- 1INFN-Laboratori Nazionali del Gran Sasso, L’Aquila, Italy
- 2SNOLAB, Sudbury, ON, Canada
The study of rare fundamental physics phenomena, such as double-beta decay, rare nuclear decays and dark matter, requires very low levels of background radiation in order to observe a signal. To achieve the required background levels, experiments are located deep underground as these facilities provide significant rock overburden and commensurate reduction in the cosmic ray flux and cosmic ray-spallation induced products. An overview of the sources of these backgrounds will be presented. Taking advantage of the deep underground laboratory spaces, there have been a growing number of underground measurements in other fields, including environmental monitoring, benchmarking of other physical techniques, Life Science studies in low background environments, and material selection. The exceptional sensitivity and high resolution of high-purity germanium detectors allows for very sensitive measurements using gamma-ray spectrometry. Their use has been increasing as they allow for non-destructive measurements of experiment components, which can be directly used if they meet specified background requirements. This paper will discuss the current most sensitive ultra-low background germanium detectors in operation and explain how to achieve the best level of background reduction to attain the best sensitivities. In addition, an overview of several complementary low background measurement methods will be discussed. A proposed program to cross calibrate germanium detectors at several laboratories will be described and a searchable database used to store radioactivity measurements of experimental materials will be introduced.
Introduction
The study of radioactive backgrounds has been ongoing for several decades, it began in the 1950’s with the search for little-known backgrounds in materials surrounding particle accelerators to determine if those materials were activated from the accelerator itself. Counting facilities were then developed to measure the materials and then to search for lesser known backgrounds. This involved building dedicated facilities with the appropriate construction materials to shield the counters from local backgrounds. Once sufficient local backgrounds were removed from the counters, dedicated background experiments were gradually developed as the need arose. The counters were then developed for local use quantifying radionuclides in basic materials such as rock, concrete and steel. This led to a natural transition to low background material assays for nuclear and particle physics experiments as these became more sensitive to backgrounds from their construction materials. The initial counting facilities were usually developed by the individual experiments as their needs arose. Gradually these counters were developed into permanent facilities and improved to increase sensitivity (see for example Refs. 1 and 2).
It was realized that to increase sensitivity and to remove cosmogenic backgrounds, moving the background counters to underground laboratories would be very beneficial. For these early low background counting facilities shallow underground labs were developed with depths up to 100 m. As experiments required lower background materials to observe rare event searches, the materials to build the counter were improved and to further remove atmospheric backgrounds the counters were moved to deep underground laboratories. The search for low background materials continues with a particular emphasis on the search to find materials which have low concentrations of the radioactive chain elements,
The backgrounds of interest can include muons, alphas, betas, gammas or neutrons, depending upon the experiment and component in question (see Refs. 3 and 4). The radiation could be direct or via leaching or emanation, thus, several different detector technologies are used to estimate radioactive contamination levels. In addition, the counting detectors themselves must be very low in background contamination levels, which requires them to be radioactively clean and underground, and fabricated and shielded with low background materials. Several complementary methods are used to measure the backgrounds, which depend on the material, if the material itself is going to be used in the experiment or if some of the material can be sacrificed for the assay (see for example Ref. 5). These methods and their advantages and drawbacks will be discussed in Section 3.
Background Sources
The energy range of the expected signals in experiments searching for rare events is approximately 1–5 MeV, the same range as that of the decay of radionuclides and other nuclear reactions. The background induced by cosmic rays, especially muons, is diminished by going underground. The rock overburden reduces efficiently their intensity and their reaction products through interaction with matter (mainly neutrons). The muon flux at LNGS is for example suppressed by more than six orders of magnitude to less than 1 muon m
However, just having an experiment deep underground is not enough to reduce the backgrounds to levels required for the current generation of experiments. All materials contain traces of radioactive nuclides and the most important task is to bring down the intrinsic background of the detector set-up. In general, materials contain radioactive impurities because either they are not radio-pure or they come into contact with radio impurities during their production process. Other substances such as copper are intrinsically very radio-pure, but can contain radio impurities by being exposed to cosmic rays after manufacturing.
Primordial Radionuclides and Natural Decay Chains
All nuclides that still exist since they were produced in stellar nucleosynthesis are called primordial;
Cosmogenic Radionuclides
This group of radionuclides is produced through interaction with matter of secondary and tertiary cosmic ray particles [6]. For example
Anthropogenic Radionuclides
These are man-made radionuclides i.e., artificially produced through nuclear reactions either in nuclear power and reprocessing plants, nuclear weapons testing, and production of radioactive sources for industrial and medical applications. The radionuclides belonging to this group are for example
Radio-Assay Techniques
There are several different techniques available to assay materials. Each technique has its advantages and drawbacks. For example, some techniques require the sample to be destroyed while other techniques may only allow a small fraction of the material to be sampled. Table 1 lists the most common counting techniques used, the type of background each method is best suited for and the current sensitivity of each method. Several of these common counting techniques will be described in the following sections.
Gamma-Ray Spectrometry
Gamma-ray spectrometry (GRS) with high purity germanium (HPGe) detectors is an all-important tool for material screening in rare event experiments. In order to achieve the best sensitivity, the most important prerequisite for these detectors is that near the sample and inside the detector itself the radioactivity concentration is as low as possible. In comparison to other methods (e.g., mass spectrometry or neutron activation) GRS provides a more inclusive method of radionuclide detection. The information about all contributing gamma-ray emitting radionuclides is combined in a single energy spectrum. The excellent energy resolution of HPGe detectors allows for a highly efficient separation of different gamma-ray lines and for the best way to identify the various radionuclides present. One further very important advantage is that material screening can be performed non-destructively, and no elaborate sample treatment is needed.
As previously discussed, the primordial radionuclides
Ultra-Low Background (ULB) HPGe spectrometers are specially developed detectors that use carefully selected materials (e.g., very pure copper, not exposed or at least only very shortly exposed to cosmic rays, ultrapure aluminium) as construction materials and very low radioactivity containing electronic components inside the detector end cap close to the germanium crystal. When operated deep underground [13], or even at rather shallow depths but with efficient active shielding [14], ULB HPGe detectors can reach sensitivities on the order of
ICP-MS Mass Spectrometry
Inductively coupled plasma mass spectrometry (ICP-MS) is a type of mass spectrometry which uses inductively coupled plasma to ionize the sample [15, 16, 17, 18]. The sample is atomized creating atomic and small polyatomic ions, which can then be detected. It is often used to detect metals and some non-metals which are dissolved in liquids at very low concentrations. The ions are then separated on the basis of their mass-to-charge ratio and a mass spectrometer then detects the ion signal, which is proportional to the concentration of the isotope, for this it is a non radiometric technique as it does not look for radioactive decay in itself, but for the concentration of the radioactive isotope. The absolute concentration in the sample can be determined through calibrations with certified reference materials. ICP-MS screening is useful for small samples or when a piece of a large sample can be used. The sensitivity of current methods down to nBq per kg levels for U and Th can now be achieved, so this method is very important in finding low background materials as it is one of the most sensitive background detection method currently used [19, 20]. The main drawback of this method is the condition that the sample preparation is destructive and only small samples of larger materials can be measured.
Alpha Spectrometry
Many isotopes can decay via alpha particle emission, these alpha particles can then be detected and counted using alpha spectrometry to identify and quantify the isotope based on properties of the emitted alpha particle. Similar to gamma spectrometry, energy spectra are detected with high precision alpha detectors and electronics and analyzed to determine the nuclide. Samples are often measured following chemical separation to isolate the radionuclides of concern due to the complexity associated with correcting for interferences since alpha particle energies are often very close together. Alpha spectrometry is widely used in several applications, including: environmental radioactivity monitoring, health physics personnel monitoring, materials testing, geology and mineralogy, forensics and nuclear fuel processing, see for example Ref. 21.
Alpha counting is often used for material testing as a complementary method to gamma counting. Samples are generally machined into thin disks, or a liquid solution is placed on a metal disk and then allowed to dry to give a uniform coating. If the thickness of the layer or of the sample is too thick then the detected energy line spectra are broadened to lower energies, due to some of the energy of the alpha particles being lost during their interactions through the layer of active material [22]. The samples can be measured with various types of detectors, some of the most sensitive detectors currently used are ionization chambers with or without sensor wires. The current detectors can detect alphas over an energy range of 1 to 10 MeV with a sensitivity better than 100 nBq cm
A second method of alpha spectrometry is to use internal liquid scintillation counting, in which the sample is mixed with or dissolved into a scintillation cocktail. The light emissions are then counted using photomultipliers and the signal is then analyzed using a combination of pulse shape discrimination and coincidence counting for identifying alpha beta coincidence events. The sensitivity of this method is on the order of 1 mBq/kg for
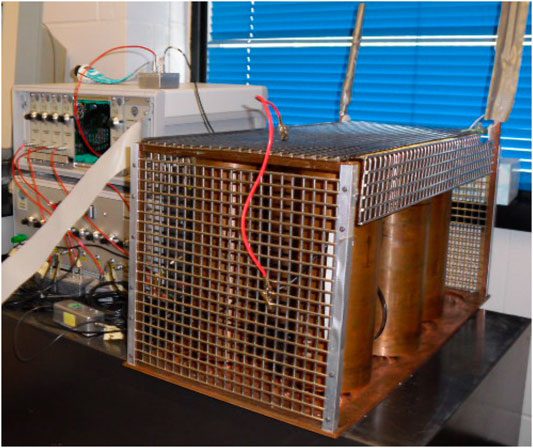
FIGURE 1. A photograph of a custom built alpha beta coincidence liquid scintillation detector located at SNOLAB.
Radon Assay Measurements
Radon emanation of a material is the process in which the radon atoms formed from the decay of
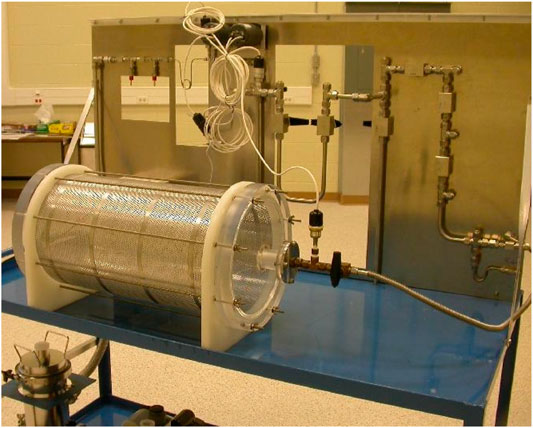
FIGURE 2. A photograph of a typical radon exhalation chamber at SNOLAB. The sample material is placed in the cylindrical chamber where the radon progeny are accumulated for several
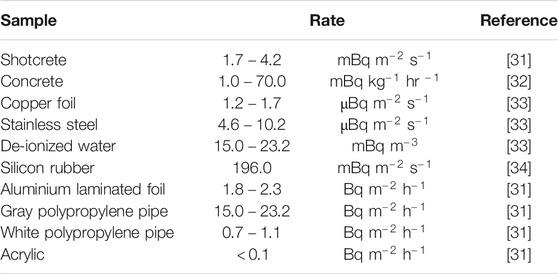
TABLE 2. Typical radon exhalation rates for some common construction materials used in underground experiments.
Neutron Activation
A sample which is not normally radioactive can be activated with neutrons causing its components to form radioactive isotopes which can then be detected using the methods described above. The process involves neutrons inducing radioactivity in the sample in which the nuclei capture free neutrons, thus becoming heavier and entering into excited states. The excited nucleus then decays by emitting gamma rays, beta and alpha particles, fission products and neutrons. The main drawback of this method is that the activation products could have long half-lives, thus making the sample unusable for the immediate deployment in an experiment. The main advantage of this method is that the sample usually does not need to be destroyed to complete the measurement, so this method is very useful if the activated isotopes have half-lives of at most a few days or months. This method is not used as much as in the past due the limited opportunities to irradiate samples as suitable activation reactors are in declining use. A very good overview on this method, its application in the field of rare events physics experiments and its actual sensitivity is given in Ref. 26.
Röntgen Excitation Analysis
Röntgen excitation analysis or X-ray fluorescence involves the emission of characteristic “secondary” (or fluorescent) X-rays from a material that has been excited by being bombarded with high-energy X-rays or gamma-rays. This method was first proposed by Glocker and Shreiber in 1928 [27]. This technique is used for elemental and chemical analysis in the composition of materials, such as metals, glass and ceramics, building materials, forensic science, archaeology and others. It can be used to determine U and Th in different matrices (see, e.g., Refs. 28, 29).
Germanium Detector Calibrations
Several underground laboratories operate germanium detectors which are used for low background counting. These detectors may be operated by the laboratories themselves or directly by various experiments. Each detector group has developed their own analysis method based on their experience with their particular detectors and analysis techniques. This makes it difficult to compare samples counted on different detectors without cross calibrations to take into account the different detector characteristics and analysis methods. Different research groups are currently taking a lead role in comparing as many germanium detectors as possible to determine the best detectors to measure the large number of samples expected to be counted to find the best possible materials to build their experiments.
The detector comparison is using calibration sources created from NIST and IAEA standards containing several isotopes in known quantities at very low levels. The sources are prepared in a container which can be fit most detectors to simplify the comparisons between the detectors. The detector groups will be sent the calibration sources without knowing the composition and will then report their results. The different groups will keep their results confidential until each laboratory has counted the calibration sources to avoid biases. The results from the different detectors will then be compared to determine the consistency of the results and if the measurements returned the expected concentration levels of the isotopes contained in the sources, this will allow the different analysis methods to be compared and to determine if there are any fundamental differences between the various analysis techniques. In addition, the detector sensitivities will be made available such that one can select which detector will be best suited for particular samples to make the best use of the detectors to count samples as efficiently as possible. For example, known high background materials should be counted on higher background detectors and materials expected to be low background should be counted on lower background detectors to maximize the number of samples counted.
Material Assay Database
The low background community has an ongoing effort to bring together all relevant low background counting results from around the world to create a central database. The database is a useful tool in tabulating data from many laboratories into one place so that those searching for new materials have a central repository to search for their materials. The database is currently hosted at SNOLAB and is maintained by an international collaboration of scientists who are responsible for developing the database application, defining the material assay data format and encouraging laboratories and experiments to contribute their data. The database is located at the following web site: www.radiopurity.org.
The project began at Lawrence Berkeley National Laboratory and has grown with the support of the wider low-background community, in particular the Assay and Acquisition of Radiopure Materials (AARM) collaboration and the ILIAS program which created a database of radio-pure materials from data collected at several European laboratories. The new database incorporated all of the data from the ILIAS database as its starting point and has continued to add new data from ongoing experiments. Currently, improvements are being planned to upgrade the software and databases to keep them current and to allow new data to be more easily added to keep the database relevant for the next generation of low background experiments.
Future Prospects
To further improve the low background detection counting techniques, the background levels of the existing counting systems must be reduced by at least an order of magnitude from current levels. To accomplish this large task, a program to find cleaner materials to build the counters themselves will be required. This includes, for example, finding the raw materials such as copper and lead with even lower internal backgrounds as these will be required to better shield the current suite of experiments.
One such proposed low background facility is being developed at SNOLAB which will includes its own supply of radon-reduced air to allow detector development in the underground lab. In addition it is being proposed to build a general purpose shielding tank with its own veto system to identify muon spallation events from cosmic rays. The tank would contain an inner acrylic tank and a photomultiplier array counter. Such a tank may prove critical to find cleaner materials for future experiment construction.
Summary
There are many different techniques employed to measure radioactive backgrounds, the techniques can depend on several factors, such as the sample size, whether or not the sample itself will be used in the final experiment, can the sample be sacrificed, etc. To fully understand a material background, often it can be counted using several techniques, where one can use germanium spectrometry to measure the bulk background of a material while using alpha spectrometry to measure the surface backgrounds. A program to cross calibrate low background detectors at several laboratories around the world is in progress which will allow a comparison of the different detectors and the different analysis techniques used by each experimental group. Finally, an improved database to collate the low background results for common materials will be further improved to enhance the user experience and to include data from many more underground laboratories.
Data Availability Statement
The original contributions presented in the study are included in the article/supplementary material, further inquiries can be directed to the corresponding author/s.
Author Contributions
ML and IL jointly contributed to this review of the low background radiation techniques by researching the topic and jointly writing and reviewing the text. IL created the tables and the figures.
Funding
SNOLAB operations are supported by the Canada Foundation for Innovation and the Province of Ontario, with underground access provided by Vale Canada Limited at the Creighton mine site.
Conflict of Interest
The authors declare that the research was conducted in the absence of any commercial or financial relationships that could be construed as a potential conflict of interest.
Acknowledgments
We would like to thank LNGS and SNOLAB and their staff for support through underground space, logistical and technical services.
References
1. Hult M, Preusse W, Gasparro J, Köhler M. Underground gamma-ray spectrometry. Acta Chim Slov (2006). 53:1–7.
2. Laubenstein M, Hult M, Gasparro J, Arnold D, Neumaier S, Heusser G, et al. Underground measurements of radioactivity. Appl Radiat Isot. (2004). 61:167–72. doi:10.1016/j.apradiso.2004.03.039
3. Formaggio JA, Martoff CJ. Backgrounds to sensitive experiments underground. Annu Rev Nucl Part Sci (2004). 54:361–412. doi:10.1146/annurev.nucl.54.070103.181248
4. Heusser G. Low-radioactivity background techniques. Annu Rev Nucl Part Sci. (1995). 45:543–90. doi:10.1146/annurev.ns.45.120195.002551
5.BOREXINO. Measurements of extremely low radioactivity levels in BOREXINO. Astropart Phys. (2002). 18:1–25. doi:10.1016/S0927-6505(01)00179-7
6. L’Annunziata M. Radiation physics and radionuclide decay In: M L’Annunziata, editor Handbook of radioactivity analysis. Amsterdam, Netherlands: Elsevier, Academic Press (2012). p 1–162.
7. Plant JA, Saunders AD. The radioactive earth. Radiat Protect Dosim. (1996). 68:25–36. doi:10.1093/oxfordjournals.rpd.a031847
8.UNSCEAR. Sources and effects of ionizing radiation. In United Nations Scientific Committee on the Effects of Atomic Radiation, , editor UNSCEAR 2008 report to the general assembly with scientific annexes, volume I: sources. New York, NY: United Nations (2008). p 1–463.
9. Pradler J, Singh B, Yavin I. On an unverified nuclear decay and its role in the dama experiment. Phys Lett B. (2013). 720:399–404. doi:10.1016/j.physletb.2013.02.033. |
10. Nisi S, Di Vacri A, Di Vacri ML, Stramenga A, Laubenstein M. Comparison of inductively coupled mass spectrometry and ultra low-level gamma-ray spectroscopy for ultra low background material selection. Appl Radiat Isot. (2009). 67:828–32. doi:10.1016/j.apradiso.2009.01.021.
11. Maneschg W, Laubenstein M, Budjáš D, Hampel W, Heusser G, Knöpfle KT, et al. Measurements of extremely low radioactivity levels in stainless steel for GERDA. Nucl Instrum Methods Phys Res Sect A Accel Spectrom Detect Assoc Equip. (2008). 593:448–53. doi:10.1016/j.nima.2008.05.036
12. Köhler M, Hult M, Arnold D, Laubenstein M, Reyss JL. Reference measurements of low levels of 60Co in steel. Appl Radiat Isot. (2004). 61:207–11. doi:10.1016/j.apradiso.2004.03.047
13. Heusser G, Laubenstein M, Neder N. Low-level germanium gamma-ray spectrometry at the μbq/kg level and future developments towards higher sensitivity In: P Povinec J Sanchez-Cabeza, editors Radionuclides in the environment. Amsterdam, Netherlands: Elsevier (2006). p. 495–510.
14. Heusser G, Weber M, Hakenmüller J, Laubenstein M, Lindner M, Maneschg W, et al. Giove: a new detector setup for high sensitivity germanium spectroscopy at shallow depth. Eur Phys J C. (2015). 75:531. doi:10.1140/epjc/s10052-015-3704-2
15. Gray AL, Date AR. Inductively coupled plasma source mass spectrometry using continuum flow ion extraction. Analyst (1983). 108:1033–50. doi:10.1039/AN9830801033
16. Houk RS. Mass spectrometry of inductively coupled plasmas. Anal Chem. (1986). 58:97A–105A. doi:10.1021/ac00292a003
17. Meermann B, Nischwitz V. ICP-MS for the analysis at the nanoscale–a tutorial review. J Anal At Spectrom. (2018). 33:1432–68. doi:10.1039/C8JA00037A
18. Tanner SD, Baranov VI, Bandura DR. Reaction cells and collision cells for icp-ms: A tutorial review. Spectrochim Acta B Atom Spectrosc. (2002). 57:1361–452. doi:10.1016/S0584-8547(02)00069-1. |
19. Dobson J, Ghag C, Manenti L. Ultra-low background mass spectrometry for rare-event searches. Nucl Instrum Methods Phys Res Sect A Accel Spectrom Detect Assoc Equip. (2018). 879:25–30. doi:10.1016/j.nima.2017.10.014
20. Povinec PP. New ultra-sensitive radioanalytical technologies for new science. J Radioanal Nucl Chem. (2018). 316:893–931. doi:10.1007/s10967-018-5787-3
21. Aggarwal SK. Alpha-particle spectrometry for the determination of alpha emitting isotopes in nuclear, environmental and biological samples: Past, present and future. Anal Methods. (2016). 8:5353–71. doi:10.1039/c6ay00920d
23.Mirion Technologies. Passivated implanted planar silicon (PIPS) detectors. Smyrna, GA: Mirion Technology Inc. (2020). Available at: https://www.mirion.com/products/passivated-implanted-planar-silicon-detectors (Accessed November 4, 2019).
24. Sakoda A, Ishimori Y. Mechanisms and modeling approaches of radon emanation for natural materials. Jpn J Health Phys. (2017). 52:296–306. doi:10.5453/jhps.52.296. |
25.Durridge. RAD7 radon detector user manual. Boston, MA: Durridge Company Inc. (2020). Available at: www.durridge.com/support/product-manuals
26. Clemenza M. Low background neutron activation: a high sensitivity technique for long-lived radionuclides determination in rare events physics experiments. J Radioanal Nucl Chem. (2018). 318:1765–72doi:10.1007/s10967-018-6333-z.
27. Glocker R, Schreiber H. Quantitative Röntgenspektralanalyse mit Kalterregung des Spektrums. Ann Phys. (1928). 390:1089–102. doi:10.1002/andp.19283900805
28. Kazy SK, D’Souza SF, Sar P. Uranium and thorium sequestration by a pseudomonas sp: Mechanism and chemical characterization. J Hazard Mater. (2009). 163:65–72. doi:10.1016/j.jhazmat.2008.06.076. |
29. Park JY, Lim JM, Ji YY, Lim CS, Jang BU, Chung KH, et al. Rapid screening of naturally occurring radioactive nuclides (238U,232Th) in raw materials and by-Products samples using XRF. J Radiat Prot Res. (2016). 41:359–67. doi:10.14407/jrpr.2016.41.4.359
30. Lucas HF. Improved low‐level alpha‐scintillation counter for radon. Rev Sci Instrum. (1957). 28:680–3. doi:10.1063/1.1715975
31. Bigu J, Hallman E. Radon emanation from shotcrete (1992). p. 1–2. SNO Technical Report SNO-STR-92-064.
32. Trevisi R, Leonardi F, Risica S, Nuccetelli C. Updated database on natural radioactivity in building materials in europe. J Environ Radioact. (2018). 187:90–105. doi:10.1016/j.jenvrad.2018.01.024.
33. Zuzel G, Simgen H. High sensitivity radon emanation measurements. In: D. Arnold, S. Jerome, and M. Hult, editors. 5th international conference on radionuclide metrology–low-level radioactivity measurement techniques ICRM-LLRMT’08; Elsevier Applied Radiation and Isotopes (2009). September 22–26, 2008. (Braunschweig, Germany). p. 889–93. doi:10.1016/j.apradiso.2009.01.052
34. Zuzel G. Highly sensitive measurements of 222-radon diffusion and emanation. In: R. Ford, B. Cleveland M Chen, editors Topical workshop on low radioactivity techniques: LRT 2004. AIP Conference Proceedings (2005). Vol. 785, p 142–9. Sudbury, Ontario, Canada, December 12–14, 2004. (Melville, New York). doi:10.1063/1.2060465
Keywords: gamma-ray spectrometry, ultra-low background, germanium detectors, alpha spectrometry, backgrounds
Citation: Laubenstein M and Lawson I (2020) Low Background Radiation Detection Techniques and Mitigation of Radioactive Backgrounds. Front. Phys. 8:577734. doi: 10.3389/fphy.2020.577734
Received: 01 July 2020; Accepted: 30 September 2020;
Published: 06 November 2020.
Edited by:
Maria Antonella Tabocchini, National Institute of Health (ISS), ItalyReviewed by:
Cristina Nuccetelli, National Institute of Health (ISS), ItalyJiri Hulka, National Radiation Protection Institute, Czechia
Copyright © 2020 Laubenstein and Lawson. This is an open-access article distributed under the terms of the Creative Commons Attribution License (CC BY). The use, distribution or reproduction in other forums is permitted, provided the original author(s) and the copyright owner(s) are credited and that the original publication in this journal is cited, in accordance with accepted academic practice. No use, distribution or reproduction is permitted which does not comply with these terms.
*Correspondence: Matthias Laubenstein, bWF0dGhpYXMubGF1YmVuc3RlaW5AbG5ncy5pbmZuLml0, Ian Lawson, bGF3c29uQHNub2xhYi5jYQ==