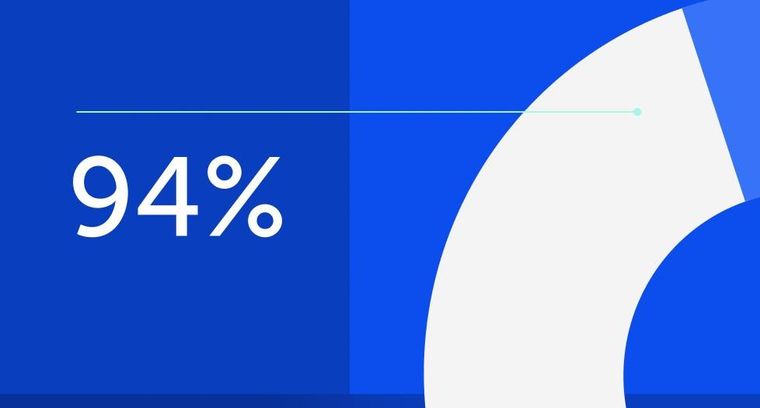
94% of researchers rate our articles as excellent or good
Learn more about the work of our research integrity team to safeguard the quality of each article we publish.
Find out more
REVIEW article
Front. Phys., 14 April 2021
Sec. Low-Temperature Plasma Physics
Volume 9 - 2021 | https://doi.org/10.3389/fphy.2021.617345
This article is part of the Research TopicLow-Temperature Plasma for Biomedical ApplicationsView all 10 articles
Plasma treatments are currently being assessed as a seed processing technology for agricultural purposes where seeds are typically subjected to pre-sowing treatments to improve the likelihood of timely and uniform germination. The aim of this review is to summarize the hypotheses and present the evidence to date of how plasma treatments affect seeds, considering that there is difficulty in standardizing the methodology in this interdisciplinary field given the plethora of variables in the experimental setup of the plasma device and handling of biological samples. The ever increasing interest for plasma agriculture drives the need for a review dedicated to seeds, which is understandable to an interdisciplinary audience of biologists and plasma physicists. Seeds are the first step of the agricultural cycle and at this stage, the plant can be given the highest probability of establishment, despite environmental conditions, to exploit the genetic potential of the seed. Furthermore, seedlings seem to be too sensitive to the oxidation of plasma and therefore, seeds seem to be the ideal target. This review intentionally does not include seed disinfection and sterilization due to already existing reviews. Instead, a summary of the mechanisms of how plasma may be affecting the seed and its germination and developmental properties will be provided and discussed.
The motivation driving plasma-seed treatment research is the importance of food. The world population is projected to increase to 10 billion by 2050 and even without increasing the food supply, it is necessary to maintain the current food production and quality [1]. Most food begins with planting seeds.
The aim of this review is to centralize the hypotheses and present the evidence to date of how plasma treatments affect seeds, considering that there is difficulty in standardizing the methodology in this interdisciplinary field given the plethora of variables in the experimental setup of the plasma device and handling of biological samples. Considering that plasma agriculture has been gaining more attention recently, it is therefore useful to have a review dedicated to seeds, which is understandable to an interdisciplinary audience of biologists and plasma physicists.
The review is organized as follows. The Seed Structure and Development section will be presented first to establish a common ground, followed by a summary of current techniques used to improve seed survival in section Seed Performance. In section Non-thermal Plasma (NTP) as a Seed Processing Technology, plasma will be introduced and the motivation for its application on seeds followed by a corroboration of recent research to provide an overview of physical, chemical, and biological mechanisms that can be triggered by plasma components is in section Mechanisms of How Non-thermal Plasma Affects Seeds and Their Subsequent Development.
Although very diverse, all seeds have generally evolved to contain all their needs to develop into plantlets once the environmental conditions are perceived as appropriate. The living tissues of seeds are protected by the seed coat (testa), which can vary between species and cultivars, or depend on the plants being fertile or clones unable to produce the next generation. Inside the dry mature seed, the embryo is in a partially desiccated, quiescent state, poised to germinate upon the addition of water or in other words, imbibition. It is then provided with stored foods through the endosperm during germination, a process which is the transition from an inactive to active seed that grows, ruptures the seed coat and develops from a seedling first into a plantlet, which is generally still frail and particularly sensitive to external stresses, to then a more stress-resistant autotrophic plant.
The trigger for germination requires a number of external parameters to be met, which will vary from seed to seed, but are generally a combination of water, temperature and light. Additionally, each seed has different requirements due to structural differences, particularly in the seed coat. Imbibition is the first step where the seed undergoes three stages with initially rapid, then slow and finally rapid water uptake. Water reactivates the enzymes that can repair DNA and membrane damages by using pre-existing RNA transcripts produced during seed maturation, and activates enzymes involved in beta-oxidation and amylases to break down stored oil and starch into sugars for energy and cell wall production. Proteases break down storage proteins into amino acids for protein synthesis [2].
After the third water uptake stage, the seed is swollen since the tissues have expanded and the embryo grows. The first visible sign of germination is the protrusion of the radicle, which later becomes the root. From there, the hypocotyl, which connects the root and shoot, hooks out and brings out the shoot with the cotyledon/s, then the true leaves. Once photosynthesis starts, the plantlet can grow independently from prior storages of organic matter and will only take nutrients and water from the soil and the surrounding media.
Prior to germination, there are hormones and inhibitors that prevent the process, to ensure the right environment and maximize the probability of the germinated seed to survive and thrive. The hormone abscisic acid (ABA) is known for its role in maintaining dormancy and for inhibiting germination. When this is removed by a lengthy water imbibition, another hormone gibberellic acid (GA) is produced and germination begins. As the embryo grows, both auxin and cytokinin are involved in cell expansion and cell division, respectively, whereas later during the stressful life of the plant, hormones such as salicylic acid, jasmonic acid and ethylene will play a role in plant defense to protect it against abiotic stresses such as cold, heat, dehydration, and biotic stresses, such as herbivores, viral, fungal and bacterial pathogens. More information about plant defense can be found in Andersen et al. [3].
The best chance for survival is to provide the seed with an opportunity to germinate from the very beginning, assuming they are not dead, and for food production, there are additional criteria beyond successful germination which are germination uniformity and rate for a single harvest when done on an industrial scale. For this reason, many centuries ago, a method called priming was developed to ensure a more uniform and faster germination.
Seed priming is a method which can increase plant growth parameters, such as germination rate and uniformity and contribute to higher yields and greater plant resistance. As reviewed by Pawar and Laware [4] and Lutts et al. [5], the concept of priming is to provide water and activate the metabolism of the seed to repair damage before continuing the embryo development and the emergence of the root.
Priming is frequently applied using water (hydro-priming), which requires soaking the seeds for a given timeframe. This water treatment can be modified to ameliorate germination rate, efficiency and uniformity with the addition of salts (halo-priming), solutes to change the osmotic pressure (osmo-priming), micronutrients like boron and iron (nutri-priming), hormones like gibberellic acid (hormonal priming) or beneficial microorganisms, such as Pseudomonas species (bio-priming), and metallic nanoparticles like iron and silver (nano-priming). Priming can also be done without water by using a solid and non-soluble material such as sand or clay called matrix priming [4].
Depending on the seed and its structure, it can be primed with wet treatments by soaking in cold, warm, boiling water or dry treatments using dry heat or microwaves. Seeds can also be primed using acid scarification and physical scarification. Seeds, specifically their seed coats, can also be modified with compounds such as selenium or salicylic acid [6] or agents that are protectants, nutrients, symbionts, soil adjuvants (hydrogels) and colorants [7]. Protectants, such as pesticides, and colorants make up the bulk of coatings and are applied mostly to crops and vegetables to mainly deter insects (44%), weeds, and fungi [8].
Pesticides in the seed coatings can transfer into the soil through rain and enter the groundwater and wastewater treatment plants. Since these compounds are highly toxic, persist for a long time and become more toxic with time when held in storage, remediation methods, used to remove toxic chemical compounds, can be done with microorganisms, clay, polymeric materials or UV-H2O2 and UV- ozone, and hydroxyl radicals and at times, even require additional water resources [9].
Although protective coatings are important and occupy a multi-billion-dollar market, the amount of investment in remediation techniques highlights that it would be beneficial to consider alternatives to minimize pesticide use. For this reason, alternatives have been considered such as biocontrol using fungi or bacteria, biopesticides derived from natural compounds such as grapefruit seed extract [10], physical methods such as ultrasound [11, 12] as well as genetic engineering [13]. It would also be ideal to find a method with minimal energy consumption in view of energy savings considering that energy input is five to ten times greater than the output in the form of food in North America [14].
Ideally, more effective solutions for seed treatment to ensure rapid and uniform germination should not include toxic residues, consume little energy, have low penetration depth to avoid injuring cells, and favor long storage time [15], while subsequently supporting optimal seed development. These criteria can possibly be met with non-thermal plasma (NTP) treatments.
Plasma is an ionized gas that can be ignited under low or atmosphere pressure conditions. The plasma composition depends on the operating parameters such as voltage, frequency, humidity, flow rate, and gas mixture. Gases such as argon, oxygen, nitrogen, helium, and/or air can be ionized by electric fields to form electrons, ions, UV, thermal radiation and reactive species. Specifically, air plasmas contain reactive oxygen species (ROS) such as superoxide (), hydrogen peroxide (H2O2), hydroxyl radical (OH·), singlet oxygen (1O2), and ozone (O3) and reactive nitrogen species (RNS) such as nitric oxide (NO·), peroxynitrite and nitrogen dioxide radical () [16]. Han et al. [17] and Laroussi [18] describe the types of plasma treatments in detail. Šimončicová et al. [19] describe the types of plasma treatments for biological applications. In short, they include dielectric barrier discharge (DBD) as the most common plasma source, followed by plasma jet, corona, microwave, radiofrequency, and gliding arc. The DBD generates plasma by alternating high voltage (kV) between two electrodes, one or both of which are insulated by a dielectric to prevent electric arcs. The DBD may generate the plasma in a volume of gas between the electrodes (VDBD) or on the surface of a dielectric adjacent to electrodes (SDBD). In a plasma jet, there is usually a DBD excitation of gas flowing in a thin tube. Corona ignites plasma at the tip of a sharp edge and forms diffuse plasma toward the ground electrode. Microwave plasma is produced using a magnetron at a very high frequency in the GHz range, whereas radiofrequency plasma is ignited in the MHz range; both are ignited in a vacuum chamber at low gas pressure. Gliding arc generates an arc at the shortest distance between two diverging electrodes, which then expands. The seeds and seedlings can be treated directly with the plasma or indirectly at a distance away from the plasma. They can also be soaked or watered using liquids exposed to plasma. These liquids are considered as plasma-activated media (PAM) and if water is used, it is called plasma-activated water (PAW). Comparing the gaseous and aqueous treatments, similar effects on macroscopic plant properties have been reported [20, 21].
Plasma treatment may enhance seed survival without toxic residues since all the constituents in plasmas may be found in nature and recombine shortly afterwards. Moreover, components in plasma have approximately 10 nm deep penetration, limiting it to surface functionalization [12]. Importantly, plasma treatments are considered to be low maintenance with low energy costs [22]. Therefore, many studies have been performed involving plasma treatments of agronomic interest, such as quinoa, basil, tomato, wheat, radish, soybean, mung bean, rice, Ajwain and Umbu and seeds deemed important for the landscape like Norway spruce [23].
Randeniya and de Groot [22] and Puac et al. [24] reviewed the observed effects on germination and subsequent plant growth. The extensive list of these effects in this review has been summarized in Figure 1. It can increase germination probability and biomass [25] or increase disease resistance or stress resistance [26, 27]. Additionally, it can accelerate or delay germination and subsequent development [28], decrease water consumption [29], decrease levels of microbial pathogens or insects [30, 31], without detectable toxic residues [20]. Molina et al. [32] proposed to make a hydro-absorbant polymer coating with short plasma treatments on specific seed types, and Kopacki et al. [33] suggested to use plasma for seed coatings against fungal pathogens.
Figure 1. Effects of plasma treatment on seeds which includes surface modifications, changing growth parameters, modulating disease, and stress resistance through metabolism.
Other than treating the seed or plant directly, plasma treatment can also be used on the plant's surroundings by degrading volatile organic compounds in the soil to improve soil health [34, 35]. It may also have potential as an alternative to fungicide [36] and be implemented in industry as field studies have shown [16, 37].
Table 1 shows the legend describing the categories of Table 2, which is a compilation of papers described in four categories: seed coat modification, growth parameters, metabolism, and disease or stress resistance and further divided by the scale of information i.e., macroscopic, microscopic or molecular properties. Seed disinfection and sterilization were intentionally left aside and can instead be found in more detail in the food processing field [108].
Table 1. Legend describing each category of Table 2 for the type of plasma and seed used in the study as well as the main findings (see this table for a description of the categories).
Quite consistently, most reports refer to optimized plasma setups that can significantly change germination and plant growth parameters. For example, germination rate may be accelerated, shoot and root lengths may be longer [68] and more branching of the roots [25] or stronger root system [85] were reported although it is difficult to know the causative agent behind these effects due to varying plasma-seed treatment methodologies.
Not all observe the same effects and instead, plant growth can be improved without changing germination rate [49] or scientists have strong variation in their experiments [53, 69]. This could mean that further optimization is required, which is time-consuming considering the number of variables in the experimental design. It is not yet clear by which mechanism(s) these effects arise but understanding this will simplify the experimental design and potential scale up for the future so that the results are reproducible. Here, we take a closer look at the physical, chemical, and biochemical factors derived from plasma treatments to have a more detailed understanding of what is happening to the seed. A summary of the plasma-seed interactions at the seed surface and at a molecular level are given in Figures 2, 3, respectively.
Figure 2. Summary of the possible mechanical, chemical, and biochemical interactions of plasma components with the seed surface.
Figure 3. Summary of hypotheses and current evidence of plasma-seed treatments on a molecular scale.
The physical factors such as heat, ultraviolet, and electromagnetic fields and mechanical scarification are the first contact point with the seed coat and may then trigger downstream consequences from this initial interaction. In the following section, each physical factor and its effect will be presented individually.
The temperature of plasma treatments can range from room temperature up to 90°C, so in principle it is possible to have an effect depending on the temperature and treatment time although not many authors think that increased temperature is responsible for the changes in plants. Temperature is monitored in a few studies by measuring the electrode temperature, calculating the gas temperature from spectra or measuring the temperature of the seed directly using an infrared camera or thermocouple [47, 109] but it has not yet been done on a molecular level by assessing the seed or plant response. Kitazaki et al. [110] compared plasma and heat treatments by heating the seeds on a hot plate but did not see the same effect on the plant development.
Others have tried to look into heat shock proteins (HSPs), which are induced with temperatures ranging from 31 to 37°C, but HSPs are often not exclusively induced by heat. They may also accumulate under oxidative stress, high intensity irradiation, and desiccation [111]. Iranbakhsh et al. [78] treated wheat seedlings with plasma and observed increased expression in heat shock factor A4A in both the root and shoot system in correlation with an increase of growth parameters and mitigating the negative effect of salinity stress. They only reached a maximum of 29°C in a 2 min plasma treatment, suggesting that heat alone is not responsible for the observed effects in this particular study but the role of HSPs during plasma treatment remains unclear since it was shown not to change in the study by Mildažiene et al. [53].
The role of high-energy photons in plasma-seed treatments has been controversial since UV has had a negligible effect so far but the effects of UV on seeds and plants could theoretically contribute to the wettability or growth enhancement effects indirectly; for example, by producing radicals or reactive oxygen species (ROS) such as ozone [60]. Gao et al. [52] checked the effects of UV separately from plasma and observed that UV had only a minor contribution toward the seed wettability. Sarinont et al. [102] also reported that there was no effect from UV when they saw the lack of growth enhancement effect after they blocked UV with a quartz plate.
It is known that just UV-B can accelerate germination of safflower seeds but then negatively affects growth [112]. Noble [113] made the same conclusions with kale, cabbage, radish and agave seeds. Likewise, Sadeghianfar et al. [114] showed accelerated germination of maize and sugar with UV-C treatment but instead, saw an increase in plant growth parameters and suspected this may be due to breaking down the seed coat and increase in temperature.
There may be differences in effects depending on the wavelength since it has been shown that UV-A had a more pronounced effect than UV-C but both were able to accelerate the germination rate and improve growth parameters [115]. On the one hand, UV is often associated with inducing DNA damage. Prakrajang et al. [46] compared gamma radiation with plasma and saw only with gamma irradiation that the plants did not grow well and thus suspected it was induced DNA damage. On the other hand, plants treated with UV were able to better cope with drought stress, possibly by activating DNA repair mechanisms [116]. This may be due to an increase in phenolic compounds, which often have a role in disease or stress resistance and this response can be triggered by intense UV light which is accompanied by high temperature and photo-oxidative damage in nature [117].
Babajani et al. [59] and Iranbakhsh et al. [118] both considered that plasma-derived UV may be detected by photoreceptors which then might affect secondary metabolism and trigger stress responses if the treatment is done briefly. UV is also linked with photomorphogenesis and cell elongation, division, and differentiation [119]. Iqbal et al. [63] compared laser and plasma treatment separately on seeds and saw similar types of effects: damage to the seed coat, an increase in water uptake and protein content.
Pauzaite et al. [23] and Mildažiene et al. [53] also attributed the changes in growth parameters to radiation since Mildažiene et al. [53] saw similar protein expression profiles when comparing seeds treated with plasma or electromagnetic fields (EMF). This is not entirely surprising since pulsed electric fields also affect seed germination [120, 121]. It needs to be kept in mind though that electric field treatment is also accompanied by oxidative stress and ozone [122] where ozone has been suggested by Patwardhan and Gandhare [123] to be the main effective parameter in the treatment.
Static or alternating magnetic fields can also change germination probability, growth rates, increase root, and shoot length, change redox status of plants possibly by increasing hydrogen peroxide (H2O2), altering photosynthesis, alleviating drought stress or increasing mineral content [124, 125]. Arguably, UV may have a role but a minor contribution in terms of direct effect considering that the above studies used several hours long UV treatments to have an effect whereas most plasma treatment are in the seconds or minutes range. Nevertheless, photons and electromagnetic fields can still possibly contribute indirectly through the production of RONS.
In terms of mechanical effects, it has been suggested that altering the seed coat may play a role in modifying germination rate. Typically, a seed has four layers: the cuticle, epidermis, hypodermis, and parenchyma. The differences in the microstructures and chemistry of these layers determine differences between species and even cultivars. The seed coat role is like a water modulator; it controls the entry of water so it can be absorbed slowly by the cotelydons to minimize or avoid imbibition damage [126].
It has been suggested that the removal of the lipid layer allows for better access to water, a requirement for triggering germination [127]. Bafoil et al. [61] showed the importance of the seed coat by using mutants of Arabidopsis plant model Ler and Col-0 ecotypes and showed the rearrangement of lipid components, changes in lignin and seed coat erosion. Many, although not all authors have observed with scanning electron microscopy (SEM) that seed surfaces treated with plasma have an eroded appearance [52, 83, 86, 93, 96, 104], where Mildažiene et al. [128] only saw etching on the seed surface facing plasma treatment and others did not see any changes [29, 110, 129].
A majority of these authors have been able to correlate these changes with increased water uptake. For example, Pawlat et al. [72] observed that plasma treatment changed the seed structure by removing the upper cuticle layers covered with wax, a polymer present to prevent water loss under heat stress, and may form micro-pores to aid water absorption. Bafoil et al. [61] also worked with an Arabidopsis mutant gtap5, which is not able to make suberin or cutin and saw that plasma was not able to improve the germination without these polymers in the seed coat, suggesting their importance. This waxy layer was even considered as a parameter in the experimental design of a study done by Park et al. [80]. They used two seed types, one with and the other without a waxy layer, and their results indirectly implied that this waxy layer plays a role in the effect that plasma treatment will have on the seeds, suggesting plasma indeed may be improving the seed coat permeability, considering wax limits water loss and controls gas exchange.
Billah et al. [40] pointed out that there may be exothermic reactions from the plasma that release heat and may melt the wax due to its low evaporation temperature of 37°C or eroded by reactive species. This was also pointed out by Holc et al. [130]. Wang et al. [83] also suggested that through surface modification via etching, the seed is able to absorb water through increased hydrophilicity. This enhanced water absorption by modifying the seed structure is further supported by others who observed the degradation of cellulose on seed surfaces [131]. Currently, the analysis of components with FTIR-ATR is limited to cellulose since it is difficult to differentiate between different organic plant components like cellulose, hemicellulose, pectins, etc. Wang et al. [83] used both FTIR-ATR to measure seed surface changes as well as FTIR to analyze the gas exhaust from the plasma treatment. They needed to omit wavenumbers i.e., peaks below 1,500 cm−1 that were difficult to assign to specific functional groups during analysis but also suggested that the spectral bands are mainly attributed to cellulose rather than wax.
Junior et al. [96] showed that water may be guided differently after plasma treatment due to surface modifications. They observed that the hilum increased the amount of water absorption, the micropyle had a more open configuration and in particular, the water absorption was improved mainly through the hilum rather than micropyle. In any case, by thinning the seed coat, it is logical to assume that water will be more readily absorbed.
Interestingly, the water absorption can be controlled by the plasma treatment using different gases and coating thickness as mentioned by Volin et al. [28]. Depending on the working gas, 0.5–2 μm thick coatings were applied and their thickness modified imbibition. This suggests that the seed coat thickness can be mechanically modified by etching or by changing the chemical properties.
Despite adding to the thickness with an additional layer of coating, germination was improved in a few instances, which highlights the importance of chemistry, but it is difficult to separate the etching effect from the chemistry in this study. As it still remains, it is not known yet whether this increased permeability is principally due to mechanical mechanisms such as etching as Pawlat et al. [132] suggested, a combination of both mechanical and chemical as mentioned by Gómez-Ramírez et al. [90], Tounekti et al. [70], and Park et al. [80] or solely due to chemistry.
SEM is an insightful tool, which can provide quick qualitative results of the plasma-seed treatment. Nevertheless, caution should be exercised when interpreting SEM images because plant genetics influence the seed coat pattern. Otherwise, observed changes might already be pre-existing and should instead be attributed to biological variation rather than plasma treatment and therefore, using the same seed before and after treatment is recommended.
Pawlat et al. [132] noticed that the seed shape influences the type of change affecting the seed and showed in their SEM images that the seed edge was torn off whereas the middle grew in sharpness. This brings attention to the fact that this process needs to be delicately handled. For example, Cui et al. [55] used tape to prevent the movement of seeds during the plasma treatment but this may affect the seed coat especially if it is done in the presence of moisture. As a result, it seems that there is a limit to how much information can be extracted from surface analysis using microscopy. Instead, it would be useful to further explore the chemical modifications since this is currently limited at the moment in the literature.
It may very well be that the chemistry is sufficient to affect downstream processes considering that an effect in growth parameters without visible seed coat modifications has been observed [57]. Mildažiene et al. [85] also observed positive growth effects using vacuum and EMF without visibly changing the seed coat structure using SEM. It may also be the case that the results will depend partly on whether there is physical or physiological dormancy meaning whether dormancy is due to the seed coat or embryo.
On the one hand, etching may play more of a role in certain seed types with impermeable seed coats but, on the other hand, it may be that a specific concentration and/or mixture of reactive species from a higher power plasma is required to observe an effect. In the latter, mechanical damage might merely be a side effect, which too can contribute to enhanced water absorption but is not the primary factor. For this reason, chemical factors will now be discussed in the next section.
In addition to analyzing mechanical damage, SEM can be coupled with energy dispersive X-rays (EDX) to look at the composition of seed surface. Other methods such as X-ray Photoelectron Spectroscopy (XPS) and micro X-ray fluorescence spectroscopy (μ-XRF) can also be used to acquire information about the elemental distribution. It has been observed by several authors like Pérez-Pizá et al. [36] and da Silva et al. [93] that lipid layers undergo chemical oxidation, which can therefore improve interaction with water.
Shapira et al. [82] found that irreversible wettability is not due to electric charging, which could indirectly imply that it may be done chemically. Pérez-Pizá et al. [36] also correlated the increased hydrophilicity with oxidized seed using nitrogen and oxygen plasmas. This perhaps suggests that hydrophilicity again is done through chemical rather than mechanical changes. This may not be entirely dependent on the gas type but rather about producing an appropriate profile of reactive species at sufficient concentrations that will oxidize the outermost lipids. It has been shown by Gómez-Ramírez et al. [90] using XPS and EDX that plasma treatment can oxygenate carbon and deposit nitrogen groups on the seed surface. They hypothesized that these elements, as well potassium, are later absorbed by the seed in the presence of water [133].
Ambrico et al. [50] instead used μ-XRF and showed the concentration and redistribution of macro- and micronutrients such as potassium after plasma treatment. As an example, on the one hand, the diffusion of potassium into the seed interior may improve the germination through enzyme activation, help with water retention, or detoxify ROS [134]. On the other hand, potassium at the surface can be interpreted as seed damage since it may be used as an indicator of cell membrane integrity [135]. Interestingly, Zhou et al. [98] exposed seeds to plasma-treated water and they had the lowest leakage rate by measuring electrical conductivities, which is used to measure the cell membrane integrity. This mobilization of nutrients perhaps is not lost to the environment but is loosened and absorbed immediately by the seed [136].
When considering gases, it could be that oxygen on the surface is responsible for not only increased wettability but may also assist in seed respiration. Considering that oxygen is another requirement for germination, Sarinont et al. [102] observed oxygen, NO and nitrogen gas to be the most effective for plant growth but fumigation with oxygen gas already had an effect on growth parameters, albeit mild compared to plasma. This fumigation might be sufficient to improve plant growth like in the case of nanobubbles [137]. Although fumigation had a positive effect on plant growth, plasma application had a stronger effect which may be due to the transport process being more efficient i.e., speed up the process by not relying on diffusion or provide more directionality. This highlights the presumably overlooked importance of oxygen especially considering that Rahman et al. [73] saw also more obvious changes in growth parameters when using an oxygen admixture instead of air.
This principle could likewise work for nitrogen gas but instead of modifying respiration, it may be directed toward other cellular processes such as photosynthesis since it is a major component of chlorophyll or protein synthesis [138]. As mentioned in the paper of Gao et al. [52], the presence of CO and signals confirmed that chemical etching of the seed surface by plasma played an important role in stimulation of seed germination [139]. These chemical changes may then be responsible for changing the biochemistry and molecular events in the plant and therefore, these will be discussed in the next section.
Regardless of whether the changes to the seed coat surface might loosen an elicitor such as oligosaccharins, as mentioned by Iranbakhsh et al. [78], many authors are in agreement and speculate that it is principally reactive oxygen and nitrogen species (RONS) that trigger biological processes.
Oxidation of the seed coat is very often observed but this can be propagated internally since there can be an increase in maldonaldehyde (MDA), a product of lipid peroxidation, after plasma treatment as seen by both Los et al. [57] and Cui et al. [55]. This may be among the first steps in the signal transduction considering that lipid peroxidation does not rely on enzymatic activity, which is very limited in dry seeds [140].
Mujahid et al. [44] mentioned that it may be instead the hydroxyl radicals which are responsible for the cell wall loosening. Bafoil et al. [61] calculated an increased expression of 23 genes for class III peroxidases after plasma treatment, which are proteins localized in the seed coat which regulate concentrations of hydrogen peroxide and precede the rupture for germination. Pauzaite et al. [23] also suggested that ROS may alter the seed coat pigmentation, which is known to be linked to germination. The flavonoid biosynthetic pathways and abscisic acid, a hormone for dormancy, are regulated by the same gene locus. Although they had changes in the seed coat flavonoids, they could not find any clear connection in their study between flavonoids and seed germination parameters.
Others have seen that the seed coat pigmentation does in fact influence seed permeability and the rate of imbibition where brown seeds had faster water uptake, reached a germination optimum sooner but were more susceptible to imbibition damage than black seeds [141]. Liu et al. [56] also attempted to decipher between seed types using PAW and brought up in their discussion that seed storage proteins are typically oxidized during germination, which may facilitate the mobilization of the storage reserve. Therefore, it may be possible that plasma-derived ROS may be affecting the seed pigmentation first and then downstream the germination rate. Additionally, considering that Mueller et al. [142] suggested that ROS play a role in the cleavage of cell wall polymers, it may be that changes inside the seed in turn modify the outer layers. The mechanical pressure on the endosperm needs to be relieved for the radical protrusion but this is based on cell wall loosening, which is linked to the action of ROS [140] and this, again, may be another argument that external seed coat erosion might not be necessary for modifying germination.
Alternatively, it could also be that chemical modifications to the seed coat, for example through lipid oxidation, may lead to the carbonylation of proteins, rendering them more susceptible to cleavage and leading to the breakdown of aleurone layer [140, 143]. Therefore, post-translational modifications, such as carbonylation and sulfhydryl group's oxidation, are also another mechanism by which ROS, specifically H2O2, can shift a seed from a dormant to non-dormant state as shown by Oracz et al. [144] and Valderrama et al. [145].
Generally, it is not fully understood how external ROS are detected and how these signals are transduced in seed cells but this process may partly depend on the presence of water. Water is needed to trigger germination and increase respiration, a reaction which oxidizes sugars to release energy in the form of ATP. During respiration, ROS are produced as by-products and thus, ROS generation is the hallmark from dormant to metabolically active seeds [140]. Where the moisture content is low, there is very little, if any, enzymatic activity but there may be hydrated pockets within the seed, permitting limited metabolic activity. It could be that this pocket of water may assist the diffusion and retention of ROS in the seed and, therefore it may be that plasma-derived ROS accumulate in these pockets and trigger signaling for intracellular programs. This process may be done more efficiently with hydrated instead of desiccated seeds due to the higher water content. Nevertheless, in dry conditions, ROS may have an effect that is simply paused until imbibition [140] and this may be why there are long-term effects [23] or why long-term storage of plasma-treated seeds can still have a positive effect on growth parameters relative to the untreated seeds [102]. On the other hand, the effects of the plasma treatment may be continuously ongoing but are not morphologically obvious until imbibition and subsequent growth. Mildažiene et al. [128] pointed out that biochemical changes continue to occur in seeds at various levels, including hormonal balance, gene expression, oxidative processes, mRNA content, and protein translation during storage and these changes may occur across all of these levels after plasma treatment.
Although it is not known which ROS is/are responsible for the effect and how it/they enter, it is known that seeds have pores whose size is genetically regulated and this also may assist the diffusion of ROS into the seed [126]. H2O2 can interact with the surface and diffuse through the membrane but charged species such as superoxide are not able to bypass the membrane and are dependent on voltage-dependent anion channels called porins, which are only found in the mitochondria [146]. Despite its charge, superoxide can break down into hydroxyl and singlet oxygen and these may be able to bypass more easily [147]. In some cases, RONS bypass the membrane using aquaporins, protein channels used for water transport [148]. Transport also depends on the life stage of the plant since ozone can be taken up through the stomata in the leaves. Seol et al. [149] observed an accumulation of ROS in chloroplasts, and suggested that through the micropores, ROS can travel down further into the plant tissue, from the epidermis into the mesophyll but if too potent, chloroplast degradation occurs.
Considering the lifetime and complexity of the reactive species reactions, there is a bias for researchers to measure longer-lived species like H2O2, O3, and NO and therefore, most of the focus for this section will be dedicated to these species herein [150].
Surprisingly, little has been done to monitor and measure ozone during plasma treatments and it seems that many overlook the effect of ozone on seed germination despite it being possible to enhance germination with optimized treatment parameters. On the one hand, ozone can impair plant growth by replacing CO2 and reducing photosynthesis. This is done by inhibiting the opening of the stomata due to the reduced flow of potassium ions [151]. In some cases, plants like mung beans are not able to overcome ozone stress with their antioxidant defenses [152]. On the other hand, ozone has been used to enhance seed germination [153] or improve fruit quality [154]. Ozone seemingly has an ambivalent role that is dependent on the concentration and length of treatment [155] as it is the case for many other treatments i.e., hormone, nanoparticles, heat. The authors mentioned that there is a variable response to the same ozone treatment based on the species and it is weak and transient. By using electron paramagnetic resonance (EPR), they showed that O3 increased the concentration of radicals (carbon and oxygen species) in all tested species except one.
Pawlat et al. [72] measured 0.01 ppm of ozone produced during plasma treatment and had an effect on the growth parameters but it is not clear whether this is due to ozone or other treatment parameters, such as the short heat treatment at 40°C. Perhaps this ozone treatment might not have been sufficient, in terms of concentration and exposure time but is a good example to point out that it would be helpful to include as a control in studies to clarify whether the effect is due to ozone, other reactive species or other parameters.
It is especially interesting to differentiate this because ozone can trigger the production of ethylene, which then breaks down abscisic acid, the seed dormancy hormone [156]. Alternatively, it could be that ozone generates short-lived species inside the seed, which may be recognized as a signal for germination since the accumulation of ROS and peroxidation products is linked with seed dormancy alleviation [144]. As Sudhakar et al. [157] pointed out, the production of hydrogen peroxide is observed in the early imbibition period of tomato seeds and nitric oxide, hydroxyl radicals and superoxide radicals accumulate during seed germination in different species. For this reason, many scientists speculate that H2O2 and NO are the reason for the observed effects using plasma treatments, whether or not it is the short-lived species such as superoxide, hydroxyl and NO that are eventually converted into H2O2 [55].
Wang et al. [83] used a nitrogen plasma in open air and suggested that nitrogen oxides, which are known to have a role in dormancy and germination signaling, are present after plasma treatment and may initiate these biological processes [158]. Instead, Puac et al. [159] looked at meristematic cells of carrots treated with a RF plasma for <2 min and suggested that at least H2O2 and superoxide can pass through the cell membrane and reactive species may be one mechanism since they observed fluctuations in levels of redox quenching antioxidant enzymes such superoxide dismutase (SOD), catalase (CAT), ascorbate peroxidase (APX), which control and limit damage from excessive levels of ROS. This was also observed by Henselova et al. [160] in maize using a DSCBD for <2 min. Simultaneously, these antioxidative enzymes may behave like sensors to detect ROS availability and redox perturbations so that an organism can respond appropriately [145, 161]. There is also an overlapping relationship between these enzymes, NO and H2O2 where NO positively regulates APX1 through a post-translation modification, S-nitrosylation, which then enhances resistance to oxidative stress and improves immune responses [162] and NO regulates itself as well as ROS [145, 163].
Rahman et al. [73] suggested that plasma induces H2O2 formation, which is found in hydrated seeds and is involved in imbibition and early germination. They compared Ar/O2 to Ar/air admixtures and observed higher concentrations of H2O2 with Ar/O2 without inducing scavengers that counter H2O2 production. They concluded that plants treated with this admixture had better growth parameters due to the increased H2O2 concentration and did not see any changes in NO. Likewise, after plasma treatment, an increase in H2O2 concentration was correlated with positive effects on germination, whereas a decrease was correlated with negative effects on germination [23]. The same authors also showed for the first time the oscillatory dynamics of H2O2, which occurs in Arabidopsis and conifers but it is not characteristic for other seed types such as radishes and sunflowers. This already suggests that there can be differences between species including on a molecular level.
Kang et al. [39] interestingly did not see an effect and observed high variation in rice germination. Los et al. [57] also saw no changes in H2O2 levels in wheat but still had growth enhancement. This suggests that there are either differences in the plasma that do not necessarily lead to changes in H2O2 and requires optimization, or that seeds respond differently, and it is not exclusively due to H2O2 but instead to nitrites and nitrates.
This latter point was heavily emphasized by Billah et al. [40] who observed an increase in H2O2 but think that nitrogen is the main contributor for enhanced growth in gram seeds. In contrast, Liu et al. [56] compared different gases using direct and indirect plasma treatments to produce PAW and soaked a variety of seeds. They were tempted to believe that effects are more likely due to oxygen-derived species. Interestingly, NO can downregulate the signal for H2O2 and will activate genes for antioxidant enzymes as pointed out by Iranbakhsh et al. [118] so once again, it is difficult to separate the effects of both H2O2 and NO.
In the case of H2O2, it is considered a long-distance signaling molecule, which is highly interconnected with hormones, metabolism and gene transcription. The signaling likely includes MAPK cascades as pointed out also by Babajani et al. [59]. ROS mediated signaling includes calcium signaling, protein phosphorylation and gene transcription which are redox sensitive. The relationship between ROS and MAPK is not fully elucidated and both are able to regulate each other. These complexes phosphorylate transcription factors, kinases, phosphatases or other proteins, which can then change enzyme activity or gene expression [164].
Additionally, ROS may produce changes in calcium signaling and use different signatures in terms of duration and amplitude depending on the species and this will dictate what happens downstream, for example root elongation [165]. It was shown by Cui et al. [55] that there was increased calcium in the roots of 4-day-old Arabidopsis seedlings which were previously plasma-treated as seeds and they mentioned this may eventually lead to plant growth if maintained at a low level. Since there is little evidence about signaling due to plasma treatments, the focus now will shift from signal transduction to how hormones, metabolism and gene expression are modified with plasma, starting first with hormones.
It is difficult to differentiate between the action of ROS and hormones because ROS are highly interconnected with hormones like salicylic acid (SA) and jasmonic acid (JA), which are widely reported. Information remains scarce with auxin and cytokinins, which are hormones that affect germination properties [150]. Nevertheless, “hormones modulate the effects of ABA/GA balance: auxin IAA (indole-3-acetic acid) is a negative regulator of germination; ethylene, cytokinins, brasinosteroids, and strigolactones can stimulate germination; SA and jasmonate (stress hormones) may affect germination positively or negatively depending on the situation” [53].
There are authors like Kitazaki et al. [110], who agree on the importance of reactive species. Specifically, they think it is the relationship between ROS and the hormones, which stimulates plant growth. It is true that the importance of hormones cannot be overlooked considering that the seed coat is a source of hormones for the developing seed [166, 167]. Mildažiene et al. [85] also demonstrated how a dry seed undergoes subtle metabolic modulation by using proteomics. They showed that vacuum affected the auxin/cytokine balance, cold plasma increased GA, and EMF decreased the amount of ABA and increased IAA and SA without changing GA despite not being able to make a clear connection between phytohormones and germination kinetics. Ji et al. [168, 169] also observed an increase in GA in spinach seeds but did not measure any other hormones. The increase in GA may be the result of plasma-derived ozone [157] although it is often speculated that it is specifically auxin and cytokinins that are affected, which are hormones that increase and stimulate cell division, proliferation and elongation. Perez Pizá et al. [170] showed that with plasma treatment, there was a decrease in ABA and increase in IAA, a hormone which increases growth by regulating enzyme activity. They also detected H2O2, which coincided with an increase in ethylene after 24 h of imbibition.
It may be that plasma-derived ROS regulate hormone production while for example increased auxin levels may be the reason for increased lateral root growth as pointed out by Wang et al. [171] where the authors correlated increased levels of auxin in tomato with lateral root growth under drought stress. Stolárik et al. [104] found changes in auxin and cytokinins with a 2-min plasma treatment in peas. They found an increase in IAA and oxIAA as well as zeatin, the most common cytokinin, and correlated this with increased growth parameters. This is logical since these two hormones work together; auxin is responsible for DNA replication and initiating the cell cycle whereas cytokinins trigger cell division and mitosis [172].
It seems that plasmas may somehow modulate concentrations of hormones and there may be a link with auxin especially considering that this hormone also affects xylem differentiation [173]. It has been observed that plasma treatment modified the diameters of root and stems as well as the differentiation of tissues such as xylem and phloem [51, 52, 58, 174]. These changes in root morphology may then enhance nutrient exchange [68] which was also proposed by Zahoranová et al. [97]. Moreover, Babajani et al. [59] pointed out that auxin transport may also be affected by NO, which is often produced in plasmas. Additionally, it could very well be the case that auxin biosynthesis is upregulated because it is known that abiotic or biotic stresses increase the shikimate pathway which produces the precursor for IAA.
As stated previously, there is a link between hormones and metabolism and both primary and secondary metabolism, which are involved in growth and defense, respectively, are altered after plasma treatment [175].
Regarding primary metabolism, increased ATP levels along with ethanol were observed, suggesting increased anaerobic respiration [92]. Moreover, higher concentrations of protein and sugar were observed by Islam et al. [64] and Billah et al. [40] due to an increase in reserve utilization enzymes. Billah et al. [40] explained that this could be because H2O2 transduces the signal for soluble sugar synthesis and therefore, more soluble sugar and protein are seen after plasma treatment [62]. Moreover, exogenous treatment with H2O2 can stimulate germination by breaking dormancy through the oxidative pentose phosphate pathway, which provides reducing power and carbon for the new growth [176]. This pathway also links primary and secondary metabolism because it provides the precursor for the shikimate pathway, which is important for plant defense.
Regarding secondary metabolism, an increase in enzymatic antioxidants like catalase [58], superoxide dismutase [78], phenylalanine ammonia lyase (PAL) and peroxidases [59] as well as non-enzymatic antioxidants like total soluble sugar and proline to better tolerate stress have been observed, likely due to the increased concentration of ROS. Furthermore, Ghasempour et al. [43] showed an increase in phenols, chlorophyll, flavonoids and alkaloids with plasma treatment. Pauzaite et al. [23] has seen changes in flavonoids after plasma treatment while others have observed increased phenolic compounds [45, 168, 177].
ROS may also trigger defense compounds which can originate from the shikimate pathway and phenylpropanoid pathway to induce the production of precursors. For example, H2O2 can activate the shikimate pathway [178, 179]. ROS can also make the plant readily available in a redox state to respond quickly to stress as stated by Filatova et al. [45]. Iranbakhsh et al. [118] suggests that the defense response, triggered by ROS and/or UV, modulates the hormone balance since they saw an increase in PAL, a key enzyme in the phenylpropanoid pathway. This pathway also produces protective proteins called pathogenesis-related proteins and depending on whether they are acidic or basic, they can be upregulated by salicylic acid and ROS or methyl jasmonate and ethylene, respectively [180].
Although Perez et al. [181] used PAW, they looked at several genes involved in plant defense in an infected tomato plant and saw an increase in the gene expression of pal (phenylalanine ammonia lyase) but not pr1a, pr4, pr5 (pathogenesis related protein) or erf1 (ethylene response factor). The gene pal is involved in the phenylpropanoid pathway and makes defense compounds like phytoalexins and phenolic compounds and therefore, this information complements the increase in phenolic compounds seen experimentally by others previously mentioned in the section Metabolism.
ROS can also affect gene transcription either directly or indirectly through hormone conjugates as mentioned by Stolárik et al. [104]. Redox sensitive transcription factors are widespread among animal, bacteria and plants [147] and these factors can be modified through the formation of disulfides upon sensing ROS [140].
Unsurprisingly, there are others who think plasma also modifies gene expression although molecular information concerning plasma-seed treatments is very limited. Hayashi et al. [182] suggested that ROS generated in plasma with water vapor may be a method to control the redox state of the plant by changing the thiol quantity (oxidizing cysteine to cysteine with OH radicals). This has an important role in gene transcription and therefore can change the plant response.
Iranbakhsh et al. [38] observed an increase in the transcription factor WRKY1 and other enzymes involved in secondary metabolism in plasma-treated hemp seeds. WRKY is of particular interest since it is a family of transcription factors involved in many biotic and abiotic stress responses such as fungus, cold stress, salt stress, and drought tolerance. Furthermore, they are known for regulating phenolic compounds and there are specific factors such as AtWRKY23 that regulate auxin. Moreover, there are also interactions between MAPK cascades and this WRKY family and they can also activate PR proteins [183].
The other genes that have been studied were by Guo et al. [91] who observed an increase in LEA chaperone during stress, Ji et al. [168] who saw increased expression of a hydrolytic enzyme called pullulanase in spinach seeds, Ghasempour et al. [43] who saw an increase in gene expression for DAT, an enzyme in the biosynthesis of vinblastine and vincristine, alkaloids with anti-cancerous properties and Islam et al. [64] who showed changes in gene expression of ascorbate peroxidase and catalase but not superoxide dismutase with an air plasma in rapeseed.
These few preliminary studies demonstrate that plasma treatment can change gene expression for both primary and secondary metabolism, for growth and defense, respectively.
These changes by the plasma treatment in the short-term may be due to epigenetics such as regulating DNA cytosine methylation, which inactivates gene expression [74, 184].
Zhang et al. [92] checked the methylation of genes involved in ATP synthase, an enzyme needed to produce energy for the cell, and TOR kinase, an enzyme which may increase metabolism and biosynthesis for energy and biomass production, and saw decreased methylation, meaning that the expression of these genes increased. Nevertheless, it cannot be ruled out yet that there may be changes to the DNA considering that genotoxic effects from plasma treatment have been observed [185].
Although it was not verified, Mildažiene et al. [85] found many similarities between cold plasma and electromagnetic treatment in protein expression but this may also be done epigenetically or through post-translational modifications. Despite the authors attributing the changes to radiation, it may be the action of hydroxyl radicals or other ROS since they too are produced from high energy radiation [186]. Additionally, there also remains the possibility that instead the DNA repair process is triggered by for example ozone and this may be responsible for the improvement in germination kinetics [187, 188].
Lastly, the plant genome itself may play a role in sensitivity to plasma treatment. Kobayashi et al. [47] treated Arabidopsis seedlings and saw that the ecotypes Col and Ler responded differently to the same plasma treatment although the results were not statistically significant. Lo Porto et al. [67] also pointed out that asparagus germination is strongly influenced by ecotype although they did not include this in their study. Therefore, the genetic component should not be overlooked when considering plasma-seed treatments and studies should be done on multiple generations since plasma treatment can have long-lasting effects in the same generation as observed by Sarinont et al. [102] where the growth enhancing effects remained even after 17 months of storage of plasma-treated radish seeds.
In summary, it remains difficult to infer whether these effects are primarily attributed to the action of a single mechanism like mechanical scarification, chemical modifications, or changes in biochemistry through ROS; even within ROS, each species behaves and functions differently. Additionally, variables such as the state of dehydration or hydration of the seed may influence the interaction and retention of ROS.
Ozone specifically in the presence of UV radiation may undergo reactions to eventually generate superoxide and hydroxyl radicals in the seed coat or these short-lived species may directly interact with the seed coat and this could be the critical point that determines how the external physical and chemical stimuli are transformed into internal biological stimuli.
The seed coat pigments can be altered and seed coat embedded enzymes like superoxide dismutase, NADPH oxidases or peroxidases can transform these species into signaling molecules like H2O2, which can more easily diffuse through the membrane or the apoplastic space, and then transduce this signal either through secondary messengers like calcium and MAPK cascade or be transmitted without the assistance of enzymes. Once the signal is sent, the hormonal balance may be modulated between GA and ABA to break dormancy, to increase auxin and cytokinin to accelerate the germination process, and increase ethylene, SA and JA for stress or disease resistance.
In parallel, metabolism may be modulated through the addition of water and enhanced gas exchange to modify enzyme activity to break down food reserves but at the same time, increase enzymatic and non-enzymatic antioxidants to shield against this sudden burst of ROS either externally derived or from metabolism. As a result of this stressful stimulus, metabolism in other defense pathways like shikimate and phenylpropanoid may be then activated to produce precursors in anticipation of future stressful events such as hormones or protective proteins.
What has been shown to date is a combination of plasma device geometries, treatment methods and seeds that are able to alter the plant parameters. On the one hand, this diversity emerges from individual researchers considering what is relevant for their society and local economy, but on the other hand, this also makes it difficult to standardize the current research. We seem to have reached a point where there is potential in this technology as a proof-of-concept although there may be an inherent bias by publishing solely positive results, giving the impression that finding these setups is simple and it only takes trial-and-error to optimize the treatment conditions. Therefore, it would be helpful for the readers to also publish negative results to know what is not working when designing these treatments to move toward standardization and improve our fundamental understanding.
Most scientists are using DBD plasma enclosed in a chamber with air as the process gas although there is flexibility in choosing the type of plasma device. Air plasma is practical and produces a rich chemistry. However, the role of humidity in these plasma-seed treatments is still debated. Is it best to work with dry or wet seeds? Should one control and add or remove humidity, for example, by a gas flow?
Many authors reported evidence, more often than not, that plasma treatment modifies the seed surface in such a way that water uptake increases; this is either done by poration, removal of the topmost layers or through oxidizing the surface to improve the water interaction. It is not yet clear whether it is through mechanical, chemical means or both and whether this is dependent on the plasma treatment, seed type or both. How much energy needs to be injected into the plasma to obtain these effects? To address this, future studies should continue correlating surface modifications with changes in germination in a systematic manner and clearly record the electrical characteristics. This would clarify if mechanical erosion is necessary to induce a change, under which circumstances mechanical erosion or chemical alterations are needed, and specifically which gas types and electrical properties are needed.
It also seems that most authors agree that RONS are predominantly responsible for the observed plant growth effects. However, it is not yet clear whether it is ROS, RNS or the synergistic action of both. It is also not clear if it is purely a RONS effect because plasma is a synergy of individual mechanisms. Although most authors agree that the effects are not due to heat, it remains difficult to define temperature in these studies, especially in the presence of a gas flow. Is the seed temperature after treatment, the plasma temperature or the temperature of the device measured? Is it certain that an increase of a few degrees is not enough to trigger a heat shock response? Is the relative humidity altered by the plasma heating? For this reason, it would be useful for scientists to include controls in their studies for heat, ozone, electric fields, and humidity, mimicking what they are using in the plasma treatment where possible.
Most authors observe changes at the molecular level although these studies are still extremely limited. More studies focusing on gene and protein expression would at least provide insight about whether the same genes are activated across different plasma treatments and across different seed types. It is also not clear whether these effects are long-term and at which point they would be considered genotoxic or adverse for plant growth. The bottleneck here will be that gene expression studies are limited to already sequenced plant genomes. However, these results may be applicable to closely related species.
As a next step, it would be useful to understand how each parameter in the plasma treatment affects the outcome so it is more predictable to be able to control the output. To accomplish this, it will require the continued collaborative efforts of biologists, chemists and physicists and these standardized protocols will likely need to be tailored to the seed type due to the diversity in the seed coat and build on the information from industry or associations such as AOSA (Association of Official Seed Analysts) where plasma is an added parameter.
Much of the outcome of the plasma-seed treatment seems to depend on both the plasma setup and seed features. If the seed has many layers that need to be scarified, plasma may help with mechanical erosion through etching or by melting the wax with the heat produced as a by-product of plasma generation. If the seed is rather permeable, it may functionalize the surface through the addition of chemical groups on the surface to become more hydrophilic and enhance gas exchange to then affect the seed biochemistry. Therefore, it may be that there are several modes of plasma treatment that can be selected i.e., if you need mechanical scarification and erosion, then use high power AC and argon for ion bombardment, if you need a gentle dose with ROS for chemical modification of seed coat, use nanopulse power for better ROS generation and lower temperature, or if you want to generate NO or H2O2 or if you want to delay or accelerate germination for storage or sowing, respectively, choose the appropriate gas type.
Very little has been done in terms of economic analysis other than by Niemira [189] but it would be useful to mention explicitly the power density of the device, the maximum number of seeds that can be treated to have an effect and potentially disclose the cost of manufacturing the plasma device to then see which designs would be easiest to implement and scale-up. This does not mean one plasma device is used universally as one of the advantages is the flexibility in design so people can adapt their treatment to their surroundings (seeds may vary in their value or importance depending on the country). By understanding the plasma-seed interactions and mechanisms, this will also help with setting up regulations around this technology since it is still ill-defined.
Generally, future studies should ideally correlate results using methods from different fields as far as possible, such as combining material science techniques with biological analysis and recording plasma parameters to find trends across experiments. The most urgent questions are: which treatment parameters are necessary to see a reproducible beneficial effect on seeds, can these parameters be applied to a plethora of seeds or is it necessary to tailor them to each seed type, how is the plasma treatment affecting the seed on a molecular level, is it possible to have these plasma treatments and biological effects consistently reproduced, can the plasma treatment be reliably scaled up for industrial applications, and how does plasma treatment compare to already existing methods such as acid or mechanical scarification? Provided that scientists now focus on the molecular effects of plasmas, with time, we might understand in detail how plasma-seed treatments work to develop this into a viable seed processing technology. Our hope is that plasma treatments will be another technology useful to the agriculture community.
AW conceptualized the review and figures, performed literature search, designed the figures, and wrote the manuscript. IF and AH read and approved the manuscript. All authors contributed to the article and approved the submitted version.
This work was supported by an ad-hoc grant of the Swiss Federal budgetary framework 2017–2020 for the ETH Domain.
The authors declare that the research was conducted in the absence of any commercial or financial relationships that could be construed as a potential conflict of interest.
The authors thank Prof. Pierre Goloubinoff for the useful comments.
2. Dekkers BJ, Pearce S, van Bolderen-Veldkamp RP, Marshall A, Widera P, Gilbert J, et al. Transcriptional dynamics of two seed compartments with opposing roles in Arabidopsis seed germination. Plant Physiol. (2013) 163:205–15. doi: 10.1104/pp.113.223511
3. Andersen EJ, Ali S, Byamukama E, Yen Y, Nepal MP. Disease resistance mechanisms in plants. Genes. (2018) 9:339. doi: 10.3390/genes9070339
4. Pawar VA, Laware SL. Seed priming A critical review. Int J Sci Res Biol Sci. (2018) 31:94–101. doi: 10.26438/ijsrbs/v5i5.94101
5. Lutts S, Benincasa P, Wojtyla L, Kubala S, Pace R, Lechowska K, et al. Seed Priming: New Comprehensive Approaches for an Old Empirical Technique. New Challenges in Seed Biology-Basic and Translational Research Driving Seed Technology. Rijeka: InTechOpen (2016). p. 1–46.
6. Wang W, Chen Q, Hussain S, Mei J, Dong H, Peng S, et al. Pre-sowing seed treatments in direct-seeded early rice: consequences for emergence, seedling growth and associated metabolic events under chilling stress. Sci Rep. (2016) 6:19637. doi: 10.1038/srep19637
7. Pedrini S, Merritt DJ, Stevens J, Dixon K. Seed coating: science or marketing spin? Trends Plant Sci. (2017) 22:106–16. doi: 10.1016/j.tplants.2016.11.002
8. Aktar W, Sengupta D, Chowdhury A. Impact of pesticides use in agriculture: their benefits and hazards. Interdiscip Toxicol. (2009) 2:1–12. doi: 10.2478/v10102-009-0001-7
9. Marican A, Durán-Lara EF. A review on pesticide removal through different processes. Environ Sci Pollut Res. (2018) 25:2051–64. doi: 10.1007/s11356-017-0796-2
10. Choi JS. Seed-surface disinfection and germination effects of grapefruit seed extract (GSE) on Lactuca sativa seeds. Toxicol Environ Health Sci. (2017) 9:169–75. doi: 10.1007/s13530-017-0318-0
11. Tito SI. Utilization of ultrasonic waves (Acheta domesticus) as a biocontrol of mosquito in Malang Agricultural Institute. In: AIP Conference Proceedings, Vol. 1908. East Java: AIP Publishing (2017). p. 050006.
12. Guo Q, Meng Y, Qu G, Wang T, Yang F, Liang D, et al. Improvement of wheat seed vitality by dielectric barrier discharge plasma treatment. Bioelectromagnetics. (2018) 39:120–31. doi: 10.1002/bem.22088
13. Paoletti MG, Pimentel D. Environmental risks of pesticides versus genetic engineering for agricultural pest control. J Agric Environ Ethics. (2000) 12:279–303. doi: 10.1023/A:1009571131089
15. Hussain S, Zheng M, Khan F, Khaliq A, Fahad S, Peng S, et al. Benefits of rice seed priming are offset permanently by prolonged storage and the storage conditions. Sci Rep. (2015) 5:8101. doi: 10.1038/srep08101
16. Li L, Li J, Shen M, Hou J, Shao H, Dong Y, et al. Improving seed germination and peanut yields by cold plasma treatment. Plasma Sci Technol. (2016) 18:1027–33. doi: 10.1088/1009-0630/18/10/10
17. Han Y, Cheng JH, Sun DW. Activities and conformation changes of food enzymes induced by cold plasma: a review. In: Critical Reviews in Food Science and Nutrition, Vol. 59. Oxford: Taylor and Francis Inc. (2019). pp. 794–811.
18. Laroussi M. Cold plasma in medicine and healthcare: the new frontier in low temperature plasma applications. Front Phys. (2020) 8:74. doi: 10.3389/fphy.2020.00074
19. Šimončicová J, Kryštofová S, Medvecká V, Durišová K, Kalináková B. Technical applications of plasma treatments: current state and perspectives. Appl Microbiol Biotechnol. (2019) 103:5117–29. doi: 10.1007/s00253-019-09877-x
20. Sivachandiran L, Khacef A. Enhanced seed germination and plant growth by atmospheric pressure cold air plasma: combined effect of seed and water treatment. RSC Adv. (2017) 7:1822–32. doi: 10.1039/C6RA24762H
21. Sajib SA, Billah M, Mahmud S, Miah M, Hossain F, Omar FB, et al. Plasma activated water: the next generation eco-friendly stimulant for enhancing plant seed germination, vigor and increased enzyme activity, a study on black gram (Vigna mungo L.). Plasma Chem Plasma Proc. (2019) 40:119–43. doi: 10.1007/s11090-019-10028-3
22. Randeniya LK, de Groot GJJB. Non-Thermal plasma treatment of agricultural seeds for stimulation of germination, removal of surface contamination and other benefits: a review. Plasma Proc Polym. (2015) 12:608–23. doi: 10.1002/ppap.201500042
23. Pauzaite G, Malakauskiene A, Nauciene Z, Zukiene R, Filatova I, Lyushkevich V, et al. Changes in Norway spruce germination and growth induced by pre-sowing seed treatment with cold plasma and electromagnetic field: short-term versus long-term effects. Plasma Proc Polym. (2018) 15:1700068. doi: 10.1002/ppap.201700068
24. Puač N, Gherardi M, Shiratani M. Plasma agriculture: a rapidly emerging field. Plasma Proc Polym. (2018) 15:1700174. doi: 10.1002/ppap.201700174
25. Măgureanu M, Sîrbu R, Dobrin D, Gîdea M. Stimulation of the germination and early growth of tomato seeds by non-thermal plasma. Plasma Chem Plasma Proc. (2018) 38:989–1001. doi: 10.1007/s11090-018-9916-0
26. Jinkui F, Decheng W, Changyong S, Zhang L, Xin T. Effects of cold plasma treatment on alfalfa seed growth under simulated drought stress. Plasma Sci Technol. (2018) 20:035505. doi: 10.1088/2058-6272/aa9b27
27. Wu ZH, Chi LH, Bian SF, Xu KZ. Effects of plasma treatment on maize seeding resistance. J Maize Sci. (2007) 15:111–3.
28. Volin JC, Denes FS, Young RA, Park SM. Modification of seed germination performance through cold plasma chemistry technology. Crop Sci. (2000) 40:1706–18. doi: 10.2135/cropsci2000.4061706x
29. Bormashenko E, Grynyov R, Bormashenko Y, Drori E. Cold radiofrequency plasma treatment modifies wettability and germination speed of plant seeds. Sci Rep. (2012) 2:1–8. doi: 10.1038/srep00741
30. Shintani H, Shimizu N, Imanishi Y, Sekiya T, Tamazawa K, Taniguchi A, et al. Inactivation of microorganisms and endotoxins by low temperature nitrogen gas plasma exposure. Biocontrol Sci. (2007) 12:131–43. doi: 10.4265/bio.12.131
31. Shintani H, Sakudo A, Burke P, McDonnell G. Gas plasma sterilization of microorganisms and mechanisms of action. Exp Ther Med. (2010) 1:731–8. doi: 10.3892/etm.2010.136
32. Molina R, López-Santos C, Gómez-Ramírez A, Vílchez A, Espinós JP, González-Elipe AR. Influence of irrigation conditions in the germination of plasma treated Nasturtium seeds. Sci Rep. (2018) 8:16442. doi: 10.1038/s41598-018-34801-0
33. Kopacki M, Pawlat J, Terebun P, Kwiatkowski M, Starek A, Kiczorowski P. Efficacy of non-thermal plasma fumigation to control fungi occurring on onion seeds. In: Electromagnetic Devices and Processes in Environment Protection With Seminar Applications of Superconductors (ELMECO and AoS), 2017 International Conference on IEEE, Naleczow (2017). pp. 1–4.
34. Chen HL, Lee HM, Chen SH, Chang MB, Yu SJ, Li SN. Removal of volatile organic compounds by single-stage and two-stage plasma catalysis systems: a review of the performance 25 enhancement mechanisms, current status, and suitable applications. Environ Sci Technol. (2009) 43:2216–27. doi: 10.1021/es802679b
35. Stryczewska HD, Ebihara K, Takayama M, Gyoutoku Y, Tachibana M. Non-thermal plasma- based technology for soil treatment. Plasma Proc Polym. (2005) 2:238–45. doi: 10.1002/ppap.200400061
36. Pérez-Pizá MC, Prevosto L, Grijalba PE, Zilli CG, Cejas E, Mancinelli B, et al. Improvement of growth and yield of soybean plants through the application of non-thermal plasmas to seeds with different health status. Heliyon. (2019) 5:e01495. doi: 10.1016/j.heliyon.2019.e01495
37. Zhang B, Li R, Yan J. Study on activation and improvement of crop seeds by the application of plasma treating seeds equipment. Arch Biochem Biophys. (2018) 655:37–42. doi: 10.1016/j.abb.2018.08.004
38. Iranbakhsh A, Oraghi Ardebili Z, Molaei H, Oraghi Ardebili N, Amini M. Cold plasma up-regulated expressions of WRKY1 transcription factor and genes involved in biosynthesis of cannabinoids in hemp (Cannabis sativa L.). Plasma Chem Plasma Proc. (2020) 40:527–37. doi: 10.1007/s11090-020-10058-2
39. Kang MH, Veerana M, Eom S, Uhm H, Ryu S, Park G. Plasma mediated disinfection of rice seeds in water and air. J Phys D Appl Phys. (2020) 53:14. doi: 10.1088/1361-6463/ab79de
40. Billah M, Sajib SA, Roy NC, Rashid MM, Reza MA, Hasan MM, et al. Effects of DBD air plasma treatment on the enhancement of black gram (Vigna mungo l.) seed germination and growth. Arch Biochem Biophys. (2020) 681:108253. doi: 10.1016/j.abb.2020.108253
41. Rezaei S, Ghobadian B, Ebadi MT, Jangi F, Ghomi H. Effects of cold plasma on the color parameters of Hyssop (Hyssopus officinalis L.) using color imaging instrumentation and spectrophotometer. Color Res Appl. (2020) 45:29–39. doi: 10.1002/col.22433
42. Koga K, Attri P, Kamataki K, Itagaki N, Shiratani M, Mildažiene V. Impact of radish sprouts seeds coat color on the electron paramagnetic resonance signals after plasma treatment. Jap J Appl Phys. (2020) 59:SHHF01. doi: 10.35848/1347-4065/ab7698
43. Ghasempour M, Iranbakhsh A, Ebadi M, Oraghi Ardebili Z. Seed priming with cold plasma improved seedling performance, secondary metabolism, and expression of deacetylvindoline O-acetyltransferase gene in Catharanthus roseus. Contrib Plasma Phys. (2020) 60:e201900159. doi: 10.1002/ctpp.201900159
44. Mujahid Z, Tounekti T, Khemira H. Cold plasma treatment to release dormancy and improve growth in grape buds: a promising alternative to natural chilling and rest breaking chemicals. Sci Rep. (2020) 10:1–10. doi: 10.1038/s41598-020-59097-x
45. Filatova I, Lyushkevich V, Goncharik S, Zhukovsky A, Krupenko N, Kalatskaja J. The effect of low-pressure plasma treatment of seeds on the plant resistance to pathogens and crop yields. J Phys D Appl Phys. (2020) 53:244001. doi: 10.1088/1361-6463/ab7960
46. Prakrajang K, Sarapirom S, Mai Sci CJ, Thisawech M, Saritnum O, Phakham W. Effects of Plasma Technique and Gamma Irradiation on Seed Germination and Seedling Growth of Chili Pepper, Vol. 47 (2020). Available online at: http://epg.science.cmu.ac.th/ejournal/.
47. Kobayashi M, Wang Y, Kumagai S, Uraoka Y, Ito T. Effects of cold atmospheric plasma irradiation on Arabidopsis seedlings. Jap J Appl Phys. (2020) 59:SAAB09. doi: 10.7567/1347-4065/ab4e7b
48. Dawood N. Effect of RF plasma on Moringa seeds germination and growth. J Taibah Univ Sci. (2020) 14:279–84. doi: 10.1080/16583655.2020.1713570
49. Sidik MAB, Buntat Z, Nawawi Z, Jambak MI, Buntat Y, Musa FN. Effects of cold plasma treatment on the growth rate of corn and eggplant plants. In: Proceedings of 2018 International Conference on Electrical Engineering and Computer Science, ICECOS 2018 (2019). p. 441–6.
50. Ambrico PF, Šimek M, Ambrico M, Morano M, Prukner V, Minafra A, et al. On the air atmospheric pressure plasma treatment effect on the physiology, germination and seedlings of basil seeds. J Phys D Appl Phys. (2019) 53:104001. doi: 10.1088/1361-6463/ab5b1b
51. Seddighinia FS, Iranbakhsh A, Oraghi Ardebili Z, Nejad Satari T, Soleimanpour S. Seed Priming with cold plasma and multi-walled carbon nanotubes modified growth, tissue differentiation, anatomy, and yield in bitter melon (Momordica charantia). J Plant Growth Regul. (2019) 39:87–98. doi: 10.1007/s00344-019-09965-2
52. Gao X, Zhang A, Héroux P, Sand W, Sun Z, Zhan J, et al. Effect of dielectric barrier discharge cold plasma on pea seed growth. J Agric Food Chem. (2019) 67:10813–22. doi: 10.1021/acs.jafc.9b03099
53. Mildažiene V, Aleknavičiute V, Žukiene R, PauŽaite G, Naučiene Z, Filatova I, et al. Treatment of common sunflower (Helianthus annus L.) seeds with radio-frequency electromagnetic field and cold plasma induces changes in seed phytohormone balance, seedling development and leaf protein expression. Sci Rep. (2019) 9:1–12. doi: 10.1038/s41598-019-42893-5
54. Šerá B, Zahoranová A, Bujdáková H, Šerý M. Disinfection from pine seeds contaminated with Fusarium circinatum Nirenberg and O'Donnell using non-thermal plasma treatment. Romanian Rep Phys. (2019) 71.
55. Cui D, Yin Y, Wang J, Wang Z, Ding H, Ma R, et al. Research on the physio-biochemical mechanism of non-thermal plasma-regulated seed germination and early seedling development in arabidopsis. Front Plant Sci. (2019) 10:1322. doi: 10.3389/fpls.2019.01322
56. Liu B, Honnorat B, Yang H, Arancibia J, Rajjou L, Rousseau A. Non-thermal DBD plasma array on seed germination of different plant species. J Phys D Appl Phys. (2019) 52:025401. doi: 10.1088/1361-6463/aae771
57. Los A, Ziuzina D, Boehm D, Cullen PJ, Bourke P. Investigation of mechanisms involved in germination enhancement of wheat (Triticum aestivum) by cold plasma: effects on seed surface chemistry and characteristics. Plasma Proc Polymers. (2019) 16:1800148. doi: 10.1002/ppap.201800148
58. Moghanloo M, Iranbakhsh A, Ebadi M, Nejad Satari T, Oraghi Ardebili Z. Seed priming with cold plasma and supplementation of culture medium with silicon nanoparticle modified growth, physiology, and anatomy in Astragalus fridae as an endangered species. Acta Physiol Plant. (2019) 41:1–13. doi: 10.1007/s11738-019-2846-5
59. Babajani A, Iranbakhsh A, Oraghi Ardebili Z, Eslami B. Seed priming with non-thermal plasma modified plant reactions to selenium or zinc oxide nanoparticles: cold plasma as a novel emerging tool for plant science. Plasma Chem Plasma Proc. (2019) 39:21–34. doi: 10.1007/s11090-018-9934-y
60. Lotfy K, Al-Harbi NA, Abd El-Raheem H. Cold atmospheric pressure nitrogen plasma jet for enhancement germination of wheat seeds. Plasma Chem Plasma Proc. (2019) 39:897–912. doi: 10.1007/s11090-019-09969-6
61. Bafoil M, Le Ru A, Merbahi N, Eichwald O, Dunand C, Yousfi M. New insights of low-temperature plasma effects on germination of three genotypes of Arabidopsis thaliana seeds under osmotic and saline stresses. Sci Rep. (2019) 9:8649. doi: 10.1038/s41598-019-44927-4
62. Singh R, Prasad P, Mohan R, Verma MK, Kumar B Radiofrequency cold plasma treatment enhances seed germination and seedling growth in variety CIM-Saumya of sweet basil (Ocimum basilicum L.). J Appl Res Med Aromatic Plants. (2019) 12:78–81. doi: 10.1016/j.jarmap.2018.11.005
63. Iqbal T, Farooq M, Afsheen S, Abrar M, Yousaf M, Ijaz M. Cold plasma treatment and laser irradiation of Triticum spp. seeds for sterilization and germination. J Laser Appl. (2019) 31:042013. doi: 10.2351/1.5109764
64. Islam S, Omar FB, Sajib SA, Roy NC, Reza A, Hasan M, et al. Effects of LPDBD plasma and plasma activated water on germination and growth in rapeseed (Brassica napus). Gesunde Pflanzen. (2019) 71:175–85. doi: 10.1007/s10343-019-00463-9
65. Kabir AH, Rahman MM, Das U, Sarkar U, Roy NC, Reza MA, et al. Reduction of cadmium toxicity in wheat through plasma technology. PLoS ONE. (2019) 14:e0214509. doi: 10.1371/journal.pone.0214509
66. Afsheen S, Fatima U, Iqbal T, Abrar M, Muhammad S, Saeed A, et al. Influence of cold plasma treatment on insecticidal properties of wheat seeds against red flour beetles. PlST. (2019) 21:085506. doi: 10.1088/2058-6272/ab19ee
67. Lo Porto C, Sergio L, Boari F, Logrieco AF, Cantore V. Cold plasma pretreatment improves the germination of wild asparagus (Asparagus acutifolius L.) seeds. Sci Hortic. (2019) 256:108554. doi: 10.1016/j.scienta.2019.108554
68. Jiang J, Li J, Dong Y. Effect of cold plasma treatment on seedling growth and nutrient absorption of tomato. Plasma Sci Technol. (2018) 20:044007. doi: 10.1088/2058-6272/aaa0bf
69. Hosseini SI, Mohsenimehr S, Hadian J, Ghorbanpour M, Shokri B. Physico-chemical induced modification of seed germination and early development in artichoke (Cynara scolymus L.) using low energy plasma technology. Phys Plasmas. (2018) 25:013525. doi: 10.1063/1.5016037
70. Tounekti T, Mujahid ZUI, Khemira H. Non-thermal dielectric barrier discharge (DBD) plasma affects germination of coffee and grape seeds. AIP Conf Proc. (2018) 1976:020029. doi: 10.1063/1.5042396
71. Khatami S, Ahmadinia A. Increased germination and growth rates of pea and Zucchini seed by FSG plasma. J Theoret Appl Phys. (2018) 12:33–8. doi: 10.1007/s40094-018-0280-5
72. Pawłat J, Starek A, Sujak A, Terebun P, Kwiatkowski M, Budzeń M, et al. Effects of atmospheric pressure plasma jet operating with DBD on Lavatera thuringiaca L. seeds' germination. PLoS ONE. (2018) 13:e0194349. doi: 10.1371/journal.pone.0194349
73. Rahman MM, Sajib SA, Rahi MS, Tahura S, Roy NC, Parvez S, et al. Mechanisms and signaling associated with LPDBD plasma mediated growth improvement in wheat. Sci Rep. (2018) 8:1–11. doi: 10.1038/s41598-018-28960-3
74. Hayashi N, Ono R, Nakano R, Shiratani M, Tashiro K, Kuhara S, et al. DNA microarray analysis of plant seeds irradiated by active oxygen species in oxygen plasma. Plasma Med. (2016) 6:459–71. doi: 10.1615/PlasmaMed.2016018933
75. Matra K. Atmospheric non-thermal argon-oxygen plasma for sunflower seedling growth improvement. Jpn J Appl Phys. (2018) 57:01AG03. doi: 10.7567/JJAP.57.01AG03
76. Bafoil M, Jemmat A, Martinez Y, Merbahi N, Eichwald O, Dunand C, et al. Effects of low temperature plasmas and plasma activated waters on Arabidopsis thaliana germination and growth. PLoS ONE. (2018) 13:e0195512. doi: 10.1371/journal.pone.0195512
77. Štěpánová V, Slavíček P, Kelar J, Prášil J, Smékal M, Stupavská M, et al. Atmospheric pressure plasma treatment of agricultural seeds of cucumber (Cucumis sativus L.) and pepper (Capsicum annuum L.) with effect on reduction of diseases and germination improvement. Plasma Processes Polym. (2018) 15:1700076. doi: 10.1002/ppap.201700076
78. Iranbakhsh A, Ardebili NO, Ardebili ZO, Shafaati M, Ghoranneviss M. Non-thermal plasma induced expression of heat shock factor A4A and improved wheat (Triticum aestivum L.) growth and resistance against salt stress. Plasma Chem Plasma Proc. (2018) 38:29–44. doi: 10.1007/s11090-017-9861-3
79. Iranbakhsh A, Oraghi Ardebili Z, Oraghi Ardebili N, Ghoranneviss M, Safari N. Cold plasma relieved toxicity signs of nano zinc oxide in Capsicum annuum cayenne via modifying growth, differentiation, and physiology. Acta Physiol Plantarum. (2018) 40:154. doi: 10.1007/s11738-018-2730-8
80. Park Y, Oh KS, Oh J, Seok DC, Kim SB, Yoo SJ, et al. The biological effects of surface dielectric barrier discharge on seed germination and plant growth with barley. Plasma Proc Polym. (2018) 15:1600056. doi: 10.1002/ppap.201600056
81. Zhang J, Kwon T, Kim S, Jeong D. Plasma farming: non-thermal dielectric barrier discharge plasma technology for improving the growth of soybean sprouts and chickens. Plasma. (2018) 1:285–96. doi: 10.3390/plasma1020025
82. Shapira Y, Chaniel G, Bormashenko E. Surface charging by the cold plasma discharge of lentil and pepper seeds in comparison with polymers. Colloids Surf B Biointerfaces. (2018) 172:541–4. doi: 10.1016/j.colsurfb.2018.09.004
83. Wang XQ, Zhou RW, De Groot G, B.azaka K, Murphy AB, Ostrikov KK. Spectral characteristics of cotton seeds treated by a dielectric barrier discharge plasma. Sci Rep. (2017) 7:1–9. doi: 10.1038/s41598-017-04963-4
84. Kim J-W, Puligundla P, Mok C. Effect of corona discharge plasma jet on surface-borne microorganisms and sprouting of broccoli seeds. J Sci Food Agric. (2017) 97:128–34. doi: 10.1002/jsfa.7698
85. Mildažiene V, Pauzaite G, Nauciené Z, Malakauskiene A, Zukiene R, Januskaitiene I, et al. Pre-sowing seed treatment with cold plasma and electromagnetic field increases secondary metabolite content in purple coneflower (Echinacea purpurea) leaves. Plasma Processes and Polymers. (2018) 15:1700059. doi: 10.1002/ppap.201700059
86. Li Y, Wang T, Meng Y, Qu G, Sun Q, Liang D, et al. Air atmospheric dielectric barrier discharge plasma induced germination and growth enhancement of wheat seed. Plasma Chem Plasma Proc. (2017) 37:1621–34. doi: 10.1007/s11090-017-9835-5
87. Sera B, Sery M, Gavril B, Gajdova I. Seed germination and early growth responses to seed pre-treatment by non-thermal plasma in hemp cultivars (Cannabis sativa L.). Plasma Chem Plasma Proc. (2017) 37:207–21. doi: 10.1007/s11090-016-9763-9
88. Puligundla P, Kim JW, Mok C. Effect of corona discharge plasma jet treatment on decontamination and sprouting of rapeseed (Brassica napus L.) seeds. Food Control. (2017) 71:376–82. doi: 10.1016/j.foodcont.2016.07.021
89. Puligundla P, Kim JW, Mok C. Effects of non-thermal plasma treatment on decontamination and sprouting of radish (Raphanus sativus L.) seeds. Food Bioproc Technol. (2017) 10:1093–102. doi: 10.1007/s11947-017-1886-3
90. Gómez-Ramírez A, López-Santos C, Cantos M, García JL, Molina R, Cotrino J, et al. Surface chemistry and germination improvement of Quinoa seeds subjected to plasma activation. Sci Rep. (2017) 7:1–12. doi: 10.1038/s41598-017-06164-5
91. Guo Q, Wang Y, Zhang H, Qu G, Wang T, Sun Q, et al. Alleviation of adverse effects of drought stress on wheat seed germination using atmospheric dielectric barrier discharge plasma treatment. Sci Rep. (2017) 7:1–14. doi: 10.1038/s41598-017-16944-8
92. Zhang JJ, Jo JO, Huynh DL, Mongre RK, Ghosh M, Singh AK, et al. Growth-inducing effects of argon plasma on soybean sprouts via the regulation of demethylation levels of energy metabolism-related genes. Sci Rep. (2017) 7:1–12. doi: 10.1038/srep41917
93. da Silva ARM, Farias ML, da Silva DLS, Vitoriano JO, de Sousa RC, Alves-Junior C. Using atmospheric plasma to increase wettability, imbibition and germination of physically dormant seeds of Mimosa Caesalpiniafolia. Colloids Surf B Biointerfaces. (2017) 157:280–5. doi: 10.1016/j.colsurfb.2017.05.063
94. Meng Y, Qu G, Wang T, Sun Q, Liang D, Hu S. Enhancement of germination and seedling growth of wheat seed using dielectric barrier discharge plasma with various gas sources. Plasma Chem Plasma Proc. (2017) 37:1105–19. doi: 10.1007/s11090-017-9799-5
95. Nalwa C, Thakur AK, Vikram A, Rane R, Vaid A. Studies on plasma treatment and priming of seeds of bell pepper (Capsicum annuum L.). J Appl Nat Sci. (2017) 9:1505–9. doi: 10.31018/jans.v9i3.1392
96. Junior CA, De Oliveira Vitoriano J, Da Silva DLS, De Lima Farias M, De Lima Dantas NB. Water uptake mechanism and germination of Erythrina velutina seeds treated with atmospheric plasma. Sci Rep. (2016) 6:33722. doi: 10.1038/srep33722
97. Zahoranová A, Henselová M, Hudecová D, Kalináková B, Kováčik D, Medvecká V, et al. Effect of cold atmospheric pressure plasma on the wheat seedlings vigor and on the inactivation of microorganisms on the seeds surface. Plasma Chem Plasma Proc. (2016) 36:397–414. doi: 10.1007/s11090-015-9684-z
98. Zhou R, Zhou R, Zhang X, Zhuang J, Yang S, Bazaka K, et al. Effects of atmospheric-pressure N2, He, Air, and O2 microplasmas on mung bean seed germination and seedling growth. Sci Rep. (2016) 6:32603. doi: 10.1038/srep32603
99. Khamsen N, Onwimol D, Teerakawanich N, Dechanupaprittha S, Kanokbannakorn W, Hongesombut K, et al. Rice (Oryza sativa L.) seed sterilization and germination enhancement via atmospheric hybrid nonthermal discharge plasma. ACS Appl Mater Interfaces. (2016) 8:19268–75. doi: 10.1021/acsami.6b04555
100. Gholami A, Navab Safa N, Khoram M, Hadian J, Ghomia H. Effect of low-pressure radio frequency plasma on ajwain seed germination. Plasma Med. (2016) 6:389–96. doi: 10.1615/PlasmaMed.2017019157
101. Matra K. Non-thermal plasma for germination enhancement of radish seeds. Proc Comput Sci. (2016) 86:132–5. doi: 10.1016/j.procs.2016.05.033
102. Sarinont T, Amano T, Attri P, Koga K, Hayashi N, Shiratani M. Effects of plasma irradiation using various feeding gases on growth of Raphanus sativus L. Arch Biochem Biophys. (2016) 605:129–40. doi: 10.1016/j.abb.2016.03.024
103. Dobrin D, Magureanu M, Mandache NB, Ionita MD. The effect of non-thermal plasma treatment on wheat germination and early growth. Innov Food Sci Emerg Technol. (2015) 29:255–60. doi: 10.1016/j.ifset.2015.02.006
104. Stolárik T, Henselová M, Martinka M, Novák O, Zahoranová A, Cernák M. Effect of low-temperature plasma on the structure of seeds, growth and metabolism of endogenous phytohormones in pea (Pisum sativum L.). Plasma Chem Plasma Proc. (2015) 35:659–76. doi: 10.1007/s11090-015-9627-8
105. Munkhuu N, Shao C, Wang D, Liu L, Muhammad I, He C, et al. Stimulating effect of low-temperature plasma on seed germination characteristics of Trifolium repens. In: Li D., Chen Y. (eds) Computer and Computing Technologies in Agriculture VIII. CCTA 2014. IFIP Advances in Information and Communication Technology, Cham: Springer (2015) 452. doi: 10.1007/978-3-319-19620-6_21
106. Ji SH, Kim T, Panngom K, Hong YJ, Pengkit A, Park DH, et al. Assessment of the effects of nitrogen plasma and plasma-generated nitric oxide on early development of Coriandum sativum. Plasma Proc Polymers. (2015) 12:1164–73. doi: 10.1002/ppap.201500021
107. Kadowaki K, Kurisaka N. Stimulation and inhibition of Arabidopsis seed germination with repetitive barrier discharges produced by polarity-reversed voltage pulses. Electr Commun Japan. (2014) 97:38–43. doi: 10.1002/ecj.11603
108. Butscher D, Waskow A, von Rohr PR. Disinfection of granular food products using cold plasma. In: Bermudez-Arguirre D, editor Advances in Cold Plasma Applications for Food Safety and Preservation. Richland, WA: Academic Press (2020). pp. 185–228.
109. Lotfy K. Effects of cold atmospheric plasma jet treatment on the seed germination and enhancement growth of watermelon. Open J Appl Sci. (2017) 7:705–19. doi: 10.4236/ojapps.2017.712050
110. Kitazaki S, Sarinont T, Koga K, Hayashi N, Shiratani M. Plasma induced long-term growth enhancement of Raphanus sativus L. using combinatorial atmospheric air dielectric barrier discharge plasmas. Curr Appl Phys. (2014) 14:S149–53. doi: 10.1016/j.cap.2013.11.056
111. Al-Whaibi MH. Plant heat-shock proteins: a mini review. J King Saud Univ Sci. (2011) 23:139–50. doi: 10.1016/j.jksus.2010.06.022
112. Farokh P, Mahmoodzadeh H, Satari TN. Response of seed germination of safflower to UV-B radiation. Res J Environ Sci. (2010) 4:70–4. doi: 10.3923/rjes.2010.70.74
113. Noble RE. Effects of UV-irradiation on seed germination. Sci Total Environ. (2002) 299:173–6. doi: 10.1016/S0048-9697(02)00232-2
114. Sadeghianfar P, Nazari M, Backes G. Exposure to Ultraviolet (UV-C) radiation increases germination rate of maize (Zea maize L.) and sugar beet (Beta vulgaris) seeds. Plants. (2019) 8:49. doi: 10.3390/plants8020049
115. Ibrahim M, Imram M, Hussain A, Aslam M, Rehmani FS, Ali B, et al. Phytochemical studies on Amberboa ramose. Pak J Chem. (2012) 2:24–8. doi: 10.15228/2012.v02.i01.p04
116. Caldwell MM, Björn LO, Bornman JF, Flint SD, Kulandaivelu G, Teramura AH, et al. Effects of increased solar ultraviolet radiation on terrestrial ecosystems. J Photochem Photobiol B Biol. (1998) 46:40–52. doi: 10.1016/S1011-1344(98)00184-5
117. Veberic R. The impact of production technology on plant phenolics. Horticulturae. (2016) 2:8. doi: 10.3390/horticulturae2030008
118. Iranbakhsh A, Ghoranneviss M, Oraghi Ardebili Z, Ardebili NO, Hesami Tackallou S, Nikmaram H. Non-thermal plasma modified growth and physiology in Triticum aestivum via generated signaling molecules and UV radiation. Biol Plant. (2017) 61:702–8. doi: 10.1007/s10535-016-0699-y
119. Huché-Thélier L, Crespel L, Le Gourrierec J, Morel P, Sakr S, Leduc N. Light signaling and plant responses to blue and UV radiations—perspectives for applications in horticulture. Environ Exp Bot. (2016) 121:22–38. doi: 10.1016/j.envexpbot.2015.06.009
120. Su B, Guo J, Nian W, Feng H, Wang K, Zhang J, et al. Early growth effects of nanosecond pulsed electric field (nsPEFs) exposure on Haloxylon ammodendron. Plasma Proc Polym. (2015) 12:372–9. doi: 10.1002/ppap.201400131
121. Dymek K, Dejmek P, Panarese V, Vicente AA, Wadsö L, Finnie C, et al. Effect of pulsed electric field on the germination of barley seeds. Lwt-Food Sci Technol. (2012) 47:161–6. doi: 10.1016/j.lwt.2011.12.019
122. Teissie J, Golzio M, Rols MP. Mechanisms of cell membrane electropermeabilization: a minireview of our present (lack of?) knowledge. Biochim Biophys Acta Gen Subj. (2005) 1724:270–80. doi: 10.1016/j.bbagen.2005.05.006
123. Patwardhan MS, Gandhare WZ. High voltage electric field effects on the germination rate of tomato seeds. Acta Agrophys. (2013) 20, 403–13.
124. Maffei ME. Magnetic field effects on plant growth, development, and evolution. Front Plant Sci. (2014) 5:445. doi: 10.3389/fpls.2014.00445
125. Bilalis DJ, Katsenios N, Efthimiadou A, Karkanis A, Efthimiadis P. Investigation of pulsed electromagnetic field as a novel organic pre-sowing method on germination and initial growth stages of cotton. Electromagn Biol Med. (2012) 31:143–50. doi: 10.3109/15368378.2011.624660
126. Souza FH, Marcos-Filho J. The seed coat as a modulator of seed-environment relationships in Fabaceae. Braz J Bot. (2001) 24:365–75. doi: 10.1590/S0100-84042001000400002
127. Sehrawat R, Thakur AK, Vikram A, Vaid A, Rane R. Effect of cold plasma treatment on physiological quality of okra seed. J Hill Agric. (2017) 8:66–71. doi: 10.5958/2230-7338.2017.00010.6
128. Mildažiene V, Pauzaite G, Malakauskiene A, Zukiene R, Nauciene Z, Filatova I, et al. Response of perennial woody plants to seed treatment by electromagnetic field and low-temperature plasma. Bioelectromagnetics. (2016) 37:536–48. doi: 10.1002/bem.22003
129. Sera B, Spatenka P, Sery M, Vrchotova N, Hruskova I. Influence of plasma treatment on wheat and oat germination and early growth. IEEE Transac Plasma Sci. (2010) 38:2963–8. doi: 10.1109/TPS.2010.2060728
130. Holc M, Primc G, Iskra J, Titan P, Kovač J, Mozetič M, et al. Effect of oxygen plasma on sprout and root growth, surface morphology and yield of garlic. Plants. (2019) 8:462. doi: 10.3390/plants8110462
131. Yamauchi Y, Kuzuya M, Sasai Y, Kondo S Surface treatment of natural polymer by plasma technique - promotion of seed germination. J Photopol Sci Technol. (2012) 25:535–8. doi: 10.2494/photopolymer.25.535
132. Pawlat J, Starek A, Sujak A, Kwiatkowski M, Terebun P, Budzeń M. Effects of atmospheric pressure plasma generated in GlidArc reactor on Lavatera thuringiaca L. seeds' germination. Plasma Proc Polym. (2018) 15:1700064. doi: 10.1002/ppap.201700064
133. Cakmak I. The role of potassium in alleviating detrimental effects of abiotic stresses in plants. J Plant Nutr Soil Sci. (2005) 168:521–30. doi: 10.1002/jpln.200420485
134. Wang M, Zheng Q, Shen Q, Guo S. The critical role of potassium in plant stress response. Int J Mol Sci. (2013) 14:7370–90. doi: 10.3390/ijms14047370
135. Miguel MVDC, Marcos Filho J. Potassium leakage and maize seed physiological potential. Sci Agric. (2002) 59:315–19. doi: 10.1590/S0103-90162002000200017
136. Carvalho LFD, Sediyama CS, Reis MS, Dias DC, Moreira MA. Influência da temperatura de embebição da semente de soja no teste de condutividade elétrica para avaliação da qualidade fisiológica. Rev Brasil Sementes. (2009) 31:9–17. doi: 10.1590/S0101-31222009000100001
137. Ahmed AKA, Shi X, Hua L, Manzueta L, Qing W, Marhaba T, et al. Influences of air, oxygen, nitrogen, and carbon dioxide nanobubbles on seed germination and plant growth. J Agric Food Chem. (2018) 66:5117–24. doi: 10.1021/acs.jafc.8b00333
138. Leghari SJ, Wahocho NA, Laghari GM, HafeezLaghari A, MustafaBhabhan G, HussainTalpur K, et al. Role of nitrogen for plant growth and development: a review. Adv Environ Biol. (2016) 10:209–19.
139. Filatova I, Azharonok V, Kadyrov M, Beljavsky V, Gvozdov A, Shik A, et al. The effect of plasma treatment of seeds of some grain and legumes on their sowing quality and productivity. Rom J Phys. (2011) 56:139–43.
140. El-Maarouf-Bouteau H, Bailly C. Oxidative signaling in seed germination and dormancy. Plant Signal Behav. (2008) 3:175–82. doi: 10.4161/psb.3.3.5539
141. Siddiqui ZS, Khan MA. The role of seed coat phenolics on water uptake and early protein synthesis during germination of dimorphic seeds of Halopyrum mucronatum (L.) Staph. Pak J Bot. (2010) 42:227–38.
142. Müller K, Hess B, Leubner-Metzger G. Chapter V: a role for Reactive Oxygen Species in Endosperm Weakening. In: Adkins SW, Navie SC, Ashmore S, editors. Germination and Dormancy of Seeds: Biology, Development and Ecology. Wallingford: CAB International (2007). p. 287.
143. Weber D, Davies MJ, Grune T. Determination of protein carbonyls in plasma, cell extracts, tissue homogenates, isolated proteins: focus on sample preparation and derivatization conditions. Redox Biol. (2015) 5:367–80. doi: 10.1016/j.redox.2015.06.005
144. Oracz K, Bouteau HEM, Farrant JM, Cooper K, Belghazi M, Job C, et al. ROS production and protein oxidation as a novel mechanism for seed dormancy alleviation. Plant J. (2007) 50:452–65. doi: 10.1111/j.1365-313X.2007.03063.x
145. Valderrama R, Begara-Morales JC, Chaki M, Mata-Pérez C, Padilla MN, Barroso JB. Hydrogen Peroxide (H2O2)- and Nitric Oxide (NO)-derived posttranslational modifications. In: Gupta D, Palma J, Corpas F, editors. Nitric Oxide and Hydrogen Peroxide Signaling in Higher Plants. Cham: Springer (2019) 37–67.
146. Ahmad P. Oxidative Damage to Plants: Antioxidant Networks and Signaling. Chapter 2. Srinagar: Elsevier Academic Press (2004).
147. Grene R. Oxidative stress and acclimation mechanisms in plants. Arabidopsis Book. (2002) 1:e0036. doi: 10.1199/tab.0036.1
148. Yusupov M, Razzokov J, Cordeiro RM, Bogaerts A. Transport of reactive oxygen and nitrogen species across aquaporin: a molecular level picture. Oxid Med Cell Longev. (2019) 2019:2930504. doi: 10.1155/2019/2930504
149. Seol YB, Kim J, Park SH, Chang HY. Atmospheric pressure pulsed plasma induces cell death in photosynthetic organs via intracellularly generated ROS. Sci Rep. (2017) 7:1–11. doi: 10.1038/s41598-017-00480-6
150. Mhamdi A, Van Breusegem F. Reactive oxygen species in plant development. Development. (2018) 145:dev164376. doi: 10.1242/dev.164376
151. Torsethaugen G, Pell EJ, Assmann SM. Ozone inhibits guard cell K+ channels implicated in stomatal opening. Proc Nat Acad Sci USA. (1999) 96:13577–82. doi: 10.1073/pnas.96.23.13577
152. Chaudhary N, Agrawal SB. The role of elevated ozone on growth, yield and seed quality amongst six cultivars of mung bean. Ecotoxicol Environ Saf . (2015) 111:286–94. doi: 10.1016/j.ecoenv.2014.09.018
153. Avdeeva V, Zorina E, Bezgina J, Kolosova O. Influence of ozone on germination and germinating energy of winter wheat seeds. In: Proceedings of the International Scientific Conference. Jelgava: Latvijas Lauksaimniecibas universitāte (2018).
154. Rodoni L, Casadei N, Concellón A, Chaves Alicia AR, Vicente AR. Effect of short-term ozone treatments on tomato (Solanum lycopersicum L.) fruit quality and cell wall degradation. J Agric Food Chem. (2009) 58:594–9. doi: 10.1021/jf9029145
155. Abeli T, Guasconi DB, Mondoni A, Dondi D, Bentivoglio A, Buttafava A, et al. Acute and chronic ozone exposure temporarily affects seed germination in alpine plants. Plant Biosyst. (2017) 151:304–15. doi: 10.1080/11263504.2016.1174169
156. Emberson LD, Pleijel H, Ainsworth EA, Van den Berg M, Ren W, Osborne S, et al. Ozone effects on crops and consideration in crop models. Eur J Agron. (2018) 100:19–34. doi: 10.1016/j.eja.2018.06.002
157. Sudhakar N, Nagendra-Prasad D, Mohan N, Hill B, Gunasekaran M, Murugesan K. Assessing influence of ozone in tomato seed dormancy alleviation. Am J Plant Sci. (2011) 2:443. doi: 10.4236/ajps.2011.23051
158. Giba Z, Grubisic D, Konjevic R. Nitrogen oxides as environmental sensors for seeds. Seed Sci Res. (2003) 13:187. doi: 10.1079/SSR2003136
159. Puač N, Živković S, Selaković N, Milutinović M, Boljević J, Malović G, et al. Long and short term effects of plasma treatment on meristematic plant cells. Appl Phys Lett. (2014) 104:214106. doi: 10.1063/1.4880360
160. Henselová M, Slováková L, Martinka M, Zahoranová A. Growth, anatomy and enzyme activity changes in maize roots induced by treatment of seeds with low-temperature plasma. Biologia. (2012) 67:490–7. doi: 10.2478/s11756-012-0046-5
161. Noctor G, Reichheld JP, Foyer CH. ROS-related redox regulation and signaling in plants. Semin Cell Dev Biol. (2017) 80:3–12. doi: 10.1016/j.semcdb.2017.07.013
162. Yang H, Mu J, Chen L, Feng J, Hu J, Li L, et al. S-nitrosylation positively regulates ascorbate peroxidase activity during plant stress responses. Plant Physiol. (2015) 167:1604–15. doi: 10.1104/pp.114.255216
163. Romero-Puertas MC, Sandalio LM. Nitric oxide level is self-regulating and also regulates its ROS partners. Front Plant Sci. (2016) 7:316. doi: 10.3389/fpls.2016.00316
164. Jalmi SK, Sinha AK. ROS mediated MAPK signaling in abiotic and biotic stress-striking similarities and differences. Front Plant Sci. (2015) 6:769. doi: 10.3389/fpls.2015.00769
165. Wilkins KA, Matthus E, Swarbreck SM, Davies JM. Calcium-mediated abiotic stress signaling in roots. Front Plant Sci. (2016) 7:1296. doi: 10.3389/fpls.2016.01296
166. Smýkal P, Vernoud V, Blair MW, Soukup A, Thompson RD. The role of the testa during development and in establishment of dormancy of the legume seed. Front Plant Sci. (2014) 5:351. doi: 10.3389/fpls.2014.00351
167. Sabelli PA, Larkins BA, editors. Advances in Seed Biology. Lausanne: Frontiers Media SA (2015). p. 238–41.
168. Ji SH, Choi KH, Pengkit A, Im JS, Kim JS, Kim YH, et al. Effects of high voltage nanosecond pulsed plasma and micro DBD plasma on seed germination, growth development and physiological activities in spinach. Arch Biochem Biophys. (2016) 605:117–28. doi: 10.1016/j.abb.2016.02.028
169. Ji SH, Ki SH, Kang MH, Choi JS, Park Y, Oh J, et al. Characterization of physical and biochemical changes in plasma treated spinach seed during germination. J Phys D Appl Phys. (2018) 51:145205. doi: 10.1088/1361-6463/aab2a2
170. Perez Pizá MC, Prevosto L, Zilli C, Cejas E, Kelly H, Balestrasse K. Effects of non–thermal plasmas on seed-borne Diaporthe/Phomopsis complex and germination parameters of soybean seeds. Innov Food Sci Emerg Technol. (2018) 49:82–91. doi: 10.1016/j.ifset.2018.07.009
171. Wang C, Zhao Y, Gu P, Zou F, Meng L, Song W, et al. Auxin is involved in lateral root formation induced by drought stress in tobacco seedlings. J Plant Growth Regul. (2018) 37:539–49. doi: 10.1007/s00344-017-9752-0
173. Fabregas N, Formosa-Jordan P, Confraria A, Siligato R, Alonso JM, Swarup R, et al. Auxin influx carriers control vascular patterning and xylem differentiation in Arabidopsis thaliana. PLoS Genet. (2015) 11:e1005183. doi: 10.1371/journal.pgen.1005183
174. Safari N, Iranbakhsh A, Ardebili ZO. Non-thermal plasma modified growth and differentiation process of Capsicum annuum PP805 Godiva in in vitro conditions. Plasma Sci Technol. (2017) 19:055501. doi: 10.1088/2058-6272/aa57ef
175. Ljung KAuxin metabolism and homeostasis during plant development. Development. (2013) 140:943–50. doi: 10.1242/dev.086363
176. Tian WN, Braunstein LD, Pang J, Stuhlmeier KM, Xi QC, Tian X, et al. Importance of glucose-6-phosphate dehydrogenase activity for cell growth. J Biol Chem. (1998) 273:10609–17. doi: 10.1074/jbc.273.17.10609
177. Scholtz V, Pazlarova J, Souskova H, Khun J, Julak J. Nonthermal plasma — a tool for decontamination and disinfection. Biotechnol Adv. (2015) 33:1108–19. doi: 10.1016/j.biotechadv.2015.01.002
178. Moon UR, Mitra A. A mechanistic insight into hydrogen peroxide-mediated elicitation of bioactive xanthones in Hoppea fastigiata shoot cultures. Planta. (2016) 244:259–74. doi: 10.1007/s00425-016-2506-6
179. Noctor G, Lelarge-Trouverie C, Mhamdi A. The metabolomics of oxidative stress. Phytochemistry. (2015) 112:33–53. doi: 10.1016/j.phytochem.2014.09.002
180. Jain D, Khurana JP. Role of pathogenesis-related (PR) proteins in plant defense mechanism. In: Singh A, Singh I, editors Molecular Aspects of plant-Pathogen Interaction. Singapore: Springer (2018). pp. 265–81.
181. Perez SM, Biondi E, Laurita R, Proto M, Sarti F, Gherardi M, et al. Plasma activated water as resistance inducer against bacterial leaf spot of tomato. PLoS ONE. (2019) 14:e0217788. doi: 10.1371/journal.pone.0217788
182. Hayashi N, Nakahigashi A, Goto M, Kitazaki S, Koga K, Shiratani M. Redox characteristics of thiol compounds using radicals produced by water vapor radio frequency discharge. Jap J Appl Phys. (2011) 50:08JF04. doi: 10.1143/JJAP.50.08JF04
183. Phukan UJ, Jeena GS, Shukla RK. WRKY transcription factors: molecular regulation and stress responses in plants. Front Plant Sci. (2016) 7:760. doi: 10.3389/fpls.2016.00760
184. Mira S, Pirredda M, Martín-Sánchez M, Marchessi JE, Martín C. DNA methylation and integrity in aged seeds and regenerated plants. Seed Sci Res. (2020) 30:1–9. doi: 10.1017/S0960258520000021
185. Kyzek S, Holubová L, Medvecká V, Zahoranová A, Ševčovičová A, Gálová E. Genotoxic effect of low temperature plasma treatment on plant seeds. Toxicol Lett. (2017) 280:S119. doi: 10.1016/j.toxlet.2017.07.333
186. Tuteja N, Singh MB, Misra MK, Bhalla PL, Tuteja R. Molecular mechanisms of DNA damage and repair: progress in plants. Crit Rev Biochem Mol Biol. (2001) 36:337–97. doi: 10.1080/20014091074219
187. Kurek K, Plitta-Michalak B, Ratajczak E. Reactive oxygen species as potential drivers of the seed aging process. Plants. (2019) 8:174. doi: 10.3390/plants8060174
188. Pandiselvam R, Mayookha VP, Kothakota A, Sharmila L, Ramesh SV, Bharathi CP, et al. Impact of ozone treatment on seed germination-a systematic review. Ozone Sci Eng. (2019) 42:1–16. doi: 10.1080/01919512.2019.1673697
189. Niemira BA. Cold plasma decontamination of foods. Annu Rev Food Sci Technol. (2012) 3:125–42. doi: 10.1146/annurev-food-022811-101132
Keywords: plasma, seeds, processing, mechanisms, germination, surface modification, stress or disease resistance
Citation: Waskow A, Howling A and Furno I (2021) Mechanisms of Plasma-Seed Treatments as a Potential Seed Processing Technology. Front. Phys. 9:617345. doi: 10.3389/fphy.2021.617345
Received: 14 October 2020; Accepted: 17 March 2021;
Published: 14 April 2021.
Edited by:
Mounir Laroussi, Old Dominion University, United StatesReviewed by:
Eugen Stamate, Technical University of Denmark, DenmarkCopyright © 2021 Waskow, Howling and Furno. This is an open-access article distributed under the terms of the Creative Commons Attribution License (CC BY). The use, distribution or reproduction in other forums is permitted, provided the original author(s) and the copyright owner(s) are credited and that the original publication in this journal is cited, in accordance with accepted academic practice. No use, distribution or reproduction is permitted which does not comply with these terms.
*Correspondence: Alexandra Waskow, YWxleGFuZHJhLndhc2tvd0BlcGZsLmNo
Disclaimer: All claims expressed in this article are solely those of the authors and do not necessarily represent those of their affiliated organizations, or those of the publisher, the editors and the reviewers. Any product that may be evaluated in this article or claim that may be made by its manufacturer is not guaranteed or endorsed by the publisher.
Research integrity at Frontiers
Learn more about the work of our research integrity team to safeguard the quality of each article we publish.