aimed at understanding the radiobiological effects of laser-driven protons quantified the yields of DNA damage in the form of DNA DSB using the foci formation assay. This assay uses immunofluorescent detection of the phosphorylated form (γ-H2AX) of the histone variant H2AX. The phosphorylation of the serine 139 amino acid residue on the H2AX protein is an early cellular response to DNA double strand break damage. The role of γ-H2AX in the formation of DNA DSB was first proposed by Rogakou et al. [85]. Sedelnikova et al. [86] found a direct correlation between the number of γ-H2AX foci and the number of radioiodine decay induced DNA DSBs providing a quantitative relationship for DNA DSB damage in human cell lines. Since then the γ-H2AX assay has been widely used in radiobiology and for over a decade has been an important biomarker of radiation induced DNA DSB damage from various type of radiations including low linear energy transfer (LET) X-rays [87], protons and high LET ions such as silicon, iron [88] and carbon ions [89, 90]. Subsequently, additional biomarkers related to γ-H2AX were also identified which phosphorylate in the close proximity of DNA DSB. Among these, the-p53 binding protein-1(53BP1) protein which recruits to the nuclear structures at the site of DNA damage and forms readily visualized ionizing radiation induced foci [91, 92] showed a good correlation with γ-H2AX [93, 94]. This has been used by numerous investigators, including in our own work quantifying proton induced DNA DSB damage in human cells [95].
Laser-driven proton-induced DNA DSB damage in mammalian cells were first detected by using the γ-H2AX foci formation assay by Yogo et al. [81] in A549 lung adenocarcinoma cells monolayers irradiated with 2.4 MeV protons delivered in multiple 15 nanosecond pulses. Using a similar set up for cell handling Kraft et al. [82] also used a multi-shot approach to study DNA DSB damage induced by variable doses. In their study, Kraft et al. reached a dose rate of 4 × 107 Gy/s and irradiated cells with doses ranging from 1.4–4.5 Gy in about 12–29 pulses. Bin et al. [84] used a single shot approach and showed the induction of γ-H2AX foci in cervical cancer cells (Hela cells) exposed to 1 Gy dose of 4.45 MeV protons. In this study, the proton beam was generated using nanometre-thin, diamond-like carbon targets and delivered at dose rates in the range of 109–1010 Gy/s, reporting 7 Gy as the highest dose [84]. Overall, in these studies, laser-driven protons induced more DNA DSB damage than conventional X-rays but no comparison with cyclotron-accelerated protons, delivered at conventional dose-rates, was made.
Such a comparison was first made by Zeil et al. [96] who compared the yields of γ-H2AX foci induced by laser-driven protons to conventionally accelerated protons and found no significant differences. A few years later Raschke et al. [97] also found that laser-accelerated protons induce similar DSB damage to that induced by cyclotron-accelerated protons measured by γ-H2AX foci formation. In both of these studies, multi-shot irradiation methodology was used to deliver the desired doses.
Our group at QUB also performed single shot irradiation of human skin fibroblast cells (AG01522B) with 10 MeV laser-accelerated protons at a dose rate of 109 Gy/s. We compared the 53BP1 foci per cell per track induced by the laser driven ions to results obtained with cyclotron-accelerated protons at the same energy but at a dose rate of 0.06 Gy/s and also, in this case, did not observe any significant differences between the two dose-rates [49].
Clonogenic Cell Survival
The clonogenic assay has been used since the 1950s to study the radiation survival dose-response relationship for mammalian cell lines. Markus and Puck were the first to develop this assay [98] and since then it is considered a gold standard in radiation biology. Using this assay, the relative biological effectiveness (RBE), which is defined as the ratio of the doses required to cause the same biological effect using protons or other ions [99, 100] compared to reference X-ray radiation, can be calculated. RBE has contributed to a better understanding of radiotherapy dose treatment plans by providing the modeling parameters for various cell lines derived from tumour and normal tissues. Yogo et al. [46] for the first time used the clonogenic assay to determine the RBE of laser-driven protons using human salivary gland tumour cells and reported it as 1.2 ± 0.11 with reference to X-rays. In this study, the cells were irradiated with 2.25 MeV protons with a single bunch dose rate of 107 Gy/s. The cumulative doses were deposited using a multi-shot approach reducing the effective dose rate to 0.2 Gy/s on the cells. Later Doria et al. [83] also reported the cell killing effects of laser driven protons at a dose rate exceeding 109 Gy/s in V79 cells. This was the first study that employed the single shot delivery of 1–5 MeV protons at Gy level doses. Doses as high as 5 Gy were delivered in single pulses of picosecond duration. In this study an RBE of 1.4 ± 0.2 at a surviving fraction of 10% was reported.
Zeil et al. [96] compared the cell killing ability of pulsed laser-driven protons to a continuous proton beam when the squamous cell carcinoma cell line (SKX) was irradiated with energies above 6.5 MeV at a peak dose rate of 4 × 107 Gy/s. The authors did not explicitly quantify the RBE value in this paper, although the cell killing effects of the laser driven protons were reported to be similar to those delivered continuously from a Tandem accelerator at conventional dose-rates. Overall, based on the results from these papers, the RBE values for laser-driven protons ranged between 1.2 and 1.4, similar to those reported for RF-accelerated protons in the same energy range.
In summary, none of the studies discussed above highlighted significant deviations in biological response associated with the use of ultra-high dose rates provided by a laser-driven approach. Only recently, Bayart et al. [43] from the LOA group in France have reported that temporal delivery of laser-driven protons at an ultra-high dose rate of 1.5 × 108 Gy/s, may change the biological response of the human cells to synthetic lethality or cell killing induced by inhibiting the DNA damage response protein, PARP-1.
Several investigators have suggested that ultra-high dose rate exposure with electrons leads to oxygen depletion, inducing radioresistance in cells by reducing the reactive oxygen species-mediated indirect DNA DSB damage [33, 80]. When normoxic cells were irradiated with ultra-high dose rate electrons an increase in cell survival was noticed. However, there is limited information available on the response of hypoxic cells irradiated at ultra-high dose rates with protons, e.g., whether any additional DNA DSB induced are additive or synergistic in nature. We have tested this hypothesis experimentally by irradiating normal human skin fibroblast cells under hypoxic as well as normoxic conditions and we detected a significant increase in residual DNA DSB damage in hypoxic cells at 24 h post irradiation compared to the normoxic cells [101].
Sub-lethal Effects
Although a key advantage of hadrontherapy is that healthy tissues can be spared much more effectively than in X-ray radiotherapy, the sub-lethal effects induced by low dose deposition in the healthy cells surrounding a solid tumour may eventually result in the initiation of secondary cancers or other complications and are arguably as important as lethal effects for an effective radiotherapy treatment outcome. Sub-lethal effects have been demonstrated in the promotion of migration and invasion in brain tumours and liver cancer [102, 103]. Among various sub-lethal effects, cell senescence has been studied for a long time [104] and has been associated with an onset of inflammation [105], secondary tumours and cancer relapse [106] in patients undergoing radiotherapy [107–109]. Stress induced pre-mature senescence (SIPS), a process in which a sub-lethally damaged cell enters a permanent state of inactivity, has attracted interest for its possible long-term health implications after radiation exposures [110, 111]. It is different from the phenomenon of in-vitro replicative senescence, where a cell loses its ability to proliferate after a finite number of cell divisions. Normal tissue sparing and differential sub-lethal effects induction have been reported in several FLASH radiotherapy studies, and highlighted as the main advantage of this approach (e.g. in Ref. 30). Investigating whether these advantages extend to the ultra-high dose regime delivered by laser-driven ions is one of the current research objectives of laser-driven radiobiology. Manti et al. [112] irradiated human vein endothelial cells with laser-driven protons and found that laser-driven proton beams, at a dose rate of 109 Gy/s, yielded a higher number of senescent cells at quasi therapeutic doses, while causing a lower percentage of cells to enter pre-mature senescence at doses typical of healthy tissues. This work therefore provided indication of sparing effects at ultra-high dose rate. Raschke et al. [97] have shown that, while laser-driven protons induce similar levels of DNA DSB damage to cyclotron–accelerated protons, the levels of protein nitroxidation (a marker of non-targeted effects) as studied through 3- nitrotyrosine generation was lower with laser-driven proton as compared to cyclotron-accelerated protons or X-rays.
3D Cell Culture Models
3D spherical or organoid model systems have been suggested as a link between cellular in-vitro models and in vivo animal tumours [113] as they recapitulate cancer drug effects more closely to the effects observed in animal models [114, 115] and especially in Glioblastoma tumours [116, 117]. These 3D neurosphere models are highly relevant for laser-driven ion studies as the current energies achieved with laser driven ions may not be suitable for the uniform irradiation of mouse in-vivo tumours which have dimensions of a few millimetres to a centimetre. In contrast, these 3D spheres are about 500–700 μm (1 week after seeding) in diameter which enables complete irradiation of these neurospheres using laser driven ions beams with energies above 20 MeV. Recently, Brack et al. [70] have demonstrated the uniform irradiation of 3D spheroids of human tongue cancer cells with a solenoid focused laser-driven proton beam. They evaluated the impact of laser-driven protons on DNA DSB damage in 3D spheroids after a total deposited dose of 15.3 Gy delivered in multiple shots.
Animal Based In-Vivo Studies
Mice have been used in many biological studies ranging from pharmacology, drug development, and as disease models [118]. Radiation oncology has also benefitted from pre-clinical studies using the large variety of genetically modified mouse models [119, 120]. Using conventionally accelerated protons or external beam radiotherapy for in vivo experiments is however much easier than doing similar experiments with laser-driven proton beams since, as mentioned earlier, the beam energy attained with laser-driven protons is generally not yet sufficient to fully irradiate deep-seated tumours with the required doses.
Due to this limitation, one has to carefully choose the type of tumour suitable for studies with laser driven ions. A mouse ear tumor model has been recently proposed, which has been proven suitable for experiments with ∼20 MeV protons [121]. Laser-driven electrons have been used to irradiate the mouse ear tumour model and the tumour growth delay after irradiation was found comparable to X-ray induced growth delay [122]. To date no mouse based in-vivo irradiation has been reported with laser-driven protons, although they can be readily used with the above-mentioned models. The only published evidence of in-vivo research comes from a recent study published by Rösch et al. [123]. Due to a good match of the beam spot dimensions of laser-driven protons and the body size of zebrafish embryos, they can be potentially used as suitable in vivo models. 24 h after fertilization, these embryos can be easily handled, irradiated, and monitored for various radiobiological endpoints. One of the most accessible endpoints is the shape of the embryo's spine; whereas the un-irradiated controls are lengthy and have a straight spine, their irradiated counterparts are shorter in length and show a curved spine along with pericardial swelling and inhibition of yolk sac resorption (as described by Szabó et al. [124]).
Conclusion
While hadrontherapy was highlighted as a key application for laser-driven protons at an early stage of the development of laser acceleration, it is clear that direct application of laser-driven beams remains challenging, and significant progress is still needed to match the parameters required for clinical particle therapy. Efforts to upscale the energies, control the dose and improve the reliability of beam production are continuing, and there has been significant recent progress especially in developing advanced acceleration mechanisms, new techniques for beam transport and delivery, as well as novel beam diagnostics and dosimetry approaches. While further progress is being pursued, alternative applications of laser-driven protons can be exploited [125]. The answer to whether laser-driven protons at ultra-high dose rate can elicit a different biological response than conventional dose rate protons is still uncertain, but emerging indications do warrant continuation of this research, coupled to improvements in the dose delivery, dosimetry and energy range investigated. Although the maximum observed energy of laser-driven protons have recently approached 100 MeV, significant doses allowing radiobiology investigations have only been demonstrated up to ∼30 MeV. RF-accelerated proton beams at such energies may not have robust dosimetry, as the primary beam is typically degraded, which introduces uncertainties in the beam composition and range, and makes accurate comparisons challenging. For a better understanding of the radiobiological mechanisms at ultra-high dose rates there is therefore an urgent need to enhance the energy output of laser-driven proton beams, as well as to advance techniques for the uniform irradiation of large surface areas of cells in adherent monolayers or tissues within larger volumes (3D). Ultra-high dose rate radiobiology is a relatively new field and still evolving but certainly has great potential, as suggested by the recent advances of FLASH radiotherapy which has shown unique normal tissue sparing effects [30, 126, 127] that can lead to a higher therapeutic index in cancer radiotherapy [128–131]. There is no doubt that a close collaboration between the cyclotron accelerator, high power laser and FLASH radiotherapy communities will be beneficial for advancing the prospects of ultra-high dose rate proton radiobiology.
Author Contributions
PC, MB and KMP conceptualized the article. PC, GM, HA, BO and AM collected the literature, wrote various subsections. PC, KMP and MB finalized the manuscript. All authors listed have made a substantial, direct, and intellectual contribution to the work and approved it for publication.
Funding
We acknowledge funding from the Engineering and Physical Sciences Research Council (EPSRC), through Grants EP/K022415/1 and EP/P010059/1. This project 18HLT04 UHDpulse has received funding from the EMPIR programme co-financed by the Participating States and from the European Union's Horizon 2020 research and innovation programme. Additionally, BO acknowledges funding from the European Union’s Horizon 2020 research and innovation program under the Marie Sklowdowska-Curie grant agreement no 754507. AM acknowledges funding support provided by Department for the Economy, Northern Ireland.
Conflict of Interest
The authors declare that the research was conducted in the absence of any commercial or financial relationships that could be construed as a potential conflict of interest.
Acknowledgments
We would like to thank Kerstin Brüchner Elke Beyreuther and Florian-Emanuel Brack, HZDR, Dresden Germany and Emilia Rita Szabo at ELI- ALPS Hungary for allowing us to reuse material from their earlier published articles.
References
1. Citrin DE Recent developments in radiotherapy. N Engl J Med (2017) 377(11):1065–75. doi:10.1056/NEJMra1608986
2. Kinsara A, El Gizawy AS, Banoqitah E, Ma X Review of leakage from a linear accelerator and its side effects on cancer patients. J Nucl Med Radiat Ther (2016) 7(3):288. doi:10.4172/2155-9619.1000288
3. Yoon M, Ahn SH, Kim J, Shin DH, Park SY, Lee SB, et al. Radiation-induced cancers from modern radiotherapy techniques: intensity-modulated radiotherapy versus proton therapy. Int J Radiat Oncol Biol Phys (2010) 77(5):1477–85. doi:10.1016/j.ijrobp.2009.07.011
4. Durante M, Cucinotta FA, Loeffler JS Charged particles in oncology. Front Res Top (2018) 7:301. doi:10.3389/978-2-88945-391-7
5. Durante M Proton beam therapy in Europe: more centres need more research. Br J Cancer (2018) 120(8):777–8. doi:10.1038/s41416-018-0329-x
6. Bragg WH, Kleeman R XXXIX. On the α particles of radium, and their loss of range in passing through various atoms and molecules. Philos Mag J Sci (1905) 10(57):318–40. doi:10.1080/14786440509463378
7. Newhauser WD, Zhang R The physics of proton therapy. Phys Med Biol (2015) 60(8):R155–209. doi:10.1088/0031-9155/60/8/R155
8. Flanz J, Kamada T, Durante M, Jermann M, Mahajan A, et al. Proceedings to the 58th Annual Conference of the Particle Therapy Cooperative Group (PTCOG58) 10–15 June 2019. (2020). Int J Part Ther (2017) 6 (4):45–491. doi:10.14338/IJPT.19-PTCOG-6.4
9. Hu M, Jiang L, Cui X, Zhang J, Yu J Proton beam therapy for cancer in the era of precision medicine. J Hematol Oncol (2018) 11(1):1–16. doi:10.1186/s13045-018-0683-4
10. Ng J, Shuryak I Minimizing second cancer risk following radiotherapy: current perspectives. Cancer Manag Res (2014) 7:1–11. doi:10.2147/CMAR.S47220
11. Newhauser WD, Durante M Assessing the risk of second malignancies after modern radiotherapy. Nat Rev Cancer (2011) 11(6):438–48. doi:10.1038/nrc3069
12. Peeters A, Grutters JP, Pijls-Johannesma M, Reimoser S, De Ruysscher D, Severens JL, et al. How costly is particle therapy? cost analysis of external beam radiotherapy with carbon-ions, protons and photons. Radiother Oncol (2010) 95(1):45–53. doi:10.1016/j.radonc.2009.12.002
13. Newhauser WD, Zhang R, Jones TG, Giebeler A, Taddei PJ, Stewart RD, et al. Reducing the cost of proton radiation therapy: the feasibility of a streamlined treatment technique for prostate cancer. Cancers (2015) 7(2):688–705. doi:10.3390/cancers7020688
14. Schippers JM, Lomax A, Garonna A, Parodi K Can technological improvements reduce the cost of proton radiation therapy? Semin Radiat Oncol (2018) 28(2):150–9. doi:10.1016/j.semradonc.2017.11.007
15. Macchi A, Borghesi M, Passoni M. Ion acceleration by superintense laser interaction. Rev Mod Phys (2013) 85:751–793. doi:10.1103/RevModPhys.85.751
16. Ledingham K W, Bolton P R, Shikazono N, Ma CM Towards laser driven hadron cancer radiotherapy: a review of progress. Appl Sci (2014) 4:402‐443. doi:10.3390/app4030402
17. Schreiber J, Bolton PR, Parodi K “Hands-on” laser-driven ion acceleration: A primer for laser-driven source development and potential applications. Rev Sci Inst (2016) 87:071101. doi:10.1063/1.4959198
18. Strickland D, Mourou G Compression of amplified chirped optical pulses. Opt Commun (1985) 56(3):219–21. doi:10.1016/0030-4018(85)90120-8
19. Kar S, Ahmed H, Prasad R, Cerchez M, Brauckmann S, Aurand B, et al. Guided post-acceleration of laser-driven ions by a miniature modular structure. Nat Commun (2016) 7:10792. doi:10.1038/ncomms10792
20. Robson L, Simpson P T, Clarke RJ, Ledingham KWD, Lindau F, Lundh O, et al. Scaling of proton acceleration driven by petawatt-laser-plasma interactions. Nat Phys (2007) 3:58–62. doi:10.1038/nphys476
21. Bulanov SV, Khoroshkov VS Feasibility of using laser ion accelerators in proton therapy. Plasma Phys Rep (2001) 28(5):453–6. doi:10.1134/1.1478534
22. Fourkal E, Li JS, Xiong W, Nahum A, Ma CM Intensity modulated radiation therapy using laser-accelerated protons: a Monte Carlo dosimetric study. Phys Med Biol (2003) 48(24):3977–4000. doi:10.1088/0031-9155/48/24/001
23. Malka V, Fritzler S, Lefebvre E, d’Humières E, Ferrand R, Grillon G, et al. Practicability of protontherapy using compact laser systems. Med Phys (2004) 31(6):1587–92. doi:10.1118/1.1747751
24. Dale RG Dose-rate effects in targeted radiotherapy. Phys Med Biol (1996) 41(10):1871–84. doi:10.1088/0031-9155/41/10/001
25. Ling CC, Gerweck LE, Zaider M, Yorke E Dose-rate effects in external beam radiotherapy redux. Radiother Oncol (2010) 95(3):261–8. doi:10.1016/j.radonc.2010.03.014
26. Hall EJ, Brenner DJ The dose-rate effect revisited: radiobiological considerations of importance in radiotherapy. Int J Radiat Oncol Biol Phys (1991) 21(6):1403–14. doi:10.1016/0360-3016(91)90314-T
27. Fouillade C, Curras-Alonso S, Giuranno L, Quelennec E, Heinrich S, Bonnet-Boissinot S, et al. FLASH irradiation spares lung progenitor cells and limits the incidence of radio-induced senescence. Clin Cancer Res (2019) 26(6):1497–506. doi:10.1158/1078-0432.ccr-19-1440
28. Venkatesulu BP, Sharma A, Pollard-Larkin JM, Sadagopan R, Symons J, Neri S, et al. Ultra high dose rate (35 Gy/sec) radiation does not spare the normal tissue in cardiac and splenic models of lymphopenia and gastrointestinal syndrome. Sci Rep (2019) 9(1):17180. doi:10.1038/s41598-019-53562-y
29. Wilson JD, Hammond EM, Higgins GS, Petersson K Ultra-high dose rate (FLASH) radiotherapy: silver bullet or fool’s gold?. Front Oncol (2020) 9:1563. doi:10.3389/fonc.2019.01563
30. Montay-Gruel P, Petersson K, Jaccard M, Boivin G, Germond JF, Petit B, et al. Irradiation in a flash: unique sparing of memory in mice after whole brain irradiation with dose rates above 100Gy/s. Radiother Oncol (2017) 124(3):365–9. doi:10.1016/j.radonc.2017.05.003
31. Vozenin M-C, Montay-Gruel P, Limoli C, Germond JF All irradiations that are ultra-high dose rate may not be FLASH: the critical importance of beam parameter characterization and in vivo validation of the FLASH effect. Radiat Res (2020) 194(6) 571–2. doi:10.1667/RADE-20-00141.1
32. Spitz DR, Buettner GR, Petronek MS, St-Aubin JJ, Flynn RT, Waldron TJ, et al. An integrated physico-chemical approach for explaining the differential impact of FLASH versus conventional dose rate irradiation on cancer and normal tissue responses. Radiother Oncol (2019) 139:23–7. doi:10.1016/j.radonc.2019.03.028
33. Adrian G, Konradsson E, Lempart M, Bäck S, Ceberg C, Petersson K The FLASH effect depends on oxygen concentration. Br J Radiol (2020) 93(1106):20190702. doi:10.1259/bjr.20190702
34. Martin S, Prise KM, Hill MA Pushing the frontiers of radiobiology: a special feature in memory of sir oliver scott and Professor Jack fowler. Br J Radiol (2019) 92(1093):20189005. doi:10.1259/bjr.20189005
35. Durante M, Bräuer-Krisch E, Hill M Faster and safer? FLASH ultra-high dose rate in radiotherapy. Br J Radiol (2018) 91:20170628. doi:10.1259/bjr.20170628
36. Wilks SC, Langdon AB, Cowan TE, Roth M, Singh M, Hatchett S, et al. Energetic proton generation in ultra-intense laser–solid interactions. Phys Plasmas (2000), 8:542. doi:10.1063/1.1333697
37. Fuchs J, Antici P, d'Humieres E, Lefevbre E, Borghesi M, Brambrink E, et al. Laser-driven proton scaling laws and new paths towards energy increase. Nature Phys (2006), 2:48–54. doi:10.1038/nphys199
38. Wagner F, Deppert O, Brabetz C, Fiala P, Kleinschmidt A, Poth P, et al. Maximum Proton Energy above 85 MeV from the Relativistic Interaction of Laser Pulses with Micrometer Thick CH2 Targets. Phys. Rev.Lett (2016) 116:20500. doi:10.1103/PhysRevLett.116.205002
39. Macchi A Theory of light sail acceleration by intense lasers: an overview. High Power Laser Science and Engineering 2, (2014) e10. doi:10.1017/hpl.2014.13
40. Scullion C, Doria D, Romagnani L, Sgattoni A, Naughton K, et al. Polarization dependence of bulk Ion acceleration from Ultrathin foils irradiated by high-intensity ultrashort laser pulses. Phys Rev Lett (2017) 119, 054801. doi:10.1103/PhysRevLett.119.054801
41. Higginson A, Gray RJ, King M, Dance RJ, Williamson SDR Butler NMH et al. Near 100 MeV protons via a laser driven transparency enhanced hybrid acceleration scheme. Nat Commun. (2018) 9:724. doi:10.1038/s41467-018-03063-9
42. Pommarel L, Vauzour B, Megnin-Chanet F, Bayart E, Delmas O, Goudjil F, et al. Spectral and spatial shaping of a laser-produced ion beam for radiation-biology experiments. Phys Rev Acc Beams (2017) 20:082301. doi:10.1103/PhysRevAccelBeams.20.03280143
43. Bayart E, Flacco A, Delmas O, Pommarel L, Levy D, Cavallone M, et al. Fast dose fractionation using ultra-short laser accelerated proton pulses can increase cancer cell mortality, which relies on functional PARP1 protein. Sci Rep (2019) 9:10132. doi:10.1038/s41598-019-46512-1
44. Margarone D, Cirrone G, Cuttone G, Amico A, Andò L, Borghesi M, et al. ELIMAIA: a laser-driven ion accelerator for multidisciplinary applications. Quantum Beam Sci (2018) 2(2):8. doi:10.3390/qubs2020008
45. Asavei T, Bobeica M, Nastasa V, Manda G, Naftanaila F, Bratu O, et al. Laser-driven radiation: biomarkers for molecular imaging of high dose-rate effects. Med Phys (2019) 46(10):e726–34. doi:10.1002/mp.13741
46. Yogo A, Maeda T, Hori T, Sakaki H, Ogura K, Nishiuchi M, et al. Measurement of relative biological effectiveness of protons in human cancer cells using a laser-driven quasimonoenergetic proton beamline. Appl Phys Lett (2011) 98(5):053701. doi:10.1063/1.3551623
47. Yogo A, Sato K, Nishikino M, Maeda T, Sakaki H, Hori T, et al. Measurement of DNA double-strand break yield in human cancer cells by high-current, short-duration bunches of laser-accelerated protons. Jpn J Appl Phys (2011) 50(10R):106401. doi:10.1143/JJAP.50.106401
48. Zhu JG, Wu MJ, Liao Q, Geng YX, Zhu K, Li CC, et al. Experimental demonstration of a laser proton accelerator with accurate beam control through image-relaying transport. Phys Rev Accel Beams (2019) 22(6):061302. doi:10.1103/PhysRevAccelBeams.22.061302
49. Hanton F, Chaudhary P, Doria D, Gwynne D, Maiorino C, Scullion C, et al. DNA DSB repair dynamics following irradiation with laser-driven protons at ultra-high dose rates. Sci Rep (2019) 9(1):4471. doi:10.1038/s41598-019-40339-6
50. Milluzzo G, Ahmed H, Romagnani L, Doria D, Chaudhary P, Maiorino C, et al. Dosimetry of laser-accelerated carbon ions for cell irradiation at ultra-high dose rate. J Phys Conf Ser (2020) 1596:012038. doi:10.1088/1742-6596/1596/1/012038
51. Bolton PR, Borghesi M, Brenner C, Carroll DC, De Martinis C, Fiorini F, et al. Instrumentation for diagnostics and control of laser accelerated proton (ion) beams. Phys Med (2014) 30 (3):255–70. doi:10.1016/j.ejmp.2013.09.00252
52. Margarone D, Krása J, Giuffrida L, Picciotto A, Torrisi L, Nowak T, et al. Full characterization of laser-accelerated ion beams using Faraday cup, silicon carbide, and single-crystal diamond detectors. J Appl Phys (2011) 109(10):103302. doi:10.1063/1.3585871
53. Milluzzo G, Scuderi V, Amico AG, Borghesi M, Cirrone GAP, Cuttone G, et al. Laser-accelerated ion beam diagnostics with TOF detectors for the ELIMED beam line. J Instrum (2017) 12(02):C02025. doi:10.1088/1748-0221/12/02/C02025
54. Scuderi V, Milluzzo G, Doria D, Alejo A, Amico AG, Booth N, et al. TOF diagnosis of laser accelerated, high-energy protons. Nucl Instrum Methods Phys Res Sect A Accel Spectrom Detect Assoc Equip (2020) 978:164364. doi:10.1016/j.nima.2020.164364
55. Mather SJ, Mansi L IAEA technical report series. Eur J Nucl Med Mol Imaging (2008) 35(5):1030–1. doi:10.1007/s00259-008-0767-4
56. Consoli F, Tikhonchuk VT, Bardon M, Bradford P, Carroll DC, Cikhardt J, et al. Laser produced electromagnetic pulses: generation, detection and mitigation. High Power Laser Sci Eng (2020) 8:e22. doi:10.1017/hpl.2020.13
57. Schüller A, Heinrich S, Fouillade C, Subiel A, De Marzi L, Romano F, et al. The European Joint Research Project UHDpulse–metrology for advanced radiotherapy using particle beams with ultra-high pulse dose rates. Phys Medica (2020) 80:134–50. doi:10.1016/j.ejmp.2020.09.020
58. Petersson K, Jaccard M, Germond JF, Buchillier T, Bochud F, Bourhis J, et al. High dose-per-pulse electron beam dosimetry–a model to correct for the ion recombination in the Advanced Markus ionization chamber. Med Phys (2017) 44(3):1157–67. doi:10.1002/mp.12111
59. McManus M, Romano F, Lee ND, Farabolini W, Gilardi A, Royle G, et al. The challenge of ionisation chamber dosimetry in ultra-short pulsed high dose-rate Very High Energy Electron beams. Sci Rep (2020) 10(1):9089. doi:10.1038/s41598-020-65819-y
60. Palmans H, Thomas R, Kacperek A Ion recombination correction in the Clatterbridge Centre of Oncology clinical proton beam. Phys Med Biol (2006) 51(4):903–17. doi:10.1088/0031-9155/51/4/010
61. Richter C, Kaluza M, Karsch L, Schlenvoigt HP, Schürer M, Sobiella M, et al. Dosimetry of laser-accelerated electron beams used for in vitro cell irradiation experiments. Radiat Meas (2011) 46(12):2006–9. doi:10.1016/j.radmeas.2011.04.019
62. Leanza R, Romano F, Scuderi V, Amico AG, Cuttone G, Larosa G, et al. Faraday cup: absolute dosimetry for ELIMED beam line. J Instrum (2017) 12(03):C03046. doi:10.1088/1748-0221/12/03/C03046
63. Scuderi V, Amato A, Amico AG, Borghesi M, Cirrone GAP, Cuttone G, et al. Diagnostics and dosimetry solutions for multidisciplinary applications at the ELIMAIA beamline. Appl Sci (2018) 8(9):1415. doi:10.3390/app8091415
64. Bourgouin A, Schüller A, Hackel T, Kranzer R, Poppinga D, Kapsch RP, et al. Calorimeter for real-time dosimetry of pulsed ultra-high dose rate electron beams. Front Phys (2020) 8:567340. doi:10.3389/fphy.2020.567340
65. Rossomme S, Palmans H, Thomas R, Lee N, Bailey M, Shipley D, et al. SU-E-T-146: reference dosimetry for protons and light-ion beams based on graphite calorimetry. Med Phys (2012) 39(6):3736–7. doi:10.1118/1.4735204
66. Romano F, Subiel A, McManus M, Lee ND, Palmans H, Thomas R, et al. Challenges in dosimetry of particle beams with ultra-high pulse dose rates. J Phys Conf Ser (2020) 1662:012028. doi:10.1088/1742-6596/1662/1/012028
67. Fiorini F, Kirby D, Borghesi M, Doria D, Jeynes JC, Kakolee KF, et al. Dosimetry and spectral analysis of a radiobiological experiment using laser-driven proton beams. Phys Med Biol (2011) 56(21):6969–82. doi:10.1088/0031-9155/56/21/013
68. Polin K, Doria D, Romagnani L, Chaudhary P, Cirrone GAP, Maiorino C, et al. Irradiation and dosimetry arrangement for a radiobiological experiment employing laser-accelerated protons. J Instrum (2019) 14(10):C10015. doi:10.1088/1748-0221/14/10/c10015
69. Richter C, Karsch L, Dammene Y, Kraft SD, Metzkes J, Schramm U, et al. A dosimetric system for quantitative cell irradiation experiments with laser-accelerated protons. Phys Med Biol (2011) 56(6):1529–43. doi:10.1088/0031-9155/56/6/002
70. Brack FE, Kroll F, Gaus L, Bernert C, Beyreuther E, Cowan TE, et al. Spectral and spatial shaping of laser-driven proton beams using a pulsed high-field magnet beamline. Sci Rep (2020) 10:9118. doi:10.1038/s41598-020-65775-7
71. Boissonnat G, Fontbonne JM, Balanzat E, Boumard F, Carniol B, Cassimi A. Characterization and performances of DOSION, a dosimetry equipment dedicated to radiobiology experiments taking place at GANIL. Nuclear Inst Meth A(2017) (2017) 856:1–6.doi:10.1016/j.nima.2016.12.04072
72. Beddar S Real-time volumetric scintillation dosimetry. J Phys Conf Ser (2015) 573:012005. doi:10.1088/1742-6596/573/1/012005
73. O’Keeffe S, Fitzpatrick C, Lewis E, Al-Shamma AI A review of optical fibre radiation dosimeters. Sens Rev (2008) 28(2):136–42. doi:10.1108/02602280810856705
74. Hoehr C, Morana A, Duhamel O, Capoen B, Trinczek M, Paillet P, et al. Novel Gd3+-doped silica-based optical fiber material for dosimetry in proton therapy. Sci Rep (2019) 9(1):16376. doi:10.1038/s41598-019-52608-5
75. Haffa D, Yang R, Bin J, Lehrack S, Brack FE, Ding H, et al. I-BEAT: ultrasonic method for online measurement of the energy distribution of a single ion bunch. Sci Rep (2019) 9(1):6714. doi:10.1038/s41598-019-42920-5
76. Brasch A, Huber W, Waly A Radiation effects as a function of dose rate. Arch Biochem Biophys (1952) 39:245–7. doi:10.1016/0003-9861(52)90287-7
77. Prempree T, Michelsen A, Merz T The repair time of chromosome breaks induced by pulsed x-rays on ultra-high dose-rate. Int J Radiat Biol Relat Stud Phys Chem Med (1969) 15(6):571–4. doi:10.1080/09553006914550871
78. Berry RJ Effects of radiation dose-rate from protracted, continuous irradiation to ultra-high dose-rates from pulsed accelerators. Br Med Bull (1973) 29(1):44–7. doi:10.1093/oxfordjournals.bmb.a070955
79. Berry RJ, Stedeford JB Reproductive survival of mammalian cells after irradiation at ultra-high dose-rates: further observations and their importance for radiotherapy. Br J Radiol (1972) 45(531):171–7. doi:10.1259/0007-1285-45-531-171
80. Weiss H, Epp ER, Heslin JM, Ling CC, Santomasso A Oxygen depletion in cells irradiated at ultra-high dose-rates and at conventional dose-rates. Int J Radiat Biol Relat Stud Phys Chem Med (1974) 26(1):17–29. doi:10.1080/09553007414550901
81. Yogo A, Sato K, Nishikino M, Mori M, Teshima T, Numasaki H, et al. Application of laser-accelerated protons to the demonstration of DNA double-strand breaks in human cancer cells. Appl Phys Lett (2009) 94(18):181502. doi:10.1063/1.3126452
82. Kraft SD, Richter C, Zeil K, Baumann M, Beyreuther E, Bock S, et al. Dose-dependent biological damage of tumour cells by laser-accelerated proton beams. New J Phys (2010) 12(8):085003. doi:10.1088/1367-2630/12/8/085003
83. Doria D, Kakolee KF, Kar S, Litt SK, Fiorini F, Ahmed H, et al. Biological effectiveness on live cells of laser driven protons at dose rates exceeding 109 Gy/s. AIP Adv (2012) 2(1):011209. doi:10.1063/1.3699063
84. Bin J, Allinger K, Assmann W, Dollinger G, Drexler GA, Friedl AA, et al. A laser-driven nanosecond proton source for radiobiological studies. Appl Phys Lett (2012) 101(24):243701. doi:10.1063/1.4769372
85. Rogakou EP, Pilch DR, Orr AH, Ivanova VS, Bonner WM DNA double-stranded breaks induce histone H2AX phosphorylation on serine 139. J Biol Chem (1998) 273(10):5858–68. doi:10.1074/jbc.273.10.5858
86. Sedelnikova OA, Rogakou EP, Panyutin IG, Bonner WM. Quantitative detection of 125IdU-induced DNA double-strand breaks with γ-H2AX antibody. Radiat Res (2002), 158(4): 468–492. doi:10.1667/0033-7587(2002)158[0486:QDOIID]2.0.CO;287
87. Ugenskiene R, Prise K, Folkard M, Lekki J, Stachura Z, Zazula M, et al. Dose response and kinetics of foci disappearance following exposure to high- and low-LET ionizing radiation. Int J Radiat Biol (2009) 85(10):872–82. doi:10.1080/09553000903072462
88. Desai N, Davis E, O'Neill P, Durante M, Cucinotta FA, Wu H Immunofluorescence detection of clustered gamma-H2AX foci induced by HZE-particle radiation. Radiat Res (2005) 164(4):518–22. doi:10.1667/rr3431.1
89. Ghosh S, Narang H, Sarma A, Kaur H, Krishna M Activation of DNA damage response signaling in lung adenocarcinoma A549 cells following oxygen beam irradiation. Mutat Res (2011) 723(2):190–8. doi:10.1016/j.mrgentox.2011.05.002
90. Schmid TE, Zlobinskaya O, Multhoff G Differences in phosphorylated histone H2AX foci formation and removal of cells exposed to low and high linear energy transfer radiation. Curr Genomics (2012) 13(6):418–25. doi:10.2174/138920212802510501
91. Schultz LB, Chehab NH, Malikzay A, Halazonetis TD P53 binding protein 1 (53BP1) is an early participant in the cellular response to DNA double-strand breaks. J Cell Biol (2000) 151(7):1381–90. doi:10.1083/jcb.151.7.1381
92. Gupta A, Hunt CR, Chakraborty S, Pandita RK, Yordy J, Ramnarain DB, et al. Role of 53BP1 in the regulation of DNA double-strand break repair pathway choice. Radiat Res (2014) 181(1):1–8. doi:10.1667/RR13572.1
93. Neumaier T, Swenson J, Pham C, Polyzos A, Lo AT, Yang P, et al. Evidence for formation of DNA repair centers and dose-response nonlinearity in human cells. Proc Natl Acad Sci USA (2012) 109(2):443–8. doi:10.1073/pnas.1117849108
94. Asaithamby A, Uematsu N, Chatterjee A, Story MD, Burma S, Chen DJ Repair of HZE-particle-induced DNA double-strand breaks in normal human fibroblasts. Radiat Res (2008) 169(4):437–46. doi:10.1667/RR1165.1
95. Chaudhary P, Marshall TI, Currell FJ, Kacperek A, Schettino G, Prise KM Variations in the processing of DNA double-strand breaks along 60-MeV therapeutic proton beams. Int J Radiat Oncol Biol Phys (2016) 95(1):86–94. doi:10.1016/j.ijrobp.2015.07.2279
96. Zeil K, Baumann M, Beyreuther E, Burris-Mog T, Cowan TE, Enghardt W, et al. Dose-controlled irradiation of cancer cells with laser-accelerated proton pulses. Appl Phys B (2013) 110(4):437–44. doi:10.1007/s00340-012-5275-3
97. Raschke S, Spickermann S, Toncian T, Swantusch M, Boeker J, Giesen U, et al. Ultra-short laser-accelerated proton pulses have similar DNA-damaging effectiveness but produce less immediate nitroxidative stress than conventional proton beams. Sci Rep (2016) 6(1):32441. doi:10.1038/srep32441
98. Puck TT, Marcus PI Action of x-rays on mammalian cells. J Exp Med (1956) 103(5):653–66. doi:10.1084/jem.103.5.653
99. Blomquist E, Russell KR, Stenerlöw B, Montelius A, Grusell E, Carlsson J Relative biological effectiveness of intermediate energy protons. Comparisons with 60Co gamma-radiation using two cell lines. Radiother Oncol (1993) 28(1):44–51. doi:10.1016/0167-8140(93)90184-A
100. Wouters BG, Lam GK, Oelfke U, Gardey K, Durand RE, Skarsgard LD Measurements of relative biological effectiveness of the 70 MeV proton beam at TRIUMF using Chinese hamster V79 cells and the high-precision cell sorter assay. Radiat Res (1996) 146(2):159–70. doi:10.2307/3579588
101. Chaudhary P, Gwynne D, Doria D, Romagnani L, Maiorino C, Padda H, et al. Laser accelerated ultra high dose rate protons induced DNA damage under hypoxic conditions. Radiother Oncol (2016) 118 (Suppl. 1):S24–5. doi:10.1016/S0167-8140(16)30049-4
102. Wild-Bode C, Weller M, Rimner A, Dichgans J, Wick W Sublethal irradiation promotes migration and invasiveness of glioma cells: implications for radiotherapy of human glioblastoma. Cancer Res (2001) 61(6):2744–50.
103. Chung YL, Jian JJ, Cheng SH, Tsai SY, Chuang VP, Soong T, et al. Sublethal irradiation induces vascular endothelial growth factor and promotes growth of hepatoma cells: implications for radiotherapy of hepatocellular carcinoma. Clin Cancer Res (2006) 12(9):2706–15. doi:10.1158/1078-0432.CCR-05-2721
104. Hayflick L, Moorhead PS The serial cultivation of human diploid cell strains. Exp Cell Res (1961) 25(3):585–621. doi:10.1016/0014-4827(61)90192-6
105. Pribluda A, Elyada E, Wiener Z, Hamza H, Goldstein RE, Biton M, et al. A Senescence-inflammatory switch from cancer-inhibitory to cancer-promoting mechanism. Cancer Cell (2013) 24(2):242–56. doi:10.1016/j.ccr.2013.06.005
106. Demaria M, O’Leary MN, Chang J, Shao L, Liu S, Alimirah F, et al. Cellular senescence promotes adverse effects of chemotherapy and cancer relapse. Cancer Discov (2017) 7(2):165–76. doi:10.1158/2159-8290.CD-16-0241
107. Nguyen HQ, To NH, Zadigue P, Kerbrat S, De La Taille A, Le Gouvello S, et al. Ionizing radiation-induced cellular senescence promotes tissue fibrosis after radiotherapy. a review. Crit Rev Oncol Hematol (2018) 129:13–26. doi:10.1016/j.critrevonc.2018.06.012
108. Tabasso AFS, Jones DJL, Jones GDD, Macip S Radiotherapy-induced senescence and its effects on responses to treatment. Clin Oncol (R Coll Radiol) (2019) 31(5):283–9. doi:10.1016/j.clon.2019.02.003
109. Jeon HY, Kim JK, Ham SW, Oh SY, Kim J, Park JB, et al. Irradiation induces glioblastoma cell senescence and senescence-associated secretory phenotype. Tumour Biol (2016) 37(5):5857–67. doi:10.1007/s13277-015-4439-2
110. Suzuki M, Boothman DA Stress-induced premature senescence (SIPS)–influence of SIPS on radiotherapy. J Radiat Res (2008) 49(2):105–12. doi:10.1269/jrr.07081
111. Goligorsky MS, Chen J, Patschan S Stress-induced premature senescence of endothelial cells: a perilous state between recovery and point of no return. Curr Opin Hematol (2009) 16(3):215–9. doi:10.1097/MOH.0b013e32832a07bd
112. Manti L, Perozziello FM, Borghesi M, Candiano G, Chaudhary P, Cirrone GAP, et al. The radiobiology of laser-driven particle beams: focus on sub-lethal responses of normal human cells. J Instrum (2017) 12 (03):C03084. doi:10.1088/1748-0221/12/03/C03084
113. Weiswald LB, Bellet D, Dangles-Marie V Spherical cancer models in tumor biology. Neoplasia (2015) 17(1):1–15. doi:10.1016/j.neo.2014.12.004
114. Estrada MF, Rebelo SP, Davies EJ, Pinto MT, Pereira H, Santo VE, et al. Modelling the tumour microenvironment in long-term microencapsulated 3D co-cultures recapitulates phenotypic features of disease progression. Biomaterials (2016) 78:50–61. doi:10.1016/j.biomaterials.2015.11.030
115. Azzarelli R Organoid models of glioblastoma to study brain tumor stem cells. Front Cell Dev Biol (2020) 8:220. doi:10.3389/fcell.2020.00220
116. Caragher S, Chalmers AJ, Gomez-Roman N Glioblastoma’s next top model: novel culture systems for brain cancer radiotherapy research. Cancers (2019) 11(1):44. doi:10.3390/cancers11010044
117. Gomez-Roman N, Stevenson K, Gilmour L, Hamilton G, Chalmers AJ A novel 3D human glioblastoma cell culture system for modeling drug and radiation responses. Neuro Oncol (2017) 19(2):229–41. doi:10.1093/neuonc/now164
118. Rosenthal N, Brown S The mouse ascending: perspectives for human-disease models. Nat Cell Biol (2007) 9(9):993–9. doi:10.1038/ncb437
119. Kahn J, Tofilon PJ, Camphausen K Preclinical models in radiation oncology. Radiat Oncol (2012) 7(1):223. doi:10.1186/1748-717X-7-223
120. Butterworth KT Evolution of the supermodel: progress in modelling radiotherapy response in mice. Clin Oncol (R Coll Radiol) (2019) 31(5):272–82. doi:10.1016/j.clon.2019.02.008
121. Beyreuther E, Brüchner K, Krause M, Schmidt M, Szabo R, Pawelke J An optimized small animal tumour model for experimentation with low energy protons. PLoS One (2017) 12(5):e0177428. doi:10.1371/journal.pone.0177428
122. Brüchner K, Beyreuther E, Baumann M, Krause M, Oppelt M, Pawelke J Establishment of a small animal tumour model for in vivo studies with low energy laser accelerated particles. Radiat Oncol (2014) 9:57. doi:10.1186/1748-717X-9-57
123. Rösch TF, Szabó Z, Haffa D, Bin J, Brunner S, Englbrecht FS, et al. A feasibility study of zebrafish embryo irradiation with laser-accelerated protons. Rev Sci Instrum (2020) 91(6):063303. doi:10.1063/5.0008512
124. Szabó ER, Brand M, Hans S, Hideghéty K, Karsch L, Lessmann E, et al. Radiobiological effects and proton RBE determined by wildtype zebrafish embryos. PLoS One (2018) 13(11):e0206879. doi:10.1371/journal.pone.0206879
125. Bolton PR, Parodi K, Schreiber J. Applications of laser-driven particle acceleration. Boca Raton, FL; London, United Kingdom; New York, NY: CRC Press Taylor and Francis Group (2018).
126. de Kruijff RM FLASH radiotherapy: ultra-high dose rates to spare healthy tissue. Int J Radiat Biol (2019) 96(4):419–23. doi:10.1080/09553002.2020.1704912
127. Zhou G Mechanisms underlying FLASH radiotherapy, a novel way to enlarge the differential responses to ionizing radiation between normal and tumor tissues. Radiat Med Prot (2020) 1(1):35–40. doi:10.1016/j.radmp.2020.02.002
128. Al-Hallaq H, Cao M, Kruse J, Klein E Cured in a FLASH: reducing normal tissue toxicities using ultra-high-dose rates. Int J Radiat Oncol Biol Phys (2019) 104(2):257–60. doi:10.1016/j.ijrobp.2019.01.093
129. Symonds P, Jones GDD FLASH radiotherapy: the next technological advance in radiation therapy?. Clin Oncol (R Coll Radiol) (2019) 31(7):405–6. doi:10.1016/j.clon.2019.05.011
130. Yilmaz MT, Hurmuz P, Yazici G FLASH-radiotherapy: a new perspective in immunotherapy era?. Radiother Oncol (2020) 145:137. doi:10.1016/j.radonc.2019.12.015
Keywords: protontherapy, cancer, radiobiology, laser-driven ions, particle accelerator, ultra-high dose rate
Citation: Chaudhary P, Milluzzo G, Ahmed H, Odlozilik B, McMurray A, Prise KM and Borghesi M (2021) Radiobiology Experiments With Ultra-high Dose Rate Laser-Driven Protons: Methodology and State-of-the-Art. Front. Phys. 9:624963. doi: 10.3389/fphy.2021.624963
Received: 01 November 2020; Accepted: 29 January 2021;
Published: 08 April 2021.
Edited by:
Marco Durante, GSI Helmholtz Center for Heavy Ion Research, GermanyReviewed by:
Elke Beyreuther, Helmholtz-Gemeinschaft Deutscher Forschungszentren, GermanyLorenzo Manti, University of Naples Federico II, Italy
Copyright © 2021 Chaudhary, Milluzzo, Ahmed, Odlozilik, McMurray, Prise and Borghesi. This is an open-access article distributed under the terms of the Creative Commons Attribution License (CC BY). The use, distribution or reproduction in other forums is permitted, provided the original author(s) and the copyright owner(s) are credited and that the original publication in this journal is cited, in accordance with accepted academic practice. No use, distribution or reproduction is permitted which does not comply with these terms.
*Correspondence: Pankaj Chaudhary, cC5jaGF1ZGhhcnlAcXViLmFjLnVr; Kevin M. Prise, ay5wcmlzZUBxdWIuYWMudWs=; Marco Borghesi, TS5ib3JnaGVzaUBxdWIuYWMudWs=