- 1Laboratory of Radiation Biology, Joint Institute for Nuclear Research, Dubna, Russia
- 2Faculty of Nuclear Sciences and Physical Engineering, Czech Technical University in Prague, Prague, Czech Republic
- 3Faculty of Natural and Engineering Science, Dubna State University, Dubna, Russia
The radiobiological effects of accelerated ions with high charge and high energy (HZE) on mammalian cells and their propagation in time are still not sufficiently explained and attract great deal of attention. This work aims to compare the immediate and delayed effects with emphasis on the latter. As shown by our group, the dependence of mutant fraction on expression time after irradiation may have interesting, non-monotonic, character depending on LET (linear energy transfer) of the used heavy ions. We speculate that this phenomenon may occur due to the induced genomic instability. Another area of our research is the study of the DNA structural changes in these mutants induced at different expression times. Chinese hamster V79 cells were irradiated with accelerated ions 11B, 18O, 20Ne, and gamma radiation. The LET was ranging from 0.23 keV/μm of 60Co gamma rays up to 136 keV/μm of 20Ne ions. DNA of unique HPRT mutants was isolated, concentration measured, HPRT exons amplified, and analyzed at several different time points, up to about 40 days, after exposure. Over 1200 HPRT mutants were analyzed for deletions of exons and sorted into three main categories: partial deletion, PD—with deletion of one to eight exons; total deletions, TD—with all nine exons deleted; and no deletions—no change in the HPRT structure observed. In general, the number of samples with partial deletion was increasing with LET of the used radiation, suggesting that higher energy deposition to the cell nucleus is more likely to cause larger structural changes. In the case of total deletions, increase in their number with LET was observed up to LET ∼115 keV/μm followed by a sharp decrease. The samples were also analyzed for the distribution of deletions, in particular exons at various expression times, the so-called mutational patterns. Hypothesis of the mechanisms behind observed phenomena is given, and possible implications for further research are discussed.
Introduction
Accelerated heavy ions (HZE: high Z, high energy) are in the focus of biophysical and radiobiological research fields for some time now. It is mainly due to two reasons: biological effects of ions in galactic cosmic rays (GCR) that will impact (to a higher extent) astronauts during the flight missions beyond the magnetosphere of Earth [1], and their medical use in radiation therapy of cancer which grows continually [2, 3]. The knowledge of biological effects of HZE ions is limited compared to sparsely ionizing radiation such as gamma rays or X-rays, particularly due to the limited availability of ion-capable accelerators usable for irradiation of biological samples.
Research focusing on the mutation induction, their type, and the radiation-induced genomic instability is significant for understanding of the long-term effects of radiation, probably more than the cell killing [4]. Only the surviving cells may form mutations that are considered as one of the triggers of carcinogenesis [4–8], which can be developed in later stages as a delayed effect of exposure [9]. The hypoxanthine–guanine phosphoribosyltransferase (HPRT) gene mutation assay is a well-known system for easy mutagenic studies, based on the 6-thioguanine (6-TG) mutant selection [10], which has been widely used to study the mutagenic effects of ionizing radiation (IR) of various qualities in mammalian cell cultures [11–16].
It has been shown that heavy ions with high LET (linear energy transfer) can cause biological damage fundamentally different from sparsely ionizing radiation—heavy ions are usually reported as being more effective in inducing various biological effects [12, 13, 17]. Their energy deposition within the track of their particles is more prone to create complex clustered lesions within DNA—containing single strand breaks as well as double strand breaks of DNA—that are extraordinarily difficult for the cells to repair and therefore susceptible to misrepair [18]. The literature data available for the quality of HPRT gene mutations induced by heavy accelerated ions are very scarce. A number of articles describe the mutational spectra induced by IR, which seems to be different in dependence on the type of radiation used: several about the effects of sparsely ionizing radiation (X-ray, gamma rays): [8, 11, 19–24]; a few using alpha particles (or He ions): [5, 11, 14, 20, 25, 26]; but only a couple exposing the cells to heavy charged ions: [8, 21, 27–29].
The published data seem to have a high degree of variety; nevertheless, some general conclusions can be drawn: radiation with higher LET (mainly alpha particles tested) usually caused more total deletions and lower number of partial deletions [19] or samples with no deletions [8, 20] in comparison to low LET radiation (gamma or X ray). However, studies showing the same or very similar levels of total deletions after irradiation with alpha particles or gamma radiation can be found as well [24, 26, 30]—a minority of authors even observed lower numbers of total deletions after irradiation with higher LET radiation [31]. Particularly for accelerated heavy ions, Baumstark-Khan et al. reported the number of total deletions of the HPRT gene increasing with LET of accelerated oxygen ions up to approximately 318 keV/μm followed by decrease, while the number of partial deletions was changing mildly in the same LET interval. It has been shown that even heavy ions can cause small alterations or point mutations [27], possibly due to the created secondary electrons. The results of HPRT gene molecular spectra induced by different ion species show high level of heterogeneity. It was reported that the deletion spectrum was nonspecific with regard to LET for mutants induced by neon ions, but LET specific for carbon-induced mutants [21]. It points to the fact that LET may not be the only parameter governing the biological effect, and that the mechanism behind it might be substantially more complex.
This work follows up our previous publication [32] that deals mainly with the effects of HZE ions on induction of HPRT mutants in prolonged expression times (ET). Surprisingly, it was found that the maximum of mutations was reached at different ET in dependence on LET of the used radiation. With increasing LET, the maximum was moving toward later ET. The current work focuses on structural analysis of these isolated mutants (on the level of exons of HPRT gene). This could give us answer about the type of mutations caused by different kinds of radiation and help to confirm our previous hypothesis that the delayed mutations are created de novo as a manifestation of genomic instability. This investigated topic, effects of prolonged expression times on the mutational spectra induced by radiation of various qualities, has been surprisingly little mentioned in the literature. The mutational patterns were usually studied in the first days after irradiation only and were not followed through in later ET. Usually, the mutants were chosen at a set ET [8, 28, 29], after particular number of population doublings [21], or similar. Therefore, the aim of this article was to bring some possible new insights into the development of HPRT mutation deletion spectra and to help to explain the connection between the late radiation effects and the quality of radiation.
Materials and Methods
Cell Culture Cultivation
Detailed information about the cell culture and cultivation procedures have been described earlier [32]. Briefly, V79 Chinese hamster lung fibroblasts culture (ECACC 86041102) was provided by the Institute of Cytology of the Russian Academy of Sciences. During standard cultivation and recultivations, the culture was grown in Dulbecco’s modified Eagle medium (DMEM; PanEco, C410p) supplemented with 10% of fetal bovine serum (FBS; PAA Laboratories, A15–011), 0.3 mg/ml l-glutamine (PanEco, F032), 100 units/ml penicillin, 100 μg/ml streptomycin, and 0.25 μg/ml amphotericin (Sigma-Aldrich, A5955) at 37°C, 95% humidity, and in 5% CO2:95% air atmosphere.
Irradiation Conditions
During all experiments (both with sparsely and densely ionizing radiations), exponentially growing cells were irradiated in suspension in the standard nutrient medium, and the cell concentration was kept constant at 106 cells/ml. Detailed description of the irradiation setups, beam characteristics, and dosimetry was already provided [32]. In short, gamma irradiation (applied for comparison with heavy ions irradiation) was delivered via the 60Co therapy unit “Rokus-M” of the Dzhelepov Laboratory of Nuclear Problems, Joint Institute for Nuclear Research (JINR), Dubna, Russia. Accelerated heavy ion irradiations were performed with the isochronous cyclotron U400M of the Flerov Laboratory of Nuclear Reactions, JINR. Cell samples were irradiated at the radiobiological facility Genome-M. The homogeneity of the beam (95% at the biological sample) and the scattered ions (less than 1%) were monitored by five ionization chambers [33]. Cell samples were kept at minimum thickness (iunder 2 mm) and irradiated in the plateau region of the Bragg curve to guarantee uniform irradiation. Ion energies when entering and leaving the cell suspension were calculated using LISE++ software [34], and LET within the sample was estimated using SRIM software [35] as the weighted average over the sample. Three different types of ions were used in this work: 11B, 18O, and 20Ne irradiation parameter details in Table 1.
Mutation Assay and Mutant Subclone Isolation
Work procedures with cells after irradiation have been explained in detail previously [32]. The whole experimental procedure is summarized in Figure 1. Briefly, cells were detached from the irradiation cylinders within 30 min after irradiation, pooled by absorbed dose, and seeded at appropriate concentrations in a standard cultivation medium in order for the mutations to be expressed (to Recultivation 0 in Figure 1). After reaching confluence of about 80–90%, part of the cells was used for recultivation under the same conditions, prolonging the time after irradiation for expression of mutations (expression time, ET). Other part of the cells was used for the HPRT mutation assay using the 6-thioguanine (6-TG; Sigma-Aldrich, A4882) selection agent. After 10–12 days of growth, random mutant colony was isolated from each flask carefully not to be contaminated with other colonies. These isolated HPRT mutant subclones were cultured and later used for DNA extraction and following procedures. Mutant subclones were isolated approximately every third recultivation (with higher frequency at the beginning) for up to 40 days after exposure (usually 12 recultivations). Nonirradiated cells were treated in the same manner to determine the levels of spontaneous mutants (SM) during the whole investigated period.
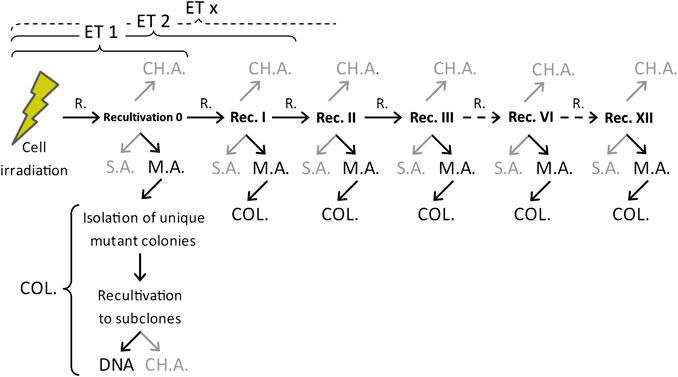
FIGURE 1. Scheme of the general experimental procedure after irradiation. In gray–methods and assays not directly covered by this work. R, recultivation; ET, expression time; M.A., mutation assay (6-thioguanine); S.A., survival assay; COL, isolation of individual mutant colonies + cell multiplication for use in other assays; DNA, analysis of DNA (isolation, concentration measurements, PCR amplifications, gel electrophoresis analysis, and evaluation); CH.A, chromosome aberration assay.
Hypoxanthine–Guanine Phosphoribosyltransferase Gene Structural Analysis
The cultured HPRT mutant subclones had their DNA isolated (not less than 106 cells per sample) using the DNK-EXTRAN-1 (Syntol, EX-509-100) kit. The DNA concentration was measured utilizing fluorescent double-stranded DNA selective Qubit dsDNA BR Assay Kit (Thermo Fisher, Q32853) on Qubit 2.0 Fluorometer (Thermo Fisher, Q32866).
Our structural analysis of the HPRT gene was based on the presence/absence of the exons. The HPRT gene consists of nine exons, and as a whole, it was sequenced by Rossiter et al. [36]. Each of the exons was amplified individually with the exception of exons 7 and 8 that are spatially close to each other and were amplified together as one amplicon. Schematic diagram of the exons, amplicons, and their sizes used in this work can be seen in Figure 2.

FIGURE 2. Diagram of the HPRT gene in V79 Chinese hamster cells with its exons and amplicons used in this study.
Used primers were based mainly on studies by Rossiter et al. [36] and Xu et al. [37] with the exception of the primers for exon 1 that were created de novo to comply with our PCR conditions. All the used primers for HPRT gene can be seen in Table 2, together with elongation factor two gene (EF) serving as control of the correctly performed PCR (all of the primers were assembled by the company Syntol). We used the approach where there is separate reaction for each exon (pair of primers). Each of the PCR tubes contained 40% of PCR mix (Syntol, M-439) with EVA Green intercalating fluorescent dye, each of the primers in concentration of 400 nM, DNA at 4 ng/μL of DNA, and deionized water up to the total volume of 25 μL.
PCR amplification was carried out in CFX96 Touch Real-Time PCR detection system (Bio-Rad, 1855195) with the usual five steps. Initial denaturation at 95°C for 180 s was followed by 35 cycles of annealing at 60°C for 45 s, elongation at 72°C for 30 s, and denaturation at 95°C for 20 s, with a final elongation at 72°C for 300 s. Afterward, a melting procedure was run; temperature was continually rising from 65 to 95°C in 0.4°C steps, with the “waiting time” of 5 s at each temperature point. Due to this approach, the fluorescent signal gives the information of presence/absence of the particular exon. Usually, this result was also confirmed on gel electrophoresis using 2% agarose gel (Helicon, AM-O710–0.5) dissolved in TAE buffer (Tris Acetate-EDTA buffer; 25x; PanEco, El040). Voltage was set to 5 V/cm of the distance between electrodes, and the power was turned on for 1 h at room temperature. The gel was stained with SYBR Safe (10,000x; Thermo Fisher, S33102) and documented on Gel Doc EZ (Bio-Rad, 170-8270).
Data Analysis
Our results, obtained by analyzing the samples for the presence or absence of the nine exons forming the HPRT gene, follow the Bernoulli distribution, where the variance can be calculated as
where p is the probability of samples having the deletion, and q is the probability of samples not having the deletion. Therefore, the standard error of the mean is
Important part of this article builds on our previous work [32]. Results from HPRT gene structural analysis are compared to the results of HPRT mutant induction with the same type of radiation. Different levels of induced mutants are described by mutant fraction (MF) that can be calculated as
where αM is the number of mutant colonies counted during mutation assay, βM is the number of cells seeded for the mutation assay, S is the cloning efficiency, αS is the number of colonies counted during survival assay, and βS is the number of cells seeded for the survival assay.
Calculations, mathematical operations, and data plotting were performed on the data in Microsoft Office 365 Excel and in SigmaPlot (v. 12.5, Sysat Software, Inc.).
Results
Induced Deletions in Dependence on Linear Energy Transfer of the Used Radiations
In the course of our experiments, DNA of over 1,200 unique HPRT mutants (irradiation induced and spontaneous) originating in a single cell was isolated and analyzed for HPRT gene exon deletions. Three main categories were distinguished (in accordance with the usual methodology): partial deletion, PD—with deletion of one to eight exons; total deletions, TD—with all nine exons deleted; and no deletions—no change in the HPRT structure observed.
Figure 3A shows a summarizing graph of all the samples analyzed with any deletion (partial or total) in relation to the ionizing radiation (IR) used for induction of mutations (error bars represent standard error of the mean of Bernoulli distribution). In order to see the general effect of used radiation, the doses and time points investigated, for a particular radiation, were averaged. The IR are ordered by increasing LET—from 0.23 keV/μm of gamma radiation [38] up to 136 keV/μm of Ne ions (details in Table 1). All of the radiation-induced mutants contained higher number of observable larger deletions than in the case of spontaneous mutants (SM; 10 ± 3.1%). It can be seen that, in general, the higher the LET, the higher the number of deletions (see also Table 3).
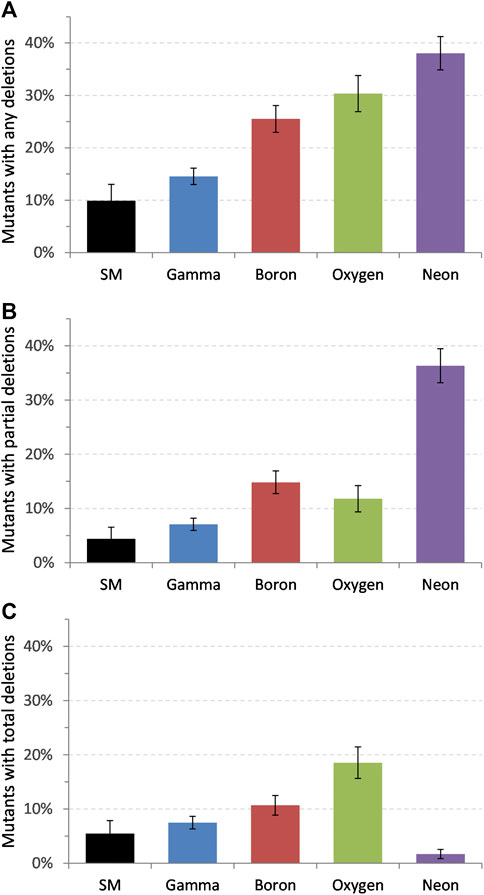
FIGURE 3. Distribution of deletions in HPRT gene for all samples (average of all the doses and time points assayed). (A) All deletions scored, (B) partial deletions, and (C) total deletions; SM, spontaneous mutants. Error bars represent standard error of the mean.
Figure 3B shows increase in the number of partial deletions with increasing LET of the incident radiation. Highest value, 36.3 ± 3.14%, was reached after irradiation with neon ions (LET ∼136 keV/μm), which is about eight times higher than that at SM (4.4 ± 2.1%). Analysis of the total deletions, where the whole HPRT gene was missing, shows a surprising phenomenon: increase in the number of total deletions with LET was observed up to oxygen ions (LET ∼115 keV/μm) followed by a sharp decrease at 20Ne ions (Figure 3C). The number of total deletions after irradiation with neon was extraordinarily low—only 4 out of 234 samples (1.7 ± 0.85%) had all the exons missing, which is lower than that at spontaneous mutants with 5.5 ± 2.4% of total deletions.
Deletions in Dependence on Expression Time
The mutant subclones for structural analysis of HPRT gene were collected in various times after irradiation (up to about 40 days since exposure) to see how the composition of deletions is changing during normal recultivation. No clear dependence on dose was observed, and the data points in Figure 4 represent the average of doses used with the specific type of radiation (details in Table 1). The gamma-irradiated samples (508 analyzed samples) for structural analysis were taken in the interval of expression times: 3–39 days. It can be seen in Figure 4A that the maximum of total deletions is reached soon after irradiation (about 6 days) followed by continuous decrease to the levels of spontaneous mutants. On the other hand, partial deletions are at their maximum cca 22 days after exposure and are only coming close to the background levels after around 40 days of cultivation. The data were also compared to our previous results focusing on the induction of mutations [32]. The mutant fraction (MF) is the ratio of the number of counted mutant colonies and the number of cells seeded, normalized to their cloning efficiency (details in Chapter: Data Analysis). Figure 4A shows this comparison of the averaged mutant fraction (MF) induced by gamma radiation at similar ET as in the case of the analysis of deletions. MF is monotonously declining from its maximum at the beginning, reaching SM levels around 20 days after. The survival of cells (their cloning efficiency) was measured at prolonged expression times as well (every time when the cells were assayed for mutations in HPRT gene) in order to correctly estimate the MF. In general, the spread in cloning efficiency was found to be about 20–30%, which is fairly typical for clonogenic measurements in V79 cell culture, without any obvious dependence on dose or time after irradiation.
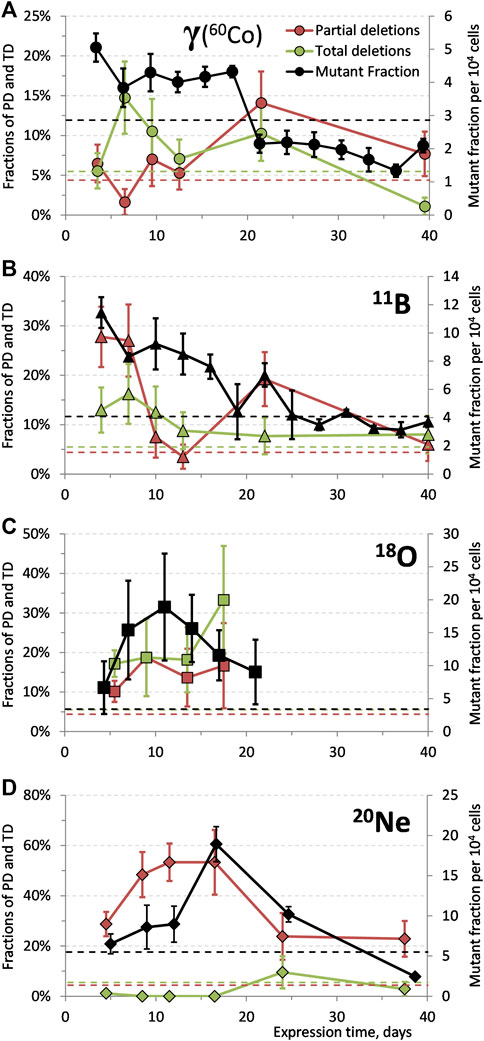
FIGURE 4. Dependences of fractions of partial and total deletions (average of used doses), and mutant fraction on expression time for HPRT mutants induced by (A) gamma radiation (60Co), (B)11B ions, (C)18O ions, and (D)20Ne ions. Dashed horizontal lines represent levels of spontaneous mutants: red line, partial deletions; green line, total deletions; black line, mutant fraction. The data points were connected to guide the eye. Data for mutant fraction were taken from our previous work [32]. Error bars represent standard error of the mean.
Partial deletions induced by boron ions (LET ∼49 keV/μm; 290 samples collected between 4 and 40 days after exposure), Figure 4B, are at their maximum in the first days after irradiation (4–7 days) with consequent decrease around 13 days, followed by second, local, maximum around 22 days after exposure before reaching the spontaneous mutants level at around 40 days. Initial rise in the number of TD is followed by decrease around 13–15 days after irradiation to the levels similar to SM. The MF is falling down continuously from its maximum shortly after irradiation, reaching background levels around 20–25 days postexposure and staying at this level.
In a tentative experiment with accelerated oxygen ions (178 samples), Figure 4C, around 72% of samples were isolated at the first time point after irradiation (5 days); therefore, the results from prolonged recultivation times have mainly informative character. The number of total deletions seems to be increasing toward 17 days since exposure, while PD stays at similar, slightly elevated, level. The estimated average of MF is peaking somewhere around 11 days after irradiation.
Extremely low levels of total deletions were observed during the whole observed period, between 4 and 38 days, after irradiation with neon ions, Figure 4D. Only 1.7% samples (4 out of 234) contained TD. On the other hand, partial deletions reached high levels (∼50%) within 9 days and remained there for approximately 8 days before decreasing. The average of PD stayed above the SM background even 38 days after irradiation.
Mutational Patterns
Mutational spectra of spontaneous as well as ionizing radiation-induced mutants (doses and time points pooled together) are depicted in Figure 5. Results of all SM samples (from all the experiments) show similar percentage of the particular missing exons from all the samples analyzed (between 5 and 9% of all the samples). Similar, uniform, distribution is found also at gamma-induced mutants; however, the absolute levels are higher ∼11–12%. Different character can be found after irradiation with boron and neon ions where particular exons exhibit significantly increased levels: exons 9 and 1 + 2 for 11B and 20Ne, respectively. Slightly elevated levels can be also seen after irradiation with oxygen ions at the first four exons.
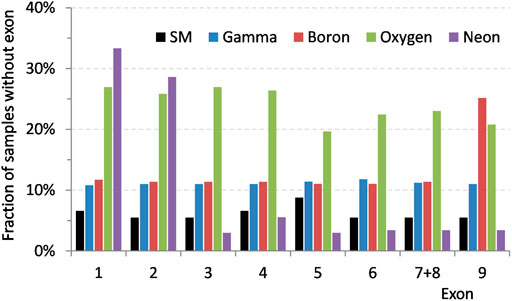
FIGURE 5. Mutational spectra of HPRT spontaneous and ionizing radiation-induced mutants (doses and time points pooled together for each particular radiation) showing percentages of all the samples with particular missing exon.
Closer look at the specific mutant deletions induced by 2 Gy of boron and neon ions, Figure 6, reveals a general trend: after an initial increase in the number of mutants with the aforementioned distinctive deletions, their number decreases (with the exception in the case of boron irradiation at 24 days where the number of mutants increases before decreasing again; it will need to be investigated if it is an example of non-monotonic dependence or an outlying value). Around 40 days after irradiation, the mutants with missing exons 1 and 2, in the case of neon irradiation, are almost completely mitigated. In mutants induced by boron ions, the number of samples with missing exon 9 is still around 15% after about 40 days.
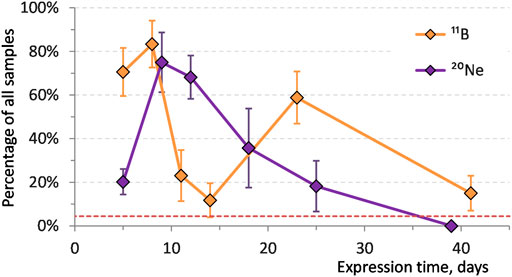
FIGURE 6. Distributions of the characteristic exons missing in HPRT mutants on expression time after irradiation by 2 Gy of 11B ions—exon 9 deletions and 20Ne ions—exons 1 and 2 deletions. Red dashed line represents partial deletions level of spontaneous mutants. Error bars represent standard error of the mean.
Non-contiguous Deletions
In the course of all the experiments, low number of noncontiguous deletions (NCD) was found—deletion of separate exons with a non-changed DNA between them [20, 28]. Their number is increasing with the LET of used radiation with the exception in irradiation with neon ions, Table 4; however, the number stays very low. Therefore, these results have so far been considered preliminary. No noncontiguous deletions were observed at spontaneous mutants.
Discussion
The number of deletions (both partial and total deletions added together = larger structural deletions) was increasing with increasing LET of the incident ionizing radiation, Figure 3A. It suggests that higher density of energy depostion in the cell nucleus is more likely to cause larger structural changes. The rest of the mutants (e.g., 90% for spontaneous mutants) contained mutations not detectable by our technique, likely point or very small deletions. Another explanation is the breakage within the intron region of HPRT gene and translocation of exon/s [20, 39], which would be also amplified correctly on PCR.
Similar growing trend can be seen also in the case of partial deletions, Figure 3B. It is worth mentioning that many different results are reported in the literature. For example, Suzuki et al. observed decrease in the number of partial deletions with increasing LET of C-ion beams (39–230 keV/μm) in the human embryonic fibroblast-like cells [29], but in the case of Ne-ion beams (63–335 keV/μm), the number of mutants with partial deletions stayed practically the same [21]. Our findings coincide with the observations of Jostes et al. (X-rays and alpha particles of 222Rn and its decay products) [30], Bao et al. (same as Jostes et al.) [26], or Baumstark-Khan et al. [8] who, as one of the few, actually studied the effects of accelerated heavy ions instead of alpha particles. Baumstark-Khan et al. observed increase in the number of PD with LET up to 250–300 keV/μm of oxygen ions followed by decrease with even higher LET.
The distribution of total deletions reveals significantly different character of dependence: a non-monotonic progression with maximum at 115 keV/μm (18O), Figure 3C. Radiation with the highest LET (20Ne) causes the lowest number of total deletions. Minimum (or in fact zero) total deletions were reported with a high LET radiation several times. Suzuki et al. observed the number of total deletions to decrease after irradiation with Ne-ion beams from 65% down to 30% at LET ∼63 and 335 keV/μm, respectively [21], and also to fall to 0% after exposure to C-ion beams (LET ∼230 keV/μm) [29]. Similar results were reached also in the case of Ni ions [5]. The most likely explanation of such phenomenon lies in the different energy deposition by different ion species due to the largely different track structure as suggested by other authors as well [8, 21, 25, 29]. Plausible hypothesis is that large clusters of energy from high LET radiations absorbed by DNA are causing large-scale deletions, making the cells more prone to cell death [12]. Only the created, secondary, lower LET electrons would be responsible for the induction of mutations [29], which could be a part of the explanation why we see high number of PD, but almost no TD after irradiation with high LET neon ions. Moreover, the high energetic high LET radiation may induce deletions not only in the HPRT gene but also in other vital genes necessary for survival [11]. As a result, the cell would be either dead or in serious proliferation disadvantage, not capable of reproduction and creation of mutant colony and therefore would not be observed, isolated, and analyzed. The effect of radiation of different LET on clonogenic survival immediately after irradiation was found as expected (e.g., refs. 13 and 40)—decreasing survival with increasing LET of radiation. Nevertheless, direct mechanism behind this is not known and needs to be studied further.
To the authors’ knowledge, no studies have directly focused on the effects of expression time on the mutation spectra in HPRT mutants induced by different types of radiation. We have studied the structural alterations in the HPRT gene in prolonged times after exposure together with induction of mutations. The observations were made up to approximately 70–80 cell generations after irradiation. We did not observe a clear general dependence on dose in the applied dose intervals (see Table 1), with a few special cases which will be mentioned. Therefore, for comprehensible comparison and in accordance with other authors [8, 21, 29], results from all the doses used were averaged.
In the case of gamma radiation Figure 4A, one of the aforementioned special cases of dose effect was observed at samples irradiated with 7 Gy that lead to the highest addition to the number of total deletions. No clear correlation of TD to dose for the lower doses used and no obvious dose dependence in the case of partial deletions were observed. It indicates that after irradiation with sparsely ionizing radiation, there has to be multiple hits within the studied region in order to cause larger deletions, and with increasing dose, the chance for multiple hits increases. This is possibly due to the repair processes and DSB mis-rejoining. Similar to us, Park et al. observed increase in the number of TD (from 21 to 39%) with dose (1–4 Gy) after gamma irradiation (60Co) in primary human skin fibroblasts [22], or Schwartz et al. (from 11 to 39%) who irradiated CHO-K1 cells with gamma rays of 137Cs with doses 0.5–6 Gy [41]. Other authors saw only negligible difference in the number of TD or PD with increasing dose after low LET irradiation [8, 11, 14]. The total deletions reach their maximum in about 7 days after irradiation—there is some time needed for them to be “expressed.” After 40 days since irradiation, the number of TD has fallen down under the level of deletions typical for spontaneous mutants. Mutant fraction follows similar trend with the main difference that the background level is reached already in about 20 days post-exposure. It seems that mutants with the gamma-induced TD are struggling during proliferation and are overgrown by healthy cells. The maximum of PD reached at about 20 days indicates the complexity of the mechanism of creation and genetic stability of deletions.
After irradiation with boron ions, we witnessed very surprising situation. The dose of 0.5 Gy did not induce any structural mutations at any of the times after irradiation. We tested a significant number of samples (101), analyzed in the same manner as all the rest of the radiation-induced mutant subclones, but we did not see a single deletion. In fact, this irradiation with the lowest tested dose of 11B ions (LET ∼49 keV/μm) caused disappearance of partial and total deletions that are normally present in the spontaneous mutants (around 10% of all deletions). At the moment, we have no explanation why this type of exposure should lead to lesser number of exon deletions than SM—this phenomenon needs to be investigated further. Some similarities can be found between the progression of TD and MF induced by boron ions (Figure 4B): maximum of both dependencies is close to the beginning of observations, followed by a decrease with time, and reaching plateau around 15 and 20 days for TD and MF, respectively. For the rest of the observed time period, both values stay in proximity of the SM level. A highly non-monotonic course of dependences obtained after irradiation with oxygen ions, and the lack of data in prolonged ET, did not allow an objective evaluation of these relations, Figure 4C. In the case of neon ions (Figure 4D), the PD levels, to certain degree, correlate with the MF levels—after the maximum at 17 days post-exposure, both values fall down to the vicinity of spontaneous mutants. In this case, PD significantly predominates over TD. These dependences, Figures 4A–D, show the importance of studying the structural changes in different times after irradiation. If only one time after irradiation is chosen, some potential information might be lost and incorrect results drawn.
It seems that the mutant fraction dependence on ET possibly approximately correlates with the total deletion dependence in case of gamma rays (60Co; LET ∼0.23 keV/μm) and with the partial deletion dependence of 20Ne ions (LET ∼136 keV/μm). This difference in trends suggests a presence of LET threshold (perhaps, there can be found a weak correlation between MF and both PD and TD after boron ions with “intermediate” LET of 49 keV/μm). This threshold would then relate to the different types of ion beams used for irradiation and the tracks created by them in the cell nuclei. There are differences in energies (possibly connected to the induction and range of delta electrons), track core and penumbra sizes [42], and differences in energy deposition in general that can lead to clustering of number of damaged sites in a small volume of DNA [12]. Signs of similar threshold were also seen in our previous work [32] when studying LET dependence of the mutant fraction maximum position. Significant increase in the expression time of the MF maximum was observed at cells irradiated with very high LET radiations (e.g., neon ions), not after exposure to sparsely ionizing radiation. This may point to another piece of evidence of the different mechanism of action of sparsely ionizing and densely ionizing radiations.
It is possible to theorize that the emergence of TD maximum could be a consequence of the increased number of mutations (higher MF), the TD maximum seems to appear after the MF maximum. One of the hypothetical explanations could be that the increased number of TD reflects the cell repair mechanisms and processes needed to cope with high number of mutations, and one of them could be the complete excision of the HPRT gene. The potential logic behind it would be that completely missing HPRT gene would not trigger the salvage pathway processes for purine synthesis. In this manner, the cell would not waste any energy and resources on non-working (or only half-working) gene, and constructed the purines de novo which would be, in this case, beneficial, as hypothesized also by Krasavin et al. [43]. Govorun et al. speculated that similar process could lead to a decrease in chromosomal instability with an increase of LET [44]. However, the total HPRT gene deletions can be parts of much larger deletions [11] affecting other, possibly vital, genes as well, so the mechanisms might, and probably will, be more complex.
Subclones with total deletions of HPRT gene are being removed with time after irradiation, with the exception at the very beginning, while the mutations are still being formed. This trend can probably be branded as general (found after irradiation with all of the radiations used); the fraction of mutants with total deletions is decreasing with increasing time since exposure and achieving levels typical for SM. It is plausible that a proliferating disadvantage of cells without HPRT exon plays an important role here, and the cells with total deletions are slowly overgrown by other cells.
Our results show the possible long-term effects of radiation. As mentioned in the paragraph above, total deletions have fallen to the SM levels within our observation period. This is mostly true also for partial deletions with the exception in the case of 20Ne-induced mutants (radiation with the highest LET used ∼136 keV/μm), where the levels of PD stayed elevated even 38 days after irradiation. This points to the radiobiological uncertainties of high LET radiations. Translation of in vitro results to higher organisms (or humans) is, of course, difficult, but it shows the necessity to continue in the research of radiobiological effects of HZE ions that can be meaningful for future space travel as well as heavy ion radiation therapy.
Several studies confirmed that ionizing radiation may induce point as well as deleterious mutations in radiation-induced HPRT mutants [11, 23, 45]. As it has been shown, the type and quantity of structural changes depends on the used radiation type and its parameters as well as on time after irradiation, Figures 3, 4. Study of the particular exons deleted shows that in case of spontaneous mutants, deletion patterns were stable across all the experiments and times of cultivation tested. Similar spectra of spontaneous mutants—mostly no large deletions or distinctive patterns—were observed by other authors as well [8, 11, 22, 24, 46]. However, conflicting reports regarding the SM can be found-some studies observed higher levels of partial [25, 47] or total deletions [26]; for example, Kiefer et al. [39] show that in their case, the mutational spectra of spontaneous mutants were changing from experiment to experiment (total deletions ranging from 4 up to 52%).
The characteristic mutational spectra of boron and neon ions, in contrast to uniform spectra of SM or gamma radiation, indicate, once again, a varied interaction of ionizing radiation of different quality, Figure 5. It might be explained by the different mechanisms of action of sparsely ionizing radiation, where gamma radiation transfers energy in many rather low-energetic events, while heavy ions create a few highly ionized tracks through the cell nucleus. Therefore, the damage by gamma rays will likely be more homogeneous than that by the HZE ions—fluence of the used gamma radiation (60Co) is two and three orders of magnitude higher than in the case of boron and neon ions, respectively (Table 1). This way, it might be possible for the heavy ions to cause heterogenous damage only in part of the genome. This effect seems to be dependent on the density of ionizations induced by the different types of ionizing radiation [11]. The typical deletion in the exon 9 after boron irradiation was produced almost solely by the dose of 2 Gy. In the case of neon ions, the distinctive spectrum (missing exons 1 and 2) was observed after both doses 0.5 and 2 Gy (to higher extent for the higher dose). As seen also for other endpoints (such as cell killing and induction of mutations), LET is not the sole parameter governing the final radiation effect [4, 13, 48] and the differences in track structure of high energetic particles may considerably affect the quality of mutations [8]. Except for our data supporting this hypothesis, the difference in mutation spectra based on the type of radiation used was documented by other authors as well: for carbon and neon ions of similar LET values [21, 28]. Radiations with high LET deposit large amount of energy in a small volume of DNA creating clusters of locally highly damaged DNA [12]; combination of such cluster damage and spatial distribution of the observed genetic material can substantially influence the mutational patterns. Moreover, strand breaks may occur not only as a consequence of direct IR damage, but also due to repair processes that are started as a result of a base change or damage [39, 49]; therefore, the mutational spectra may vary depending on the prevailing repair mechanism as well. So far, oxygen irradiation remains an open question from this point of view, where the orientation spectrum is relatively constant. This will need to be verified by further experiments.
The elimination of specific structural deletions with time, Figure 6, and the fact that both doses of high LET radiation (20Ne) caused the same type of deletion suggest that the mutants containing them are not duplicating at the same rate as healthy cells and also that the mutations are not clonally replicating. These results support the hypothesis in our previous article, stating that the delayed mutations are being created de novo due to sublethal damage caused by radiation and as manifestation of radiation-induced genomic instability. Even though we cannot reject the possibility of distribution of some of the mutations by cloning during normal cell replication, our results advocate that it should not be the reason for delayed maxima of mutant fraction as observed after high LET irradiation [32].
Noncontiguous deletions were found in all radiation-induced mutants. In general, their numbers were low—maximum of 2,8% in the case of irradiation with oxygen ions, Table 4. It has been mentioned that this type of deletions is also not induced by sparsely ionizing radiation (e.g., X-ray or gamma rays) [20, 21, 39]. We were able to detect one mutant with such a pattern after 60Co irradiation; however, the sample size was 508 gamma samples, which represents approximately 0.2%. It is therefore possible to find this type of deletion even after gamma irradiation, but the chances are at the level of error in measurement. Some authors consider the noncontiguous deletions as signature of high LET radiations [20, 39]. Due to the extremely low occurrence (even after HZE ion irradiation), and due to the fact that some studies did not observe any NCDs (e.g., 11), we do not consider the noncontiguous deletions as an usable tool for recognizing exposure to densely ionizing radiations.
Conclusion
It was shown that the fraction of exon partial deletions in HPRT gene was increasing with LET of the used radiation in the tested range from 0.23 keV/μm of gamma radiation to 136 keV/μm of 20Ne ions. Similarly, the total deletions were increasing with LET, with the exception at the highest LET radiation. It was suggested that total deletions are possibly the consequence of increased number of mutations and/or repair processes that are taking place at the same time. The number of mutants with TD is generally decreasing with expression time, reaching levels typical for SM maximally in about 30 days in all experiments—possibly due to their proliferative disadvantage. It has been shown how the proportions of deletions are changing in time after irradiation. This time dependence must be kept in mind if observed only in a single time point. Correlation between the MF dependence on ET and TD was found for sparsely ionizing radiation, while in the case of high LET neon ions, the MF correlated with PD. Distinctive mutational patterns were created by high LET radiations, contrary to sparsely ionizing radiation. We hypothesize that these LET effects are linked with differences in the tracks created by heavy ions and the spatial energy deposition within them. This could, as consequence, induce clustered DNA damage with various degrees of complexity. Lesions with different complexity may be repaired with different efficiency and also by different repair processes. Future research will be necessary to clarify the exact mechanisms behind the observed phenomena. It would be fruitful to test various heavy ions (in a wide interval of doses) and up to higher LET values.
Data Availability Statement
The raw data supporting the conclusions of this article will be made available by the authors, without undue reservation.
Author Contributions
IK, PB, NK, and RG designed the project. PB, IK, NK, YB, and DP were part of the team conducting experiments and preparing samples. IK and VM supervised the project. PB processed samples, analyzed data, and drafted the manuscript. PB, IK, NK, VM, and EK participated in the discussion and revision of the manuscript. All authors participated in the discussion of the data obtained. All authors read and approved the final manuscript.
Funding
This work has been carried out in the framework of financial topic 04-9-1077-2009/2023 JINR and supported by the grants of the Representative of the Czech Republic in the Committee of Plenipotentiaries of JINR and by the projects solved in cooperation between the Czech Republic and the JINR. This work has been cofunded by the project of Ministry of Education Youth and Sports, Czech Republic, project “Center for advanced applied science,” no. CZ.02.1.01/0.0/0.0/16_019/0000778.
Conflict of Interest
The authors declare that the research was conducted in the absence of any commercial or financial relationships that could be construed as a potential conflict of interest.
Acknowledgments
The authors thank Yu. G. Teterev and A. А. Bezbakh of the Flerov Laboratory of Nuclear Reactions (JINR), and G. N. Timoshenko and V. А. Krylov of the Laboratory of Radiation Biology (JINR) for their help with irradiation of our samples with accelerated heavy ions using the U400M cyclotron and V. N. Gaevsky of the Dzhelepov Laboratory of Nuclear Problems (JINR) for gamma irradiation at the Rokus-M unit.
References
1. Simpson JA. Elemental and isotopic composition of the galactic cosmic rays. Annu Rev Nucl Part Sci (1983) 33:323–82. doi:10.1146/annurev.ns.33.120183.001543
2. Ebner DK, Kamada T. The emerging role of carbon-ion radiotherapy. Front Oncol (2016) 6:6–11. doi:10.3389/fonc.2016.00140
3. Jermann M. Particle therapy statistics in 2014. Int J Part Ther (2015) 2:50–4. doi:10.14338/IJPT-15-00013
4. Stoll U, Barth B, Scheerer N, Schneider E, Kiefer J. HPRT mutations in V79 Chinese hamster cells induced by accelerated Ni, Au and Pb ions. Int J Radiat Biol (1996) 70:15–22. doi:10.1080/095530096145283
5. Kiefer J. Mutagenic effects of heavy charged particles. J Radiat Res (2002) 43:S21−S25. doi:10.1269/jrr.43.S21
6. Suzuki K, Ojima M, Kodama S, Watanabe M. Radiation-induced DNA damage and delayed induced genomic instability. Oncogene (2003) 22:6988–93. doi:10.1038/sj.onc.1206881
7. Smith LE, Nagar S, Kim GJ, Morgan WF. Radiation-induced genomic instability: radiation quality and dose response. Health Phys (2003) 85:23–9. doi:10.1097/00004032-200307000-00006
8. Baumstark-Khan C, Rosendahl IM, Rink H. On the quality of mutations in mammalian cells induced by high LET radiations. Adv Space Res (2007) 40:474–82. doi:10.1016/j.asr.2006.11.034
9. Allen CP, Hirakawa H, Nakajima NI, Moore S, Nie J, Sharma N, et al. Low- and high-LET ionizing radiation induces delayed homologous recombination that persists for two weeks before resolving. Radiat Res (2017) 188:82. doi:10.1667/RR14748.1
10. Thacker J, Stephens MA, Stretch A. Factors affecting the efficiency of purine analogues as selective agents for mutants of mammalian cells induced by ionising radiation. Mutat Res (1976) 35:465–78. doi:10.1016/0027-5107(76)90208-6
11. Rothkamm K, Gunasekara K, Warda SA, Krempler A, Löbrich M. Radiation-induced HPRT mutations resulting from misrejoined DNA double-strand breaks. Radiat Res (2008) 169:639–48. doi:10.1667/RR1185.1
12. Rosendahl IM, Baumstark-Khan C, Rink H. Mutation induction in mammalian cells by accelerated heavy ions. Adv Space Res (2005) 36:1701–9. doi:10.1016/j.asr.2005.03.067
13. Sørensen BS, Overgaard J, Bassler N. In vitro RBE-LET dependence for multiple particle types. Acta Oncol (2011) 50:757–62. doi:10.3109/0284186X.2011.582518
14. Little JB, Nagasawa H, Pfenning T, Vetrovs H. Radiation-induced genomic instability: delayed mutagenic and cytogenetic effects of X rays and alpha particles. Radiat Res (1997) 148:299–307. doi:10.2307/3579514
15. Stoll U, Schmidt A, Schneider E, Kiefer J. Killing and mutation of Chinese hamster V79 cells exposed to accelerated oxygen and neon ions. Radiat Res (1995) 142:288. doi:10.2307/3579138
16. Kumar PRV, Mohankumar MN, Hamza VZ, Jeevanram RK. Dose-rate effect on the induction of HPRT mutants in human G 0 lymphocytes exposed in vitro to gamma radiation. Radiat Res (2006) 165:43–50. doi:10.1667/RR-3467.1
17. Goodhead DT. Mechanisms for the biological effectiveness of high-LET radiations. J Radiat Res (1999) 40:1–13. doi:10.1269/jrr.40.S1
18. Nickoloff JA, Sharma N, Taylor L. Clustered DNA double-strand breaks: biological effects and relevance to cancer radiotherapy. Genes (2020) 11:99. doi:10.3390/genes11010099
19. Schwartz JL, Rotmensch J, Sun J, An J, Xu Z, Yu Y, et al. Multiplex polymerase chain reaction-based deletion analysis of spontaneous, gamma ray- and alpha-induced hprt mutants of CHO-K1 cells. Mutagenesis (1994) 9:537–40. doi:10.1093/mutage/9.6.537
20. Schmidt P, Kiefer J. Deletion-pattern analysis of α-particle and X-ray induced mutations at the HPRT locus of V79 Chinese hamster cells. Mutat Res (1998) 421:149–61. doi:10.1016/S0027-5107(98)00159-6
21. Suzuki M, Tsuruoka C, Kanai T, Kato T, Yatagai F, Watanabe M. Cellular and molecular effects for mutation induction in normal human cells irradiated with accelerated neon ions. Mutat Res (2006) 594:86–92. doi:10.1016/j.mrfmmm.2005.08.007
22. Park MS, Hanks T, Jaberaboansari A, Chen DJ. Molecular analysis of gamma-ray-induced mutations at the hprt locus in primary human skin fibroblasts by multiplex polymerase chain reaction. Radiat Res (1995) 141:11. doi:10.2307/3579084
23. Colussi N, van Leeuwen X, Lohman PH. Similar mutational spectra in the HPRT gene of human and hamster cell lines after exposure to either low dose rate or high dose rate X-rays. Mutat Res (1998) 401:89–97. doi:10.1016/S0027-5107(97)00318-7
24. Aghamohammadi SZ, Morris T, Stevens DL, Thacker J. Rapid screening for deletion mutations in the hprt gene using the polymerase chain reaction: X-ray and α-particle mutant spectra. Mutat Res (1992) 269:1–7. doi:10.1016/0027-5107(92)90155-U
25. Zhu LX, Waldren CA, Vannais D, Hei TK. Cellular and molecular analysis of mutagenesis induced by charged particles of defined linear energy transfer. Radiat Res (1996) 145:251. doi:10.2307/3578979
26. Bao C-Y, Ma A-H, Evans HH, Horng MF, Mencl J, Hui TE, et al. Molecular analysis of hypoxanthine phosphoribosyltransferase gene deletions induced by α- and X-radiation in human lymphoblastoid cells. Mutat Res (1995) 326:1–15. doi:10.1016/0027-5107(94)00152-U
27. Stoll U, Schneider E, Kranert T, Kiefer J. Induction of HPRT- mutants in Chinese hamster V79 cells after heavy ion exposure. Radiat Environ Biophys (1995) 34:91–4. doi:10.1007/BF01275212
28. Kagawa Y. Complex hprt deletion events are recovered after exposure of human lymphoblastoid cells to high-LET carbon and neon ion beams. Mutagenesis (1999) 14:199–205. doi:10.1093/mutage/14.2.199
29. Suzuki M, Watanabe M, Kanai T, Kase Y, Yatagai F, Kato T, et al. Let dependence of cell death, mutation induction and chromatin damage in human cells irradiated with accelerated carbon ions. Adv Space Res (1996) 18:127–36. doi:10.1016/0273-1177(95)00799-K
30. Jostes RF, Fleck EW, Morgan TL, Stiegler GL, Cross FT. Southern blot and polymerase chain reaction exon analyses of HPRT - mutations induced by radon and radon progeny. Radiat Res (1994) 137:371. doi:10.2307/3578712
31. Thacker J. The nature of mutants induced by ionising radiation in cultured hamster cells III. Molecular characterization of HPRT-deficient mutants induced by γ-rays or α-particles showing that the majority have deletions of all or part of the hprt gene. Mutat Res (1986) 160:267–75. doi:10.1016/0027-5107(86)90137-5
32. Bláha P, Koshlan NA, Koshlan IV, Petrova DV, Bogdanova YV, Govorun RD, et al. Delayed effects of accelerated heavy ions on the induction of HPRT mutations in V79 hamster cells. Mutat Res (2017) 803–805:35–41. doi:10.1016/j.mrfmmm.2017.08.004
33. Bezbakh AA, Zager VB, Kaminski G, Krylov AI, Krylov VA, Teterev YG, et al. Upgrading the genome facility for radiobiological experiments with heavy-ion beams. Phys Part Nucl Lett (2013) 10:175–8. doi:10.1134/S1547477113020039
34. Tarasov OB, Bazin D. LISE++: radioactive beam production with in-flight separators. Nucl Instrum Methods Phys Res Sect B Beam Interact Mater Atoms (2008) 266:4657–64. doi:10.1016/j.nimb.2008.05.110
35. Ziegler JF, Ziegler MD, Biersack JP. Srim–the stopping and range of ions in matter (2010). Nucl Instrum Methods Phys Res Sect B Beam Interact Mater Atoms (2010) 268:1818–23. doi:10.1016/j.nimb.2010.02.091
36. Rossiter BJF, Fuscoe JC, Muzny DM, Fox M, Caskey CT. The Chinese hamster HPRT gene: restriction map, sequence analysis, and multiplex PCR deletion screen. Genomics (1991) 9:247–56. doi:10.1016/0888-7543(91)90249-E
37. Xu Z, Yu Y, Gibbs RA, Thomas Caskey C, Hsie AW. Multiplex DNA amplification and solid-phase direct sequencing for mutation analysis at the hprt locus in Chinese hamster cells. Mutat Res (1993) 288:237–48. doi:10.1016/0027-5107(93)90090-3
38.Linear ICRU. Energy transfer journal of the international commission on radiation units and measurements (1970). Report 16. Available from: https://doi.org/10.1093/jicru/os9.1.Report16 (Accessed April 27, 2016).
39. Kiefer J, Schreiber A, Gutermuth F, Koch S, Schmidt P. Mutation induction by different types of radiation at the Hprt locus. Mutat Res (1999) 431:429–48. doi:10.1016/S0027-5107(99)00184-0
40. Barendsen GW. The relationships between RBE and LET for different types of lethal damage in mammalian cells: biophysical and molecular mechanisms. Radiat Res (1994) 139:257. doi:10.2307/3578823
41. Schwartz JL, Jordan R, Sun J, Ma H, Hsieb a W. Dose-dependent changes in the spectrum of mutations induced by ionizing radiation. Radiat Res (2000) 153:312–7. doi:10.1667/0033-7587(2000)153[0312:DDCITS]2.0.CO;2
42. Chatterjee A, Schaefer HJ. Microdosimetric structure of heavy ion tracks in tissue. Radiat Environ Biophys (1976) 13:215–27. doi:10.1007/BF01330766
43. Krasavin EA, Govorun RD, Shmakova NL, Koshlan IV, Nasonova EA, Repin MV. Genetical action of radiation with different physical characteristics on human and mammalian cells. Phys Part Nucl (2004) 35:1483–515
44. Govorun RD, Koshlan IV, Koshlan NA, Krasavin EA, Shmakova NL. Chromosome instability of HPRT-mutant subclones induced by ionising radiation of various LET. Adv Space Res (2002) 30:885–90. doi:10.1016/S0273-1177(02)00407-6
45. Fuscoe JC, Zimmerman LJ, Fekete A, Setzer RW, Rossiter BJF. Analysis of X-ray-induced HPRT mutations in CHO cells: insertion and deletions. Mutat Res (1992) 269:171–83. doi:10.1016/0027-5107(92)90198-B
46. Yamada Y, Park MS, Okinaka RT, Chen DJ. Molecular analysis and comparison of radiation-induced large deletions of the HPRT locus in primary human skin fibroblasts. Radiat Res (1996) 145:481. doi:10.2307/3579070
47. Suzuki K, Ojima M, Kodama S, Watanabe M. Delayed activation of DNA damage checkpoint and radiation-induced genomic instability. Mutat Res (2006) 597:73–7. doi:10.1016/j.mrfmmm.2005.04.024
48. Cucinotta FA, Wilson JW, Shavers MR, Katz R. Effects of track structure and cell inactivation on the calculation of heavy ion mutation rates in mammalian cells. Int J Radiat Biol (1996) 69:593–600. doi:10.1080/095530096145607
Keywords: accelerated heavy ions, V79 hamster cells, HPRT mutants, HPRT structural changes, delayed effects of radiation, genomic instability
Citation: Bláha P, Koshlan IV, Koshlan NA, Bogdanova YV, Petrova DV, Govorun RD, Múčka V and Krasavin EA (2021) Structural Changes in HPRT Gene of V79 Cells After Irradiation With Heavy Ions—Immediate and Delayed Effects. Front. Phys. 8:584326. doi: 10.3389/fphy.2020.584326
Received: 16 July 2020; Accepted: 16 November 2020;
Published: 18 January 2021.
Edited by:
Vincenzo Patera, Sapienza University of Rome, ItalyReviewed by:
Marie Dutreix, Institut Curie, FranceCharlot Vandevoorde, iThemba Laboratory, South Africa
Copyright © 2021 Bláha, Koshlan, Koshlan, Bogdanova, Petrova, Govorun, Múčka and Krasavin. This is an open-access article distributed under the terms of the Creative Commons Attribution License (CC BY). The use, distribution or reproduction in other forums is permitted, provided the original author(s) and the copyright owner(s) are credited and that the original publication in this journal is cited, in accordance with accepted academic practice. No use, distribution or reproduction is permitted which does not comply with these terms.
*Correspondence: Pavel Bláha, cGF2ZWwuYmxhaGF4QGdtYWlsLmNvbQ==