- 1Institut für Angewandte Physik und Messtechnik (LRT2), Universität der Bundeswehr München, Neubiberg, Germany
- 2Datzmann Interact & Innovate GmbH, Munich, Germany
The concept of spatial fractionation in radiotherapy was developed for better sparing of normal tissue in the entrance channel of radiation. Spatial fractionation utilizing proton minibeam radiotherapy (pMBRT) promises to be advantageous compared to X-ray minibeams due to higher dose conformity at the tumor. Preclinical in vivo experiments conducted with pMBRT in mouse ear models or in rat brains support the prospects, but the research about the radiobiological mechanisms and the search for adequate application parameters delivering the most beneficial minibeam therapy is still in its infancy. Concerning preclinical research, we consider glioma, non-small cell lung cancer and hepatocellular carcinoma as the most promising targets and propose investigating the effects on healthy tissue, especially neuronal cells and abdominal organs. The experimental setups for preclinical pMBRT used so far follow different technological approaches, and experience technical limitations when addressing the current questions in the field. We review the crucial physics parameters necessary for proton minibeam production and link them to the technological challenges to be solved for providing an optimal research environment. We consider focusing of pencil or planar minibeams in a scanning approach superior compared to collimation due to less beam halos, higher peak-to-valley dose ratios and higher achievable dose rates. A possible solution to serve such a focusing system with a high-quality proton beam at all relevant energies is identified to be a 3 GHz radio-frequency linear accelerator. We propose using a 16 MeV proton beam from an existing tandem accelerator injected into a linear post-accelerator, boosted up to 70 MeV, and finally delivered to an imaging and positioning end-station suitable for small animal irradiation. Ion-optical simulations show that this combination can generate focused proton minibeams with sizes down to 0.1 mm at 18 nA mean proton current - sufficient for all relevant preclinical experiments. This technology is expected to offer powerful and versatile tools for unleashing structured and advanced preclinical pMBRT studies at the limits and also has the potential to enable a next step into precision tumor therapy.
1 Introduction
Radiotherapy treatment of tumors is used in approximately 50% of all cancer cases worldwide and is therefore besides chemotherapy, surgery and immunotherapy one of the four pillars of cancer treatment throughout the last decades [1–4]. External beam and especially intensity modulated radiotherapy using X-rays, where radiation is applied from the outside of the body, is the standard procedure for performing state-of-the-art radiotherapy [4]. The damaging effects of radiation originate from the ionization of biochemical molecules and lead to the destruction of DNA in cells. Therefore, it is not only limited to cancerous tissue, but also normal tissue is affected by radiation. The unwanted side effects occurring in the normal tissue located in the beam path in front and behind the tumor is one of the main limiting factors for the dose which can be applied to the tumor within one fraction of radiotherapy. Modern therapy concepts aim to overcome the limits of radiotherapy and try to widen the therapeutic window, by either reducing the risks of side effects or by enhancing tumor control. One of these approaches is the radiotherapy with protons instead of X-rays, which was already introduced in 1946 [5]. When protons traverse matter, the dose is distributed following the Bragg curve, where the maximum of dose is deposited at the end of the particle range [6]. Due to this unique dose distribution, there is near-zero radiation applied behind the tumor. Additionally, the integral dose in the normal tissue in front of the tumor is reduced substantially compared to X-rays although still non-negligible. Together, proton therapy clearly widens the therapeutic window decreasing the risks of side effects and enhancing the potential of tumor control.
Originally, patient treatments with high energy protons typically have been carried out at large-scale research facilities such as iThemba Labs (formerly NAC) [7], Paul Scherrer Institute (PSI) (starting at 1996) [8] in Switzerland or Harvard Cyclotron Lab (starting at 1973) in the USA [9] and various others, by installing an extra treatment room besides several experimental target stations used for fundamental research in nuclear physics [10]. In the 1990s, first stand-alone centers have been built dedicated to treat cancer patients with proton therapy. One of the first was the cancer center in Loma Linda (USA) [11, 12]. With an increasing number of companies offering turn-key solutions for proton therapy systems (PTS), the number of proton therapy centers has increased rapidly since the beginning of the 21st century. In the following, the term “standard proton therapy” is used for this type of treatment modality.
Apart from using a different type of radiation other methods to reduce side effects have been proposed. Temporal fractionation has been established as the common solution to keep side effects in external radiation therapy under control [13]. One further approach in proton therapy is the reduction of the lateral penumbra by reducing the size of the pencil-beams in spot scanning therapy systems, which provides advantages in the treatment of shallowly situated tumors e.g. in children. A group at the St. Jude Children’s Research hospital (US) decreased the size of the beam at 221 MeV to 1.5 mm (σ) at a synchrotron-based facility compared to about 2.1 mm (σ) in the conventional mode of this system [14]. Another option is spatial fractionation that opens new possibilities. It was originally proposed in 1909 by Alban Köhler [15]. Here the use of metal grids in the beam path leads to high X-ray doses in the irradiated channels and low doses in the valleys shadowed by the grid. Thus, by sparing parts of normal tissue from radiation, side effects are reduced. Since then, several different approaches of spatial fractionation have been made. A meticulous overview is presented by Meyer et al. [16]. In the 1990s, the idea of spatial fractionation with X-rays was picked-up again and was coined microbeam radiotherapy (MRT) [17, 18]. Research in this field led to the development of dedicated beamlines at research facilities in Europe [17] and the USA [18], investigating the benefits and constraints of MRT for patient treatment in preclinical studies. Thereby, typically planar beams with beam sizes of 25–100 µm and an inter-beam distance of several hundred µm, with beam doses of several hundred Grays and valley doses of approx. 10–30 Gy are used [19–21]. Studies in rat and mouse brains lead to promising results, opening the possibility to push this method further to clinical trials [22–24]. Nevertheless, several problems remain. It is important to note that in MRT technique the tumor is irradiated with the same peak and valley pattern as the normal tissue. As the non-negligible doses in the valleys still damage normal cells this might limit the beneficial sparing effect, whereas the valley doses in the tumor might be too low to efficiently kill all cancer cells. In addition, going below the confidence interval of the prescribed tumor dose is not in accordance with the ICRU-requirements for dose homogeneity in tumor tissue [25]. The recommendations of the ICRU are well accepted in tumor therapy and act as a paradigm which has to be respected at all times during tumor therapy. But in some preclinical studies a better tumor control could be achieved using heterogeneous tumor doses in MRT [26, 27]. Additionally, simulation studies show better results when looking at the cell survival, when using heterogeneous tumor dose neglecting the upper bound of ICRU [28]. Therefore, using new therapy approaches fully exploiting the benefits of spatial fractionation could make it necessary to change the paradigm from the IRCU report 50 [25]. To do so precise and detailed preclinical research has to be performed. The major disadvantage of MRT is that this therapy approach uses X-rays, which still deposit a considerable amount of dose behind the tumor.
A method that combines both, the beneficial effects of proton radiotherapy and spatial fractionation, is proton minibeam radiotherapy (pMBRT), which was introduced in 2013 independently by two groups in France [29] and Germany [30]. In pMBRT the protons are applied like in MRT, but it can benefit from two big advantages. First, as explained above, the protons stop at the end of range and, therefore, no dose is deposited behind the tumor. Second, the angular straggling from multiple Coulomb scattering of the protons in the tissue causes a widening of the beams increasing with depth and a merging of distinct beams to a homogeneous dose distribution in the tumor, like in every conventional radiotherapy [31]. For achieving the same dose at any position in the tumor, as required in standard proton therapy, the total number of applied particles must be the same. The difference is that the protons are applied in sub-millimeter sized planar beams or pencil beam spots with center-to-center (ctc) distances in the millimeter range at the skin of the patient. In consequence, no or a low dose of radiation is applied in-between the minibeams. The ctc distances of the beams must be chosen in a way that the small angle scattering together with initial beam divergence leads to an overlapping of the beams and a quasi-homogeneous dose distribution already at the beginning of the tumor when considering unidirectional irradiation schemes [32]. Interlaced minibeams from two or more directions and heterogeneous tumor dose distributions are an additional option to increase sparing of healthy tissue up to the close tumor vicinity. The dose profiles of minibeam arrays may be chosen as steep as possible resulting in a high ratio between peak and valley doses (peak-to-valley dose ratio, PVDR), i.e., high doses in the minibeams and lowest possible doses in the valleys. With a high PVDR, the cells in the beams are in general killed due to the high doses, but the cells in the valleys get low doses that most normal cells survive without severe damage. Healthy cells in large valleys probably offer repair options by their proliferation and migration capabilities when replacing eliminated cells within the minibeam irradiated healthy tissue [32]. Although the basic principles are not yet known it is assumed that the so-called “dose-volume effect” [33, 34] and the “microscopic prompt tissue repair effect” [35, 36] play a major role in the healing of healthy tissue in pMBRT.
Preclinical studies conducted at the ion microprobe SNAKE (Superconducting Nanoprobe for Applied nuclear (Kern-)physics Experiments) [37, 38] in a mouse ear model showed that acute side effects in normal tissue are reduced by using pMBRT with PVDR = 540 compared to quasi homogeneous irradiation (PVDR 1–1.2) when pencil spot beams are applied with sizes in the range of 0.1–1 mm, a ctc distance of 1.8 mm, and a mean dose of 60 Gy [31, 39]. Side effects are negligible when beam sizes are small compared to the ctc distance. They steadily increase but are still lower than for homogeneous irradiation when decreasing the PVDR down to PVDR = 2.7 due to larger, Gaussian shaped beam sizes. Irradiations by individual X-ray pencil minibeams showed, in addition, the importance of minibeam sizes that allow for their efficient repair. While beam diameters smaller 2 mm showed nearly no side effects, a strong but gradual increase of the side effects was obtained for larger beam diameters at 60 Gy plateau dose [40].
Preclinical studies from Prezado et al. with rats showed also substantial reduction of side effects after proton minibeam irradiation of the brain with planar beams at a minibeam width of 1.1 mm and ctc of 3.2 mm (PVDR of ∼6.5) compared to homogeneous irradiation at 25 Gy mean dose [41]. Additional experiments exploiting the minibeam effect on tumor control showed that at least the same tumor control in high-grade gliomas in rats was achieved whereas survival after treatment was increased to 67% compared to 22% after homogeneous irradiation [22, 24]. The most recent study shows that cognitive function and also emotional and motor processes are mainly conserved after pMBRT [42]. Also, Dilmanian et al. have proven the technical feasibility of pMBRT at the MD Anderson Proton Therapy Center (Houston, Texas, US) [36]. Eley et al. recently used the same facility to perform a study of neurologic toxicity in a proton minibeam irradiation experiment with mice [43]. One specific approach is reported by a group from Krakow that performed first dosimetric characterization of mesh-formed collimators that are supposed to spare the eye lid during proton therapy treatment of uveal melanomas [44]. Overall, it can be concluded that pMBRT shows great potential in reducing side effects in front and behind the tumor while keeping tumor control.
Up to now although pMBRT is applicable to various kinds of tumors and therefore affects a lot of different tissues the preclinical testing at the moment is limited to studies either showing side effects in mouse ears and rat brains or only treating rat brain tumors. Effects of minibeams to various, more complex tissues such as lung, liver, heart, muscles or nervous tissue are not yet known. Furthermore, the effectiveness of pMBRT in various tumor types has also not been studied until now. Further research on these two topics is of urgent need to fully foresee the possible benefits and limits of this new type of therapy and to be able to bring it into clinics. The two main research facilities conducting pMBRT experiments in Germany and France are very specialized for the experiments on mouse ears (Germany) and rat brain (France) [32]. To be able to study various tumor types and the effects on different kinds of healthy tissue within a systematic roadmap to translate this method into clinical treatments, we believe it is necessary to build a dedicated preclinical pMBRT facility, which has also been suggested by Meyer et al. [16].
In this article, we will discuss the technical parameters which are of crucial importance for developing a preclinical small animal irradiation facility that is feasible to answer the most important questions in pMBRT. The minibeam sizes, the dose rate at the target and the energy range play an important role and are depending on the used technology related to each other. Based on existing standard proton therapy technology and methods, it will be evaluated which accelerator type and beam application method provides the best opportunities for a preclinical proton minibeam facility. The gained knowledge and the experience from the field of X-ray MRT and proton minibeam research will be reassessed for its applicability to the powerful and versatile preclinical testing facility. It will be discussed whether radio-frequency linear accelerators (RF-LINACs), which are currently being developed for standard proton therapy, can be a promising approach to fulfill the technical requirements. We will further present our idea of a research facility, capable of fully covering the preclinical experiments which are essential to prove the concept of pMBRT right and be able to bring it into clinic. This will be accompanied by our thoughts on which questions have to be answered and which kinds of tumors are best suited to be treated by pMBRT and should, therefore, be included in preclinical studies.
2 Physics Requirements for Proton Minibeam Radiotherapy
In the following, we will review and discuss the necessary parameters that are needed for designing a preclinical proton minibeam facility. This is based on the experience gained from the research performed on pMBRT so far as well as on the technological progress made in standard PTS serving in today’s clinical treatment centers.
2.1 Beam Application in Standard Proton Therapy
Concerning the acceleration of the protons to clinically relevant energies two types of accelerators have become established in the market of PTS: cyclotrons and synchrotrons. Synchrotrons are typically the first choice if a therapy center wants to use heavier ions such as carbon for therapeutic purposes in addition to protons. In almost all other cases where only protons are applied, cyclotrons are state-of-the-art. According to the PTCOG website [45], more than 95 particle therapy centers are reported to be in clinical operation at the beginning of 2020. In Europe, more than 80% of them are equipped with cyclotrons and less than 20% with synchrotrons [45].
Once accelerated, the particles are guided by magnetic fields and transported to the treatment rooms. Therein, a proton beam is shaped and measured by several devices in the nozzle, so that it can finally be applied to the target in the isocenter. Passive scattering for generating a homogeneous dose distribution in a field was the technology used in the early days of standard proton therapy [46]. For delivering a better conformal dose to the shape of the tumor, patient-specific apertures made from brass were used, while the energy was varied by range modulator wheels [47]. The PSI started to develop a new and more sophisticated beam application method called spot scanning or pencil beam scanning [8, 48, 49]. Thereby, the proton beam is magnetically focused to form a spot in the isocenter with a lateral Gaussian distribution and a size around 4–8 mm, which is increasing with decreasing beam energy [50, 51]. This pencil beam is deflected with fast dipole magnets in two perpendicular directions (x- and y-direction), producing a dose pattern that corresponds to the shape of the tumor in the beam’s eye view [52]. Compared to the simpler method of passive scattering, the spot scanning approach is able to achieve a better three dimensional dose conformity to the shape of the tumor, sparing additional healthy tissue from undesired dose [8, 53–55]. Moreover, handling of heavy patient-specific apertures at the beam nozzle–in the case of the passive scattering technology–is eliminated, saving a lot of time and costs in the treatment routine. Furthermore, a potential source of failure by using the wrong aperture is eliminated. As a consequence, almost 100% of the new PTS installations nowadays rely on the pencil beam scanning technology [45].
2.2 Minibeam Irradiation Methods –Collimation Versus Focusing
Originally, in the GRID X-ray therapy, standard metal grids with a few millimeter thickness were used that have been directly attached to the skin of the patient to laterally shape the beam in millimeter dimensions [15]. For X-ray planar microbeams and submillimeter minibeams in the 20–500 keV regime, multi-slit collimators have been manufactured with beam widths in the order of 25–75 µm or up to 0.7 mm [17, 56, 57].
When moving from X-rays to protons in early 2010s it was clear that µm-sized beams would not be needed since protons spread in tissue quickly to several 100 µm. However, in order to obtain submillimeter proton beams, the continuation of using collimators to form the proton minibeams seemed to be the logic consequence. Although proton pencil beams were available in several PT-systems the minimum sizes of a Gaussian shaped proton beam were several millimeters (σ). This was approx. one order of magnitude too large for spatial fractionation as considered in pMBRT. Therefore, the research groups actively working in the field of MRT needed to reassess the topic of beam collimation completely. In the case of clinical proton therapy, several centimeters thick metal collimators are required to safely stop the protons with therapy relevant energies. Extensive simulations were performed to optimize several parameters like material and thickness for the collimator [36, 58]. Manufacturing slits or holes of a few hundred µm width or diameter in collimators with several centimeters thickness is very challenging [51]. In complex Monte Carlo-simulations the characteristic parameters – slit width and center-to-center distance – and its influence on the dose distribution in the target were investigated [51, 59, 60]. Peucelle et al. manufactured collimators for pMBRT applications and tested them at an existing proton therapy facility [60]. They used a proton beam of 100 MeV penetrating a multislit collimator with 400 µm wide slits and a thickness of 50 mm to generate a planar minibeam. In 1 cm depth of tissue they measured a beam width of 1.1 ± 0.05 mm (fwhm) and a PVDR value of about 6.5 [60]. While all above mentioned studies investigated planar collimator designs, the following works analyzed collimators generating mesh- or grid-like minibeam patterns [44, 61, 62].
In most of these studies maximizing of the PVDR in the tissue proximal to the tumor was the major goal. However, large PVDR ratios cannot be produced either in planar minibeam cases and even worse in pencil beam minibeam cases due to scattering of the protons at the walls of the channels and penetrating portions at the collimator edges. Although PVDRs are not much larger than 15 at the entrance to the patient and decrease quickly, Prezado et al. showed that it is possible to adapt a clinical proton therapy facility for successfully performing preclinical pMBRT experiments [22, 24, 42] via using collimators with a planar multislit design. In a recent work from Lansonneur et al. a 6.5 cm brass collimator is used for a first theoretical study on clinical relevant proton minibeam treatment plans [59].
On the other hand, protons being charged particles leave the option of being focused to beam spots or lines by electromagnetic lenses which is not easily done with X-rays. This method has the potential to form minibeams with PVDRs of 100–10,000 [31, 63, 64]. The SNAKE group began simultaneously but independently from the group of Prezado to perform preclinical experiments in pMBRT, but utilizing focused 20 MeV proton beams. Instead of using a clinical PTS they utilized a proton microbeam facility and applied focused submillimeter proton pencil beams in a scanning mode to mice ears. With this method a matrix of 4 × 4 spots spread to Gaussian-like spot sizes of 0.09 mm (σ) or larger have been applied [31]. A recent work of Schneider et al. extends this idea to larger beam energies presenting extensive simulations of magnetically focused proton minibeams at a clinical proton center at the clinically relevant energies and proposes an adapted and optimized nozzle system [63]. Simulations result in PVDR values up to 1,000 at the entrance of the target.
In the following, several advantages and disadvantages of both methods for producing proton minibeams based on recent research in the field of pMBRT are presented:
(1) Collimation of the proton beam is a passive beam shaping method that unavoidably leads to a large decrease of the beam current at the target. This is independent whether the collimator is illuminated completely by a broad homogeneous dose profile or is scanned by a pencil beam scanning over the entire area of slits. When considering a ratio of irradiated to non-irradiated area of 1 : 100 as already performed in preclinical experiments using a magnetically focused pencil minibeam [31, 39, 65], the beam current at the target would be reduced at least by a factor of 100 when using a collimator. If the beam current upstream of the collimator cannot be increased, the application would take at least 100 times longer for delivering the same dose to the tumor compared to focused pencil minibeams. Even when considering planar minibeams, as shown in a recent study, the use of a multislit collimator results in a huge reduction in dose rate at the Bragg peak maximum of a 123 MeV proton beam from 2.6 Gy/s (broad beam without collimator) to 0.12 Gy/s (multislit) or even 0.09 Gy/s (SOBP condition) [51].
(2) The scattering of the proton beam at the edges of the slits (or holes) leads to enhanced lateral spreading of the proton beam adding to an unavoidable beam halo that enhances the valley dose [51, 66]. Thus, the PVDR is much lower in collimated minibeam systems compared to magnetically focused minibeams. The preclinical experiments from Sammer et al. revealed measured PVDR values larger than 540:1 for the smallest spot size used in mouse ear experiments [31]. Schneider et al. concluded that the dose simulations showed PVDR >50 in all investigated focused proton minibeam cases [63]. According to their work this is at least a factor of three more than feasible with mechanical collimators. Additionally, corresponding to the high aspect ratio, e.g., 0.4 mm (opening) to 65 mm (thickness), the slit geometry works as a divergence aperture. It cuts parts of the beam due to its intrinsic divergence. DeMarzi et al. [51] simulated this effect and showed that an 0.1° divergent collimator can theoretically mitigate this issue. However, the production and the alignment of divergent collimators to the beam would be a challenge in practical use.
(3) Preclinical simulations showed evidence that circular minibeam spots are superior to planar beams when irradiating from one side [67]. However, initial beam currents are even more reduced (quadratic reduction) when small bore holes in a grid pattern are considered compared to slits. Both geometries are acting as a collimation tool. Simulations using grids with submillimeter sized holes like 0.6 × 0.6 mm2 or even 0.05 × 0.05 mm2 indicate that not only the beam current is reduced but also the proton depth dose distribution along the beam axis is severely distorted. Martínez-Rovira et al. conclude a strong reduction in range of 105 MeV protons with these small grid sizes [62].
(4) The use of collimators for blocking high energy protons produces additional radiation due to nuclear reactions of the protons in the collimator. This leads to secondary radiation such as gamma-rays and neutrons [63] and lower energy protons, which unavoidably hit the patient. This will give an additional unwanted whole-body neutron dose to the patient [55, 68] and increase the risk of late side effects or secondary tumor induction. However, Guardiola et al. have calculated this unwanted additional biologic neutron dose in the patient to be less than 1% of the total absorbed dose [58]. On the other hand, due to the 4π characteristic of the neutron emission this dose is distributed to the whole patient. Further studies, e.g., using mesh-shaped pinhole instead of multislit collimators and performing measurements of the neutron doses would be helpful to assess the associated risks.
(5) There is a high effort for modeling the nozzle to simulate the correct depth dose distribution downstream of the interaction with the collimator. Especially the interaction at the edges of the slits has a considerable impact on the valley doses [51]. Moreover, experiments and simulations revealed that the lateral dose distribution downstream from the collimator is strongly dependent on the length of the air-gap between the collimator and the target [58]. All effects have to be modeled and implemented thoroughly in a treatment planning system for calculating the correct dose deposition in the target [69]. A recent study from Lansonneur et al. performed first theoretic investigations of therapy treatment plans with collimated minibeams and developed therefore a dedicated dose engine on the basis of TOPAS/Geant4 [59].
All of these mentioned issued do either not exist or have only minor implications if a magnetically focused beams are used as minibeam application method. Nevertheless, there are also advantages for using beam collimation:
(6) Placing a collimator in a fixed holder at the nozzle in front of the patient and illuminating it with a broad homogeneous beam is, from a technical point of view, easier to accomplish compared to focusing a hundred MeV proton beam to submillimeter spots or lines and scanning them.
(7) The collimator setup requires much less space than a magnetic focusing unit for pencil minibeams and can, therefore, be more easily integrated into rotating gantry setups at a standard PTS as demonstrated by DeMarzi et al. However, one suggestion to integrate a magnetically focused minibeam setup into a clinical proton gantry has been designed and presented by Schneider et al. [63]. They concluded that the current pencil beam scanning nozzle is not suitable for proton minibeam generation. Though, with a substantial nozzle redesign they proposed that beam spots with 0.66–1.67 mm (fwhm) are feasible at energies of 100 and 200 MeV. Beam currents are not specified, which makes it difficult to compare the benefit in the achievable dose rates at the target with the case of the collimated beams. Another possible solution for a focusing unit described in Section 3 has a length of 6 m [64]. This is too long to be integrated in a gantry nozzle, but an integration in a fixed beam nozzle of a standard PTS seems to be possible.
This study has the primary goal to evaluate the best suited technology for building a dedicated preclinical irradiation facility for proton minibeam application. To sum up the discussion so far, we conclude that for this purpose magnetically focused pencil minibeams are superior for the following reasons. This application method can provide the largest PVDR values and has therefore the highest potential for sparing healthy tissue. Especially in the testing and evaluation phase of preclinical experiments focused and scanned minibeams have a huge flexibility in producing different beam sizes, patterns and shapes as well as seamlessly variable ctc distances, and the resulting dose distributions can be modeled faster and easier. Furthermore, focusing and scanning minibeams is the only irradiation method feasible of providing intensity-modulated radiation therapy in all three dimensions [63]. Additionally, they ensure the lowest possible secondary radiation contribution. Last but not least, compared to collimation the focused minibeams offer the highest possible dose rates at the target. In particular the latter topic is an ongoing trend in the radiation oncology community for three reasons: the mitigation of moving targets [70–73], the exploration of hypofractionation [74–76] and the potential of reduced normal tissue toxicity due to the FLASH-effect at ultra-high dose rate [32, 77]. The topic of dose rate i.e. beam current will be elaborated in more detail in the next section. The preference for focused and scanned minibeams is supported also by other researchers in the field [16, 63]. Nevertheless, the technical layout of the nozzle in a preclinical facility should have the possibility to implement a collimator as well, for further evaluation of the individual advantages of both application methods.
In the process of the transition from preclinical experiments with tiny targets to clinical patient treatments with large tumors, the question of the best fitted beam application method probably has to be reassessed. Once the technical and bio-medical parameters are investigated more in-depth, it might be found out that the collimation method is a reasonable alternative for certain indications. On the other hand, the acceptance in the proton therapy community for going back to passive beam application technology with all its listed drawbacks might be very low. As nowadays proton pencil beam scanning is the state-of-the-art method in standard proton therapy the step forward to using submillimeter beams for spatial fractionation to spare healthy tissue seems feasible. The current requirements on scanning velocity, position accuracy and beam deflection are almost equal to those needed for pMBRT. Finally, the choice may also partly depend on the effort to integrate a minibeam focusing and scanning unit into a PTS nozzle, e.g., as suggested by Schneider and co-workers [63]. Another approach is based on a completely different accelerator technology [64], as used today for proton therapy, and will be discussed further in this work.
2.3 Beam Parameters for a Preclinical Facility
For the assessment of a suitable accelerator technology for producing proton minibeams within the scope of a preclinical irradiation facility, the relevant technical parameters have to be defined in a first step. These are the beam energy range, proton beam current (i.e., dose rate) at the target and beam spot size.
Considering beam energy, the existing proton therapy centers were reevaluated. The proton beam energy needed for patient treatment in clinics is typically defined to range between 70 and 230 MeV [46], sometimes up to 250 MeV [78], leading to projected ranges of protons in water between 4.1 and 33 cm [79] in water equivalent. However, for preclinical experiments with small animals such as mice or rats the energies have to be scaled down according to the animals’ size. A maximum energy of 70 MeV protons would be acceptable in a first step, as the bodies of these animals can be penetrated using this energy. But it has to be considered that in the case of small animal irradiation it is crucial to decrease the energy down to approximately 35 MeV (corresponding to a range in water of 1.2 cm [79]) in best case without the use of a range shifter. Otherwise the propagation of the minibeam size in dependence of the depth in the tissue will be substantially influenced due to the additional lateral straggling in the range shifter material.
The required beam current at the isocenter was originally determined by the maximum time tolerated for irradiating a tumor with one fraction of the dose. In this case, a general accepted rule has been established in particle therapy, that the irradiation of a tumor with a dose of 2 Gy should not last longer than 1 min [46]. In the passive beam application mode with double scattering the beam current at the target is reduced by a factor of up to five [46]. In the case of pencil beam scanning typically nearly 100% of the beam that reaches the nozzle is transported to the target. The back-calculation from a required dose rate to the necessary beam current at the nozzle depends on different additional factors such as beam diameter, scanning velocity and the time required for switching the beam energy. Thus, the application time strongly depends on the tumor volume. In the proton therapy community, an average beam current of 1 nA or a few nA at the target has been established to fulfill the mentioned dose application requirement for normal tumor volumes as used in classical X-ray fractionation schemes [46, 53, 80]. Therefore, we conclude that 1 nA proton beam current at the target should be considered as a lower limit for a preclinical as well as clinical facility.
Of course, when performing preclinical experiments, one typically deals with small tumor sizes. On the other hand, most of the small animal experiments have been conducted in single dose fractionation schemes (hypofractionation) with doses in the range of 25–60 Gy. In addition, heavily discussed challenges in radiotherapy like moving targets as well as modern therapy approaches in particular FLASH therapy require beam currents that are orders of magnitudes higher. For using the advantages of the FLASH effect, a dose rate of at least 40 Gy/s is necessary [77]. Looking at dose rates as specified by vendors for standard PTSs with pencil beam mode one finds values of 100 Gy/min (corresponding to 1.7 Gy/s) [81], which is still a factor of 23 below the lower limit set by Favaudon et al. [77]. It is certainly favorable for a preclinical facility to be able to vary the beam current over many orders of magnitude.
The specification of the required beam spot sizes for minibeam applications can for obvious reasons not be derived from standard proton therapy. The question of an optimal minibeam spot size of pencil minibeams has been addressed in two preclinical experiments [31, 40]. Besides experimental data, Sammer et al. also simulated the effects of pMBRT using dose profiles and corresponding cell survival using parameters α and β from LQ model provided by the PIDE platform [31]. Here beam spots and σ/ctc values are different to the measured ones. We think this is due to possible limitations regarding the choice of cell line and the corresponding α and β parameters. We therefore refer only to the measured beam sizes and σ/ctc here. The first experiment used single spot irradiation of mouse ears with beam sizes varying from 0.5 to 6 mm (fwhm) using X-rays at a small animal radiation research platform [40], which have comparable biological effects as proton irradiation, as shown before [39]. The smallest beam sizes of single pencil beam spots (0.5 and 1 mm diameter of pencil X-ray beams) showed no side effects, while for bigger beam sizes starting from 2 mm, side effects were increasing linearly with increasing beam sizes [40]. This result of the in vivo mouse ear study defines an upper limit of single spot irradiation with no side effects in the outermost tissues where the beams have not widened much. Another experiment was performed applying 20 MeV proton beam spots in a 4 × 4 matrix of 1.8 mm spacing at the ion microprobe SNAKE [31]. In this study the size of the Gaussian-shaped spots varied between 0.224 mm fwhm (σ = 0.095 mm) and 2.075 mm fwhm (σ = 0.883 mm). The experiment showed in both examined end points – maximum ear thickness and scoring of desquamation and erythema – the smaller the beam size the better the tissue has been spared, and the fewer side effects have shown up. The smallest spot size (σ = 0.095 mm) led to almost no side effects and therefore showed a considerable reduction compared to the second smallest (σ = 0.2 mm). Consequently, the experimental part of this study requests a beam size of σ < 0.1 mm for side effect free treatment in the superficial tissues of small animals. Slightly larger beam spot sizes might be acceptable when larger ctc’s are applied in the clinics, which show no side effects. Whether these size limits will be the same in other organs or in human tissue is to be addressed in further experiments when considering pMBRT. But beam sizes in the range of σ∼0.1 mm are requested in a preclinical facility to serve all necessary parameters for radiobiological studies in small animals.
In the case of clinical radiotherapy, the dose needed for tumor control cannot be applied in a single fraction in most of the cases. Therefore, fractionated therapy schemes are standard in conventional radio-oncology. Considering the topic in pMBRT, performing (temporal) fractionated therapy adds another complex parameter in the case of applying submillimeter beams on a day-to-day basis. This issue was already addressed in another preclinical study within the mouse ear model [65]. Here the authors wanted to investigate whether in each (temporal) fraction the very same beam spot locations have to be hit or if the exact spot position on the skin in each fraction is irrelevant. Again, the 4 × 4 beam geometry was applied using beams with a σ of 0.222 mm to the mouse ear model. The irradiation was performed in four fractions with 30 Gy each. The results revealed that the group where each spot was hit repeatedly at the same position within an accuracy of ∼0.5 σ (in this case corresponding to ≈0.1 mm accuracy) showed substantially less acute side effects compared to the irradiation where in each fraction deliberately different regions were hit and also compared to the positive control irradiation. In total, in a future preclinical facility, similar studies on optimum beam sizes and on the combination of spatial and temporal fractionation requires beam sizes in the range of σ∼0.1 mm and also an imaging and repositioning accuracy of 0.1 mm at proton energies of up to 70 MeV.
2.4 Achieving the Requirements
2.4.1 Review of Facilities in Operation
Up to now, the two European groups leading the field of preclinical pMBRT research used existing facilities and experimental setups with appropriate adaption to perform the first irradiation experiments by proton minibeams on small animals. However, both attempts are limited with respect to the important technical parameters discussed in the previous section. In the case of SNAKE, the beam sizes are well below the 0.1 mm limit and adjustable to wider beam spots by either beam scanning of a 1 µm focused beam or by passive beam spreading within a thin aluminum sheet. In addition, it has proven to deliver dose rates in a wide range from 0.01 Gy/s to about 1,000 Gy/s. But the proton energy is too low, as the tandem Van-de-Graaff accelerator, at which SNAKE is installed, delivers protons only up to an energy of about 25 MeV [82]. This corresponds to a water equivalent range of 0.64 cm [79] and thus is not sufficient for the treatment of tumors in deeper organs in mice or rats.
The French group has chosen another approach by using an existing clinical proton therapy facility based on a cyclotron accelerator at the Institute Curie-Centre de Protontherapie d’Orsay for first preclinical pMBRT experiments [22, 24, 41]. This strategy makes sense for two reasons: First, this solution allows direct access to an existing clinical system, with the opportunity to use the whole infrastructure of a clinical institute and a certified medical device. Second, it would certainly help gaining acceptance in the community if existing clinical treatment facilities can use their PTS with a possible technical upgrade also for pMBRT and thereby spread the new treatment modality widely. As mentioned above, about 80% of the PTS in Europe use cyclotrons.
Prezado et al. have already demonstrated that normal rat brains can be irradiated with planar proton minibeams produced through a collimator. A PVDR ∼10 was obtained with all drawbacks of creating minibeams by collimation as discussed above [60]. However, they could show reduced side effects in the brain compared to homogeneous irradiation. This study has been performed at 100 MeV leading to a shoot through the head of the rat, which is reasonable when only studying side effects in normal tissue. For delivering treatment doses to tumor tissue in small animals, proton energies at and below 70 MeV are needed. This ensures the maximum range of the protons is lower than the size of the animal and a tumor can be treated with full use of the Bragg peak. This can either be achieved by degrading the energy of protons coming from a clinical accelerator or by using an especially designed accelerator facility. Advantages and disadvantages of both methods are described below.
The lowest possible energy, without additional range shifters, at clinical cyclotron facilities is typically 70 MeV [46, 83]. This low energy limit of therapy cyclotrons originates from the process of energy variation and the requirement for a certain beam current at the isocenter. These cyclotrons extract the proton beam at a fixed maximum energy of about 230 MeV at maximum proton beam currents between 300 and 800 nA [78]. The emittance of the extracted beam is in the order of 5 π mm mrad [84] to 10 π mm mrad (root mean square, unnormalized) [85]. For achieving the clinically relevant energies the beam is decelerated by a degrader unit consisting of carbon wedges that are inserted in the beam path. This process completely destroys the phase space of the beam in the transverse and longitudinal dimension [86]. For transporting the beam further to the isocenter, it must be drastically cut by apertures to stay within the acceptance of the beam transport system (transverse phase space). Furthermore, an achromatic section has to be added to cut the broadened energy distribution, keeping the energy spread below a certain limit (dE/E ± 0.7%) [83] so that the Bragg peak is not smeared out too much compared to a monoenergetic beam. This is assuring a reasonable range accuracy of the proton beam within ±1 mm [85]. Both destructive beam-shaping methods lead to the fact that the transmission of the protons through the energy selection system (ESS) drops rapidly with decreasing energy. Already at about 200 MeV, the transmission is below 10%, at 70 MeV it is below 0.2% [80, 84]. Together with the maximum beam current at the extraction of the cyclotron this results in maximum beam currents of 1–2 nA at the isocenter for 70 MeV protons [78, 80]. Due to this effect, the lower limit of the proton beam current is already reached and there is no more space for further cutting the beam.
For translating the beam current into a dose rate the study of DeMarzi et al. is helpful [51]. Although they do not specify the beam current at the nozzle, it can be expected that it is in the order of a few nanoampere due to the transmission at a proton energy of 123 MeV. DeMarzi has measured a dose rate of 2.6 Gy/s at the target generated at the Bragg peak of a 123 MeV in the middle of proton pencil beam field of 5 × 5 cm2. Using the multislit collimator for minibeam widths of 1.1 mm at the depth of a rat brain, the dose rate was reduced to 0.12 Gy/s (multislit) or even 0.09 Gy/s (for SOBP condition). In consequence, the dose rate using a multislit collimator is reduced by at least a factor of 23. For reaching the lowest dose rate where FLASH effects are expected (40 Gy/s) an enhancement factor of more than 300 is necessary. Going to energies of 70 MeV and below the situation worsens due to the rapid decline in transmission.
The question is whether focusing the beam to minibeams in this scenario is an option for keeping the dose rate at least at the level of a few Gy/s and to obtain large PVDR ratios. The smallest beam size achievable at standard cyclotron-based PTS at the isocenter at an energy of 100 MeV is approximately between 5 mm [87] and 7.5 mm (σ) [69]. A demagnification of the lateral beam size with a magnetically focusing unit for example from 6 to 0.1 mm (σ) in beam size in one direction requires a demagnification factor of 60. This would imply a ratio of object distance to image distance of 60 : 1. It is unrealistic to achieve this in particular in a gantry nozzle due to three reasons: The total length in a gantry nozzle for a focusing unit is limited to about 3 m. Concerning the high demagnification factor, this would results in a distance of about 5 cm between the last magnet and the focal plane. Neither a strong enough focusing lens exists for this short focal length nor is it possible for animal treatments to have so little room for positioning and other devices such as an exit window. Additionally, one would obtain an inherent increase of the beam divergence by a factor of 60 resulting in lower PVDRs already when entering the tissue.
For further considerations, the parametrization of the Orsay gantry universal pencil beam scanning nozzle as presented by DeMarzi et al. [69] has been used as a starting point for estimation of the beam current in a minibeam resulting from a theoretical magnetic focusing unit, being well aware that there is not enough space for such a setup in a gantry. A beam spot size of 11 mm (σ) at a mean proton energy of 100 MeV and a beam divergence of 3.3 mrad (σ) at the nozzle entrance derived from [69] was used. A 5 m drift distance followed by a triplet of quadrupole magnets was simulated, which creates the focus after 0.6 m drift. The particle tracking code TRAVEL [88] is used to determine the detailed particle distributions at the beam focus for 106 protons, Gaussian distributed in the given initial longitudinal and transverse phase space. The resulting particle distribution at the focus was cut in x-y-space with a virtual aperture (radius of 0.3 mm). The transmission of protons (from the 106 protons) into this circle is 3.3% for the 100 MeV beam. Thus, the beam has to be cut by apertures to form a proton minibeam of that size without halo. The maximum current obtainable is estimated as follows: A maximum beam current of 800 nA is assumed for common cyclotron types [78]. Due to the degrader it can be expected that the beam current is reduced to 1% for 100 MeV resulting in maximal 8 nA behind the degrader and subsequently 0.27 nA (3.3%) for a spot size 0.3 mm (radius). Beam current might be even less since a lens that accepts the large divergence may be not technically feasible. In addition, the focused minibeam would have a divergence of about 30 mrad that would result in a larger beam spreading behind the beam focus than from multiple scattering. In the case of 70 MeV protons, the beam current would further reduce by a factor of approximately five due to the reduced transmission at the degrader.
In summary, using therapy cyclotrons for achieving very small spot sizes together with a high beam current for achieving high dose rate seems not possible. The situation becomes even worse when going to lower energies as needed for preclinical animal irradiation, because the lower the energy the lower the available beam current at the target due to the rapidly decreasing transmission trough the ESS. Therefore, we conclude that considering a preclinical irradiation facility for pMBRT research, proton therapy cyclotrons are not well suited because they do not provide the versatility to perform research exploring the entire parameter space for dose rate, minibeam size and energy.
To the authors’ knowledge, there is not a single proton therapy facility in operation worldwide that achieves 0.1 mm sized proton pencil spots fulfilling the requirements for energy and beam current as derived in the last chapter.
Nevertheless, it could be possible to use other types of cyclotrons with energies suited for preclinical needs. For instance, cyclotrons that are feasible of producing proton currents up to 500 μA at an output energy of about 70 MeV [89] or sector cyclotrons, also delivering 70 MeV at high beam quality [90]. These cyclotrons could be adapted to meet the requirements. Focusing a minibeam from the high current cyclotron could lead to a radical cropping of the beam by apertures to generate the required emittance but keeping tens of nA in tiny beam spots. As a consequence, a collimation process is needed but could be performed with a radiation shielding positioned far away upstream of the target in order to avoid parasitic irradiation from the large amount of secondary radiation. In 2020, several low energy cyclotrons are in clinical operation for eye tumor treatment. Among these are the cyclotrons in Clatterbridge [91], Nice [92] and Berlin [90] providing maximum energies of 62–68 MeV. For assessing the applicability of using these low energy cyclotrons to provide protons for a preclinical proton minibeam facility, a detailed study has to be conducted, which was beyond of the scope of our consideration here.
2.4.2 Radio-Frequency LINAC Concepts
Instead, a completely different particle accelerator type for PTS came into focus in the past years, since this technology has some unique physical characteristics that are complementary to a cyclotron [93]. Already in 1991, Hamm et al. suggested a compact, low current, normal conducting proton linear accelerator (LINAC) based on 3 GHz side-coupled structures for standard proton therapy [94]. The same technological principle is used in every modern clinical LINAC for X-ray radiotherapy of cancer patients in hospitals around the world. The only fundamental difference is that in this case electrons are accelerated to generate high energy (6–18 MeV) X-rays, enabling these LINACs to be built compact and light-weighted for mounting them on a small rotating gantry [95]. However, for the purpose of proton therapy the LINAC systems are more complex and need different sub-types of linear accelerators in a sequence to bring the energy up to 250 MeV for clinical use.
The existing technology of high current proton LINACs for nuclear physics experiments have been redesigned for the needs of proton therapy, which does not need particle currents in the µA to mA region. Going to much higher radio-frequencies allowed to produce higher electric fields and led to a reduction of the system length [93]. Furthermore, this high frequency enables to design smaller structure sizes allowing drift tube apertures with radii as small as 2–3 mm. The side effect of using normal conducting resonator structures is that only pulses of about 5 µs length can be generated at a maximum repetition rate of approximately 200 Hz [95]. Thus, the mean current obtained is in the range of about 20 nA due to the low duty cycle.
Two groups are currently leading the challenge to build the first clinical PTS made from these RF-LINACs: The TOP-IMPLART project headed by Dr Picardi at ENEA Frascati, Italy [96] and the CERN spin-off company AVO-ADAM with its project LIGHT [97]. Both systems follow the all-LINAC approach. For the first acceleration of the proton beam behind the ion source, a Radio-Frequency Quadrupole unit (RFQ) is used up to energies of about 5 MeV followed by Drift Tube LINACs (DTL) [95]. The second stage of acceleration is performed by a so-called SCDTL (Side-Coupled Drift Tube LINAC) [98], which has been specially designed for standard PTSs. They close the gap between the RFQs for the low beta (β = v/c) protons (β < 0.06) and the Coupled Cavity LINACs (CCL) that are becoming efficient at energies around 70 MeV [93, 99]. With the CCL technology, the proton beam can be accelerated to 230 MeV or more for clinical systems. These systems require a length between 25 and 30 m [93].
This design promises to obtain any kind of proton energy at about the same beam current but better beam quality than degraded cyclotron beams. Thus, also protons with 35–70 MeV are expected with high beam quality and tens of nanoampere beam currents being sufficient for preclinical pMBRT therapy. The calculated transverse root mean square (rms) emittances for these systems are ≈0.5 π mm mrad (unnormalized) at 70 MeV (i.e., 0.2 π mm mrad normalized) [96] which is at 70 MeV at least a factor of 10 smaller than a beam extracted from a therapy cyclotron after the ESS unit with 16–36 π mm mrad [80]. The feature of having an exceedingly small transverse emittance makes the linear accelerators a promising candidate for producing proton minibeams with high brilliance and very high dose rates within single 5 µs pulses.
For analyzing the potential of a 3 GHz LINAC in detail, a 3D-simulation study has been performed in collaboration with AVO-ADAM to calculate the transport and focusing of the proton beam for generating a minibeam at the target [64]. In this collaboration, extensive Monte Carlo beam transport simulations were performed. As an alternative to the all-LINAC approach, a 16 MeV proton beam coming from a tandem accelerator was injected into the third section of the LINAC system as a post-accelerator to achieve a proton energy of 70 MeV. Details of this setup will be presented in section 3. Simulations showed focusing the beam after the LINAC via a magnetic quadrupole triplet is feasible of delivering a mean current of 18 nA to a target in a square of 0.1 × 0.1 mm at a repetition rate of 200 Hz [64].
It can be concluded that the energy, the beam currents and the spot size required for minibeam applications in a preclinical facility can be fulfilled with this technology. Besides these crucial parameters, the LINAC technology has the potential to provide additional features that are of interest for standard proton therapy but can be advantageous even for pMBRT. In contrast to cyclotron systems, a fast and continuous energy modulation can be obtained by switching off the power of LINAC modules and tuning the power of the last LINAC module, without the use of degrading material. This feature together with high dose rates and spot scanning opens the possibility of fast dose repainting in all three dimensions. Additionally, this electronic energy variation enables to reduce remarkable amounts of radiation shielding and in consequence save costs [96].
While the beam originating from a therapy cyclotron is quasi-continuous, the LINAC structure accelerates the beam in bunches of a few µs due to its inherent duty-cycle with a mean beam current in the order of about 20 nA. Considering a bunch width of 5 µs and a maximum repetition rate of 200 Hz the beam current in one bunch is expected to be about 20 µA (1,000 times higher). In the case of small irradiation targets where only one or a few single minibeam spots are required, the effective dose rate can be orders of magnitude higher than the mean current indicates. An exploitation of this unique feature of LINACs can be used for combining pMBRT with FLASH therapy approaches. Furthermore, the omission of a beam degrading unit keeps the phase space conserved and simplifies the generation of proton minibeams. Finally, a further unique feature of LINACs is their modular structure allowing a sequenced expansion of maximum proton energy by simply adding additional structures in a later stage of the preclinical tests, e.g., for the irradiation of larger animals or even to convert the preclinical facility into a clinical one.
The two above-mentioned systems are following the roadmap on their way to manufacture a clinical standard PTS for a pencil beam scanning therapy using an all-LINAC approach. The prototype machine of TOP-IMPLART has successfully accelerated protons up to 35 MeV at the ENEA institute [100]. AVO-ADAM produced a proton beam of 52 MeV at their test site at CERN in autumn 2018 [101]. Especially the company AVO-ADAM is already very close to the energy of 70 MeV for the first phase of a preclinical irradiation facility. Nevertheless, the target energy of 70 MeV is not yet reached. Therefore, a full characterization and validation of the calculated beam parameters (emittance, beam current) is still missing. However, a measurement of the emittance at 7.5 MeV (after the first SCDTL module) showed a value ≈0.1π mm mrad (normalized; rms) and a peak beam pulse current of 39 µA [97].
3 Design Idea of a Research Facility for Preclinical Experiments
Following the technology assessment described above, the RF-LINAC system seems to open the largest possibilities for conducting preclinical research with pMBRT. In early 2020, the LIGHT and the TOP-IMPLART system are not accessible for external users for performing preclinical experiments with small animals, since they are used for commissioning and validation.
However, we are convinced that it is the right time to enter the next step of preclinical pMBRT research. Concerning the technological aspects, we have the goal to minimize costs and time to operation of a preclinical facility. Therefore, we propose using an accelerator at an operating laboratory and implementing copies of a certain number of LINAC modules as a post-accelerator for reaching the desired preclinical energies. Moreover, there is another striking argument for this combined solution. Due to the complexity and the administrative effort of performing preclinical tests with living animals, it is very likely that the available beam time of dedicated stand-alone preclinical setup cannot be used for research at full capacity. We suggest the usage of an existing high energy tandem accelerator and installing a LINAC at one of its high-energy beam lines. The total beam time can be shared between the preclinical experiments and other multidisciplinary research activities, such as material analysis and modification, fundamental radiobiology research or high resolution accelerator mass spectroscopy [82]. This approach would also distribute the running costs of such a facility among several other research partners. In the following, we want to propose a setup for such a preclinical pMBRT irradiation facility. This will be discussed with respect to technological and biomedical aspects.
3.1 The Tandem-LINAC Setup
Regarding the arguments listed above, a tandem Van-de-Graaff machine was chosen as an injector for the LINAC-structures for performing an in-depth study of the beam transport for two reasons. First, the brilliance of a tandem beam when using a multi-cusp ion-source [102] is well-suited to the acceptance of a LINAC. Second, the sharp energy distribution of the tandem beam after the 90° analyzing magnet can be utilized for matching the direct current (DC) beam coming from the tandem to the longitudinal phase acceptance of a 3 GHz LINAC and gaining transmission. In the following, the results from a study performed by Mayerhofer et al. are summarized [64]: The injection energy of 16 MeV was set for two reasons. First, this spares additional costs, because two SCDTL modules compared to the all-LINAC solution can be skipped. Second, starting at 16 MeV instead of 5 MeV (all-LINAC solution) the power efficiency and the effective field gradient (i.e., gained energy per unit length) of the SCDTL is already enhanced [96]. The 6-dimensional phase space of the proton beam coming from the “pre”-accelerator have been measured at the Munich 14 MV tandem Van-de-Graaff accelerator. These data have been used as a realistic input for the beam transport simulations through the LINAC structures and the focusing unit to produce minibeams [64]. For the acceleration from 16 to 70 MeV in total two SCDTL and four CCL (Coupled Cavity LINAC) modules are foreseen. The total setup is shown in Figure 1.
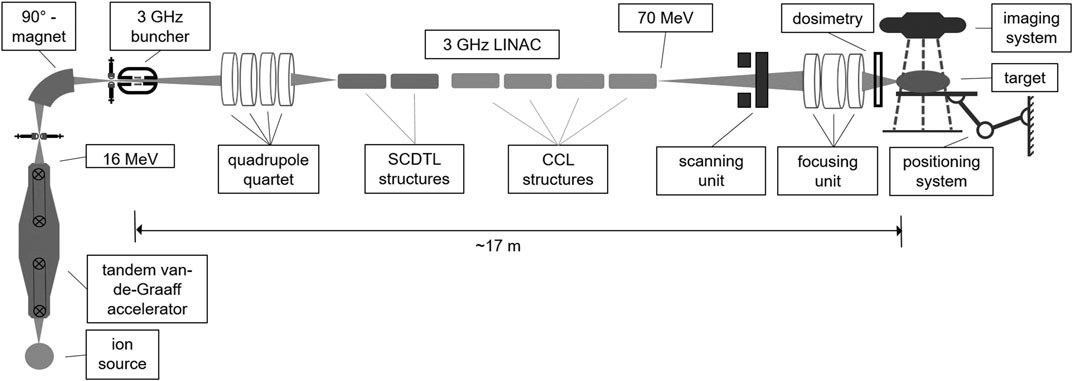
FIGURE 1. Scheme of the proposed tandem-LINAC system including matching unit, focusing stage as well as an imaging and target positioning system.
For adapting the 6-dimensional phase space of the DC proton beam coming from the tandem to the acceptance of the LINAC, a buncher unit and a quadrupole quartet is needed matching the longitudinal phase space as well as the transverse phase space, respectively. Details about the optimization of this matching process can be taken from Mayerhofer et al. [64]. Simulations show that these two matching devices increase the total transmission of the proton beam coming from the tandem through the LINAC up to 49%. This means that every second proton from the tandem is accelerated to 70 MeV and can be used for experiments. Although it is difficult to compare, in the case of a proton therapy cyclotron only one out of 500 accelerated protons reaches the target if an energy of 70 MeV is selected. All the others are stopped in the ESS producing secondary radiation that needs to be shielded with meter-thick walls.
As discussed in Section 2, we are convinced that the generation of the smallest possible proton minibeams with highest possible PVDR and at high dose rate can only be realized by focusing rather than by collimation. Thus, after extraction of the 70 MeV beam, a focusing unit has been foreseen. This ion-optical lens system demagnifies the beam to the desired minibeam spot size. Simulations showed that an electromagnetic quadrupole-triplet configuration is feasible of producing proton minibeams in a square of 0.1 mm by 0.1 mm with more than 90% of the LINAC beam, meaning an overall transmission of the tandem beam of 49% [64]. Based on measurements of the proton beam brilliance as delivered at the Munich tandem accelerator, the calculated beam current delivered in this area at the focal plane (under vacuum) is 18 nA at a repetition rate of 200 Hz. The total length of the focusing unit after the LINAC is about 6 m, therein the distance from the last quadrupole to the focal plane is designed to be about 0.6 m [64], leaving space for dose and position detection as well as an vacuum window. A two-dimensional scanning system is planned upstream of the focusing triplet. This system is still in the design phase, but a total deviation of 7 and 9 cm seems feasible in x- and y-direction, respectively. The last part of the nozzle directly before the target consists of a vacuum window and a detector measuring dose and beam position. In the case of minibeams with submillimeter sizes the propagation of the protons in air should be minimized as much as possible for keeping beam size as small as possible and PVDR values high. A detailed planning of the monitor detectors is the scope of an extra study.
We expect that similar beam parameters can be achieved when using other accelerator types as injectors for the 3 GHz LINAC proposed above. Besides using the high energy tandem accelerator, also other options exist for boosting the protons to preclinically relevant energies. As mentioned, the lowest possible proton energy for injecting into an SCDTL module is 5 MeV. This energy could even be delivered by a single ended or a smaller tandem Van-de-Graaff machine. Furthermore, in previous studies so-called “Cyclinac” solutions were already investigated for standard proton therapy. In these proposals a cyclotron was favored injecting the beam into a LINAC e.g., at energies of 24 or 62 MeV [103, 104]. Both options open additional possibilities for adapting RF-LINAC approaches for the use at existing facilities. It could be worth investigating some of these suggestions for their potential to produce proton minibeams for preclinical or even clinical applications.
3.2 End Station for Small Animal Irradiation
The configuration of the end station for the preclinical irradiation experiments has not been performed in detail, yet. However, basic considerations are described based on the experience of the small animal irradiations conducted so far. Although single fraction experiments are easier to accomplish in terms of positioning accuracy, a preclinical pMBRT system should be capable of conducting multi-fraction experiments. At the SNAKE setup a positioning system was installed for performing mouse ear irradiations. The day-to-day animal positioning during the experiment with a fractionated irradiation relied on the imaging of the blood vessels in the ear with a camera at ambient light. Using cross correlation of the reference image (day 1) and the actual image (day 2–4) the displacement vector was determined by calculation of the x- and y-displacement as well as the corresponding angular displacement (θ). A correction of the angular displacement in z-directions was excluded by animal holder design. The calculated displacement was corrected using a movable stage with motorized x- and y-axis and also a rotation axis in the plane perpendicular to the beam, where the animal holder was mounted. Using this positioning system, a day-to-day (relative) position accuracy of the ears of 0.1 mm was achieved [65].
For treatment of deeper tumors, the imaging of blood vessels via a camera will not be possible. For this kind of experiments, an image-guided system feasible of resolving deeper lying organs, bones, and tissue is necessary. We are in favor of using an existing system such as the SARRP (Xstrahl LTD., Surrey, United Kingdom) that is capable of performing a CT scan of the object to be irradiated. This stand-alone X-ray irradiation platform system already offers an imaging and positioning system for small animals with an accuracy of 0.24 mm [105]. First systems have already been added to particle irradiation facilities, where the SARRP system is adapted to be used also with a beam coming from an exterior accelerator [106, 107]. We are convinced that it is possible to further improve the positioning accuracy of such a system, e.g., by adding a more precise positioning system. However, we think that additional in-depth studies are inevitable to investigate this issue of absolute and relative positioning accuracy together with multi-fractionation treatment schemes, with a focus on the technical side as well as on the bio-medical side.
3.3 Biomedical Aspects
The final goal we are heading for is treating patients with proton minibeams in the near future. The motivation behind this large endeavor is the promising potential of reducing stress for the patient and side effects while keeping or even improving tumor control. Therefore, when thinking on building a dedicated pre-clinical research platform it is crucial to determine which tumor indication can profit most as this in consequence defines the animal models, which must be investigated during the pre-clinical phase.
Looking at particle therapy statistics it is clear that many different kinds of tumors are treated. In the search of suitable tumors which can be considered for pMBRT the following criteria were included. In general, tumors showing bad prognosis and tumors where patients suffer from severe side effects have to be tackled by new therapy options. In these cases, pMBRT might be able to add further benefit in curing the tumor as well as in sparing of normal tissue. Furthermore, one should also consider the economic aspects including possible sponsors and target market, as without financing the barrier for introducing a new therapy method is insurmountable. Therefore, the tumors which are investigated should not only be rare occasions. In the following, a selection of tumors is presented that meet one or more of the mentioned criteria for different reasons.
Pediatric cancer, which gives 10% of all treated tumors in 2014 [45] is quite promising for several reasons. First, the infant tissue is still highly proliferating and therefore prone to severe long-term side effects. As treatment gets more effective and more successful, cured patients have good prognosis and the number of long-term survivors is increasing [108]. With longer lifetime, the probability of developing late side effects or the chance for induction of a secondary cancer is increasing, especially for children as the lifespan is long in this case [109]. Last, geometrical factors also play a role in the side effects occurring in childhood cancer therapy. As the organs and therefore also the organs at risk are much smaller compared to adults, hitting these organs during treatment can have much more effect in infants compared to adults. Furthermore, children are more prone to secondary total body dose. Therefore, it is necessary to deliver effective treatment with less morbidity [110]. pMBRT is decreasing the damage to the healthy tissue as much as possible while keeping tumor control. The sparing effect in this case can be achieved in two ways; the number of healthy cells hit by radiation is decreased and these are mostly exposed to lethal doses and therefore cannot develop long term side effects. In particular, the genetic damage within cells after the first division after proton minibeam irradiation of high PVDR was much reduced as measured by induction of micronuclei [30].
Apart from childhood cancer in total and here in particular brain tumors and other malignancies of the nervous system, which are the second most diagnosed cancers in children [108] and also occur in adults, seem to be a promising target for pMBRT. The major problem in these kinds of cancers is that neurons cannot be reproduced, once dead the function is lost [111]. However, we think that neuronal cells are well suited to be spared using pMBRT. The reason lies in their structure. Neuronal cells can get up to 1 m long [112] with a cell nucleus of only several µm in diameter [113]. The cell nucleus is the sensitive target for radiation as damage to the DNA, which is stored in the nucleus, is the major reason for cell death and secondary malignancies. Therefore, when hitting a composite of neuronal cells with a pMBRT of small size the probability of hitting the nucleus of a single cell is <<1, which makes pMBRT advantageous compared to conventional therapy. Additionally, if this potential advantage can be verified using the preclinical setup, neuronal bundles such as the spinal cord are no longer a hyper-sensitive organ at risk. This opens the possibility for treatment of other tumors as also irradiation from the back might get possible.
Two types of tumors, where severe side effects occur in the affected organ itself, could be a promising target for pMBRT: lung cancers such as non-small cell lung cancer (NSCLC) and hepatocellular carcinoma (HCC) in the liver. In NSCLC, severe side effects like pulmonary fibrosis, esophagitis, pneumonitis and bronchial fistulae occur [114]. HCC have an even worse prognosis, as treatment of this kind of tumors can only be successfully performed with particle therapy not with X-rays [115]. But still the treatment is limited to small tumors as severe side effects can occur, since the liver is one of the most radiosensitive organs in the human body [116]. In these two presented examples new therapy schemes, including dose escalation and different angles of incidence, are discussed to increase patient survival, decrease side effects and open the possibility for treatment of bigger tumors and tumors of progressed state. pMBRT with its spatial fractionation scheme is an attractive option to bring radiotherapy to the next stage of controlling these tumors.
Furthermore, not only the radiosensitivity of the affected organ itself can serve as criterion for selection of a suitable tumor type for pMBRT. Also, the location in the body can serve as a deciding factor. We think that especially the treatment of tumors in the abdominal region can benefit from pMBRT. The abdominal organs are quite radiosensitive [116] and sparing those by using minibeams opens the possibility of new dose and fractionation schemes using alternative angles of incidence.
The journey for pMBRT to a first patient treatment is still long. In our opinion, investigating the effectiveness of pMBRT in treatment of glioblastoma, NSCLC and HCC can be a first step in this direction. Possible studies on pMBRT urgently need to include the investigation of side effects in the affected organs as well as in adjacent organs. Focus should not only lie on acute but also on the late side effects such as cognitive and organ disfunction, fibrosis and secondary cancer. These studies will help to identify further types of cancers which could be treated such as the ones in the abdominal region. Preclinical studies should also fully exploit the feasibility of new therapy schemes using different angles of incidence, hypofractionation and dose escalation.
4 Conclusion and Outlook
In this article we proposed a setup for a preclinical irradiation facility to fully exploit the potential of proton minibeam radiotherapy. This method is an advancement of standard proton therapy, which has already proven in first experiments to spare healthy tissue in the entrance channel by spatial fractionation with proton minibeams at sizes in the range of 0.1 mm. We are convinced that the pMBRT research stands at a turning point, right now. In preclinical experiments performed since 2013, first evidence was found that pMBRT is a promising new approach in radio-oncology. It has the potential of further improving the quality of outcome in cancer treatment of solid tumors. However, the research on important physical parameters and methods as well as research on biomedical aspects is still in its infancy.
We have given an overview of the status and limitations of pre-clinical experiments performed in mice and rats so far. First, we are convinced that the production of minibeams using focusing is superior compared to collimation and opens a wider range of options for testing with different beam shapes and sizes. Furthermore, when using focusing the beam current can be sustained, whereas collimation is blocking the majority of particles and thus reducing beam current at the patient. At the same time collimation increases secondary radiation due to nuclear interaction of the protons with the collimator material. In addition, the production of collimators for beams of this small size is challenging and only possible when accepting disadvantages such as decreasing the PVDR and inflexibility in the treatment process.
Second, we have discussed the beam parameters, that have to be met by a preclinical irradiation facility, i.e., beam energy, dose rate and beam spot size. Due to the animals’ size, the proton energy must allow experiments at and below 70 MeV. The required beam current at the isocenter is defined by the dose rate, which has to be applied to the tumor. We conclude that this should be at least 1-2 nA, as available in standard proton therapy. The available size of the minibeams should be as low as 0.1 mm as shown in preclinical studies to generate the least side effects. The mentioned values for beam current and beam size represent only a lower limit. A powerful preclinical setup should give the opportunity to vary both parameters in a wide range to be able to fully exploit the limitations of pMBRT and evaluating the sweet spots for these parameters for a most efficient treatment and highest possible benefit for the patient. We believe that it shall be feasible to tune the beam current into a domain where analyzing minibeams in combination with the FLASH effect can be studied as well as synergies with hypofractionation can be evaluated. These considerations led to the conclusion that existing therapy cyclotrons are not well suited for such a preclinical facility, since they only fulfill a fraction of these parameter setting and therefore do not offer the full versatility for preclinical research.
We discussed that 3 GHz RF-LINACs, currently developed for standard proton therapy facilities, are expected to meet all requirements for pMBRT and have the potential to serve as a unique and versatile tool for evaluating the benefits in all possible directions. We presented beam transport simulations of this modular acceleration concept that support its performance regarding possible beam currents and small emittances, which enable tiny pencil beams. However, the two currently existing LINAC systems are in the stage of the commissioning of their first prototype for standard proton therapy systems and therefore not open for preclinical research purposes. Nevertheless, the commissioning of these LINACs delivers first promising results and reported acceleration up to 52 MeV.
Therefore, we suggest using an existing Van-de-Graaff tandem accelerator as an injector for a 3 GHz RF-LINAC post-accelerator consisting of two SCDTL and 4 CCL structures providing a beam at 70 MeV. Beam transport simulations showed that focusing the proton beam to 0.1 mm spots is feasible at a proton current of 18 nA. This provides a comparatively cost-efficient solution, where infrastructure and beamtime can be shared with other research activities. The current status of knowledge and technology also allows to think out of the box. The pulsed time structure of the minibeams provided by RF-LINACs provides the opportunity to exploit the FLASH effect in combination with pMBRT. This has the potential to further enhance normal tissue protection and give space for thinking about the opportunity of hypofractionation.
Concerning an end station for small animal irradiation, we think the adaption of a commercially available SARRP beamline is the best option as CT-imaging and animal positioning is already implemented there. But adaptions have still to be done to achieve the required positioning accuracy of 0.1 mm to address all options for reirradiation the same beam spot in multi-fraction experiments.
In the last section we examined the biomedical aspects that have to be considered for a preclinical facility. When starting a project with a large financial investment and a long lead time until realization, it is mandatory to develop clear goals for the research focus. We think that defining tumor types that profit most from using pMBRT compared to standard proton therapy is one of the most important objectives. The definition has to rely on the occurrence of severe acute and late side effects in current radiation treatment, as well as if tumors are untreatable or difficult to treat with current radiotherapy approaches. Not to forget, the frequency of occurrence, as this could help to raise funding for research and a latter implementation into clinics. Consequently, we conclude that primary attention in the biomedical research at the facility should lie on glioma, NSCLC and HCC. On the other hand, investigation of the potential reduction of side effects on healthy tissue, especially neuronal cells and abdominal organs is of similar importance.
As the final goal is to treat patients, also certification procedures have to be considered already at this early stage of research gaining more and more importance as the process is going on. For example, it is necessary to take into account how the technology can be transferred into clinics and how the implementation can be realized. Furthermore, it has to be checked if and in which parts of the process already existing certification can be adapted to pMBRT, making the process of approval faster and more cost-efficient.
From the technical point of view, the outcome of the preclinical phase will give answers to the definition of the optimal parameters for beam size, beam shape (pencil or planar), the application method (focusing or collimating) and the necessary beam current. Furthermore, new application schemes such as interlacing of beams from different irradiation angles with heterogeneous tumor dose can be investigated by a dedicated preclinical approach. These adaptations could further improve the sparing of the healthy tissue but add a whole new parameter space to the testing phase.
To conclude, in our opinion it is the right time for the implementation of a preclinical irradiation facility for performing further in-depth research programs with this new treatment modality and for understanding its radiobiological mechanisms in different tissues as well as in living animals. Consequently, this research on advantages and limitations will prepare the field of pMBRT for the treatment of the first patient. Taking all this together, proton minibeam radiotherapy is a fascinating area of investigation and a huge step into the future of precision tumor therapy.
Author Contributions
GDA, JR, and GDO planned the study, GDA, JR did literature research, SG, MS, and MM contributed the chapters which are in their area of expertize, GDA and JR combined all parts and wrote the body of the paper, GDO, MS, MM, and SG did proofreading.
Funding
This study was funded by the DFG cluster of excellence, and the European transnational access program RADIATE.
Conflict of Interest
Author GD was employed by the company Datzmann interact and innovate GmbH. The remaining authors declare that the research was conducted in the absence of any commercial or financial relationships that could be construed as a potential conflict of interest.
References
1. Baskar R, Lee KA, Yeo R, Yeoh K-W. Cancer and radiation therapy: current advances and future directions. Int J Med Sci (2012). 9(3):193–9. doi:10.7150/ijms.3635
2. Delaney G, Jacob S, Featherstone C, Barton M. The role of radiotherapy in cancer treatment: estimating optimal utilization from a review of evidence-based clinical guidelines. Cancer (2005). 104(6):1129–37. doi:10.1002/cncr.21324
3. Barton MB, Jacob S, Shafiq J, Wong K, Thompson SR, Hanna TP, et al. Estimating the demand for radiotherapy from the evidence: a review of changes from 2003 to 2012. Radiother Oncol (2014). 112(1):140–4. doi:10.1016/j.radonc.2014.03.024
4. Rosenblatt E. Radiotherapy in cancer care: facing the global challenge Vienna, Austria: International Atomic Energy Agency (IAEA) (2017). 578 p.
5. Wilson RR. Radiological use of fast protons. Radiology (1946). 47(5):487–91. doi:10.1148/47.5.487
6. Bortfeld T. An analytical approximation of the Bragg curve for therapeutic proton beams. Med Phys (1997). 24(12):2024–33. doi:10.1118/1.598116
7. Jones DT, Schreuder AN, Symons JE, de Kock EA, Vernimmen FJ, Stannard CE, et al. Status report of the NAC particle therapy programme. Strahlenther Onkol (1999). 175 (Suppl. 2):30–2. doi:10.1007/bf03038883
8. Pedroni E, Bacher R, Blattmann H, Böhringer T, Coray A, Lomax A, et al. The 200-MeV proton therapy project at the Paul Scherrer Institute: conceptual design and practical realization. Med Phys (1995). 22(1):37–53. doi:10.1118/1.597522
9. Munzenrider JE, Austin-Seymour M, Blitzer PJ, Gentry R, Goitein M, Gragoudas ESet al. Proton therapy at harvard. Strahlentherapie (1985). 161(12):756–63.
10. Miller DW. A review of proton beam radiation therapy. Med Phys (1995). 22(11):1943–54. doi:10.1118/1.597435
11. Slater JM, Miller DW, Archambeau JO. Development of a hospital-based proton beam treatment center. Int J Radiat Oncol Biol Phys (1988). 14(4):761–75. doi:10.1016/0360-3016(88)90099-5
12. Slater JM, Archambeau JO, Miller DW, Notarus MI, Preston W, Slater JD. The proton treatment center at loma Linda university medical center: rationale for and description of its development. Int J Radiat Oncol Biol Phys (1992). 22(2):383–9. doi:10.1016/0360-3016(92)90058-p
13. Arcangeli G, Mauro F, Morelli D, Nervi C. Multiple daily fractionation in radiotherapy: biological rationale and preliminary clinical experiences. Eur J Cancer (1965). 15(9):1077–83. doi:10.1016/0014-2964(79)90123-3
14. Farr JB, Moskvin V, Lukose RC, Tuomanen S, Tsiamas P, Yao W. Development, commissioning, and evaluation of a new intensity modulated minibeam proton therapy system. Med Phys (2018). 45.9: 4227–4237. doi:10.1002/mp.13093
15. Köhler A. A method of deep roentgen irradiation without injury to the skin. AJR Am J Roentgen (1909). 14(5):141–2. doi:10.1259/arr.1909.0062
16. Meyer J, Eley J, Schmid TE, Combs SE, Dendale R, Prezado Y. Spatially fractionated proton minibeams. Br J Radiol (2019). 92(1095):20180466. doi:10.1259/bjr.20180466
17. Laissue JA, Geiser G, Spanne PO, Dilmanian FA, Gebbers J-O, Geiser M, et al. Neuropathology of ablation of rat gliosarcomas and contiguous brain tissues using a microplanar beam of synchrotron-wiggler-generated X rays. Int J Cancer (1998). 78(5):654–60. doi:10.1002/(sici)1097-0215(19981123)78:5<654::aid-ijc21>3.0.co;2-l
18. Slatkin DN, Dilmanian FA, Spanne PO. Method for microbeam radiation therapy. Brookhaven Science Associates LLC. U.S. Patent No US5339347A (1992).
19. Anschel DJ, Bravin A, Romanelli P. Microbeam radiosurgery using synchrotron-generated submillimetric beams: a new tool for the treatment of brain disorders. Neurosurg Rev (2011). 34(2):133–42. doi:10.1007/s10143-010-0292-3
20. Grotzer MA, Schültke E, Bräuer-Krisch E, Laissue JA. Microbeam radiation therapy: clinical perspectives. Phys Med (2015). 31(6):564–7. doi:10.1016/j.ejmp.2015.02.011
21. Prezado Y, Deman P, Varlet P, Jouvion G, Gil S, Le Clec’ H C, et al. Tolerance to dose escalation in minibeam radiation therapy applied to normal rat brain: long-term clinical, radiological and histopathological analysis. Radiat Res (2015). 184(3):314–21. doi:10.1667/rr14018.1
22. Prezado Y, Jouvion G, Guardiola C, Gonzalez W, Juchaux M, Bergs J, et al. Tumor control in RG2 glioma-bearing rats: a comparison between proton minibeam therapy and standard proton therapy. Int J Radiat Oncol Biol Phys (2019). 104(2):266–71. doi:10.1016/j.ijrobp.2019.01.080
23. Prezado Y, Sarun S, Gil S, Deman P, Bouchet A, Le Duc G. Increase of lifespan for glioma-bearing rats by using minibeam radiation therapy. J Synchrotron Radiat (2012). 19(Pt 1):60–5. doi:10.1107/s0909049511047042
24. Prezado Y, Jouvion G, Patriarca A, Nauraye C, Guardiola C, Juchaux M, et al. Proton minibeam radiation therapy widens the therapeutic index for high-grade gliomas. Sci Rep (2018). 8(1):1–10. doi:10.1038/s41598-018-34796-8
25. Landberg T, Chavaudra J, Dobbs J, Hanks G, Johansson K-A, Möller T, et al. Report 50. J ICRU (1993). os26(1):NP. doi:10.1093/jicru/os26.1.report50
26. Bouchet A, Bräuer-Krisch E, Prezado Y, El Atifi M, Rogalev L, Le Clec’ HC, et al. Better efficacy of synchrotron spatially microfractionated radiation therapy than uniform radiation therapy on glioma. Int J Radiat Oncol Biol Phys (2016). 95(5):1485–94. doi:10.1016/j.ijrobp.2016.03.040
27. Bräuer-Krisch E, Serduc R, Siegbahn EA, Le Duc G, Prezado Y, Bravin A, et al. Effects of pulsed, spatially fractionated, microscopic synchrotron X-ray beams on normal and tumoral brain tissue. Mutat Res (2010). 704(1-3):160–6. doi:10.1016/j.mrrev.2009.12.003
28. Sammer M, Girst S, Dollinger G. Optimizing proton minibeam radiotherapy by interlacing and heterogeneous tumor doLeerraum the basis of calculated clonogenic cell survival. Sci Rep (Forthcoming 2020).
29. Prezado Y, Fois GR. Proton‐minibeam radiation therapy: a proof of concept. Med Phys (2013). 40(3):031712. doi:10.1118/1.4791648
30. Zlobinskaya O, Girst S, Greubel C, Hable V, Siebenwirth C, Walsh DWM, et al. Reduced side effects by proton microchannel radiotherapy: study in a human skin model. Radiat Environ Biophys (2013). 52(1):123–33. doi:10.1007/s00411-012-0450-9
31. Sammer M, Zahnbrecher E, Dobiasch S, Girst S, Greubel C, Ilicic K, et al. Proton pencil minibeam irradiation of an in-vivo mouse ear model spares healthy tissue dependent on beam size. PloS One (2019). 14(11):e0224873. doi:10.1371/journal.pone.0224873
32. Reindl J, Girst S. pMB FLASH - status and perspectives of combining proton minibeam with FLASH radiotherapy. Cancer Immunol Res (2019). 1(1).
33. Withers HR, Thames HD. Dose fractionation and volume effects in normal tissues and tumors. Am J Clin Oncol (1988). 11(3):313–29. doi:10.1097/00000421-198806000-00008
34. Straile WE, Chase HB. The use of elongate microbeams of X-rays for simulating the effects of cosmic rays on tissues: a study of wound healing and hair follicle regeneration. Radiat Res (1963). 18(1):65. doi:10.2307/3571426
35. Dilmanian FA, Eley JG, Rusek A, Krishnan S. Charged particle therapy with mini-segmented beams. Front Oncol (2015). 5:269. doi:10.3389/fonc.2015.00269
36. Dilmanian FA, Eley JG, Krishnan S. Minibeam therapy with protons and light ions: physical feasibility and potential to reduce radiation side effects and to facilitate hypofractionation. Int J Radiat Oncol Biol Phys (2015). 92(2):469–74. doi:10.1016/j.ijrobp.2015.01.018
37. Datzmann G, Dollinger G, Goeden C, Hauptner A, Körner H-J, Reichart P, et al. . The Munich microprobe SNAKE: first results using 20 MeV protons and 90 MeV sulfur ions. Nucl Instrum Methods Phys Res (2001). 181(1-4):20–6. doi:10.1016/s0168-583x(01)00549-3
38. Hauptner A, Dietzel S, Drexler GA, Reichart P, Krücken R, Cremer T, et al. Microirradiation of cells with energetic heavy ions. Radiat Environ Biophys (2004). 42(4):237–45. doi:10.1007/s00411-003-0222-7
39. Girst S, Greubel C, Reindl J, Siebenwirth C, Zlobinskaya O, Walsh DWM, et al. Proton minibeam radiation therapy reduces side effects in an in vivo mouse ear model. Int J Radiat Oncol Biol Phys (2016). 95(1):234–41. doi:10.1016/j.ijrobp.2015.10.020
40. Sammer M, Teiluf K, Girst S, Greubel C, Reindl J, Ilicic K, et al. Beam size limit for pencil minibeam radiotherapy determined from side effects in an in-vivo mouse ear model. PloS One (2019). 14(9):e0221454. doi:10.1371/journal.pone.0221454
41. Prezado Y, Jouvion G, Hardy D, Patriarca A, Nauraye C, Bergs J, et al. . Proton minibeam radiation therapy spares normal rat brain: long-term clinical, radiological and histopathological analysis. Sci Rep (2017). 7(1):1–7. doi:10.1038/s41598-017-14786-y
42. Lamirault C, Doyère V, Juchaux M, Pouzoulet F, Labiod D, Dendale R, et al. . Short and long-term evaluation of the impact of proton minibeam radiation therapy on motor, emotional and cognitive functions. Sci Rep (2020). 10(1):13511. doi:10.1038/s41598-020-70371-w
43. Eley JG, Chadha AS, Quini C, Vichaya EG, Zhang C, Davis J, et al. . Pilot study of neurologic toxicity in mice after proton minibeam therapy. Sci Rep (2020). 10(1):11368. doi:10.1038/s41598-020-68015-0
44. Tobola-Galus A, Swakon J, Olko P. Dosimetric characterization of collimators for spatially fractionated proton therapy of the eye. Radiat Protect Dosim (2018). 180(1-4):351–4. doi:10.1093/rpd/ncy015
45.Particle Therapy Co-Operative Group. Particle Therapy Co-operatvie Group. Available from: https://www.ptcog.ch/ (Accessed April 20, 2020).
46. Bortfeld T, Paganetti H, Kooy H. MO-A-T-6B-01: proton beam radiotherapy—the state of the art. Med Phys (2005). 32(6Part13):2048–9. doi:10.1118/1.1999671
47. Koehler AM, Schneider RJ, Sisterson JM. Range modulators for protons and heavy ions. Nucl Instrum Methods (1975). 131(3):437–40. doi:10.1016/0029-554x(75)90430-9
48. August Schubiger P. PSI life sciences newsletter 1988. Switzerland: Paul Scherrer Institut (1989). Available from: http://inis.iaea.org/search/search.aspx?orig_q=RN:21075176.
49. Blattmann H, Coray A. A horizontal proton beam line for the development of a scanning technique. Radiother Oncol (1990). 17(1):17–20. doi:10.1016/0167-8140(90)90044-w
50. Grevillot L, Bertrand D, Dessy F, Freud N, Sarrut D. A Monte Carlo pencil beam scanning model for proton treatment plan simulation using GATE/GEANT4. Phys Med Biol (2011). 56(16):5203–19. doi:10.1088/0031-9155/56/16/008
51. De Marzi L, Patriarca A, Nauraye C, Hierso E, Dendale R, Guardiola C, et al. . Implementation of planar proton minibeam radiation therapy using a pencil beam scanning system: a proof of concept study. Med Phys (2018). 45(11):5305–16. doi:10.1002/mp.13209
52. Pedroni E, Meer D, Bula C, Safai S, Zenklusen S. Pencil beam characteristics of the next-generation proton scanning gantry of PSI: design issues and initial commissioning results. Eur. Phys. J. Plus (2011). 126(7):1–27. doi:10.1140/epjp/i2011-11066-0
53. Farr JB, Mascia AE, Hsi W‐C, Allgower CE, Jesseph F, Schreuder AN, et al. . Clinical characterization of a proton beam continuous uniform scanning system with dose layer stacking. Med Phys (2008). 35(11):4945–54. doi:10.1118/1.2982248
54. Karger CP, Jäkel O. Current status and new developments in ion therapy. Strahlenther Onkol (2007). 183(6):295–300. doi:10.1007/s00066-007-1645-x
55. Brenner DJ, Hall EJ. Secondary neutrons in clinical proton radiotherapy: a charged issue. Radiother Oncol (2008). 86(2):165–70. doi:10.1016/j.radonc.2007.12.003
56. Bräuer-Krisch E, Bravin A, Zhang L, Siegbahn E, Stepanek J, Blattmann H, et al. . Characterization of a tungsten/gas multislit collimator for microbeam radiation therapy at the European synchrotron radiation facility. Rev Sci Instrum (2005). 76(6):64303. doi:10.1063/1.1915270
57. Bräuer-Krisch E, Requardt H, Brochard T, Berruyer G, Renier M, Laissue JA, et al. . New technology enables high precision multislit collimators for microbeam radiation therapy. Rev Sci Instrum (2009). 80(7):74301. doi:10.1063/1.3170035
58. Guardiola C, Peucelle C, Prezado Y. Optimization of the mechanical collimation for minibeam generation in proton minibeam radiation therapy. Med Phys (2017). 44(4):1470–8. doi:10.1002/mp.12131
59. Lansonneur P, Mammar H, Nauraye C, Patriarca A, Hierso E, Dendale R, et al. . First proton minibeam radiation therapy treatment plan evaluation. Sci Rep (2020). 10(1):7025. doi:10.1038/s41598-020-63975-9
60. Peucelle C, Nauraye C, Patriarca A, Hierso E, Fournier-Bidoz N, Martínez-Rovira I, et al. . Proton minibeam radiation therapy: experimental dosimetry evaluation. Med Phys (2015). 42(12):7108–13. doi:10.1118/1.4935868
61. Charyyev S, Artz M, Szalkowski G, Chang C-W, Stanforth A, Lin L, et al. . Optimization of hexagonal-pattern minibeams for spatially fractionated radiotherapy using proton beam scanning. Med Phys (2020). 47(8):3485–95. doi:10.1002/mp.14192
62. Martínez-Rovira I, Fois G, Prezado Y. Dosimetric evaluation of new approaches in GRID therapy using nonconventional radiation sources. Med Phys (2015). 42(2):685–93. doi:10.1118/1.4905042
63. Schneider T, De Marzi L, Patriarca A, Prezado Y. Advancing proton minibeam radiation therapy: magnetically focussed proton minibeams at a clinical centre. Sci Rep (2020). 10(1):1384. doi:10.1038/s41598-020-58052-0
64. Mayerhofer M, Datzmann G, Degiovanni A, Dimov V, Dollinger G. Magnetically focused 70 MeV proton minibeams for preclinical experiments combining a tandem accelerator and a 3 GHz linear post accelerator. Med Phys (Forthcoming 2020).
65. Sammer M, Dombrowsky AC, Schauer J, Oleksenko K, Bicher S, Schwarz B, et al. . Normal tissue response of combined temporal and spatial fractionation in proton minibeam radiation therapy. Int J Radiat Oncol Biol Phys (2020). In Press, Available online 14 August 2020 doi:10.1016/j.ijrobp.2020.08.027
66. Schmelmer O, Dollinger G, Datzmann G, Goeden C, Körner H-J. A novel high precision slit system. Nucl Instrum Methods Phys Res (1999). 158(1-4):107–12. doi:10.1016/s0168-583x(99)00357-2
67. Sammer M, Greubel C, Girst S, Dollinger G. Optimization of beam arrangements in proton minibeam radiotherapy by cell survival simulations. Med Phys (2017). 44(11):6096–104. doi:10.1002/mp.12566
68. Hälg RA, Besserer J, Boschung M, Mayer S, Lomax AJ, Schneider U. Measurements of the neutron dose equivalent for various radiation qualities, treatment machines and delivery techniques in radiation therapy. Phys Med Biol (2014). 59(10):2457–68. doi:10.1088/0031-9155/59/10/2457
69. De Marzi L, Da Fonseca A, Moignier C, Patriarca A, Goudjil F, Mazal A, et al. Experimental characterisation of a proton kernel model for pencil beam scanning techniques. Phys Med (2019). 64:195–203. doi:10.1016/j.ejmp.2019.07.013
70. Grassberger C, Dowdell S, Sharp G, Paganetti H. Motion mitigation for lung cancer patients treated with active scanning proton therapy. Med Phys (2015). 42(5):2462–9. doi:10.1118/1.4916662
71. Knopf A-C, Lomax AJ. In the context of radiosurgery—pros and cons of rescanning as a solution for treating moving targets with scanned particle beams. Phys Med (2014). 30(5):551–4. doi:10.1016/j.ejmp.2014.03.010
72. Zhang Y, Huth I, Weber DC, Lomax AJ. A statistical comparison of motion mitigation performances and robustness of various pencil beam scanned proton systems for liver tumour treatments. Radiother Oncol (2018). 128(1):182–8. doi:10.1016/j.radonc.2018.01.019
73. Rietzel E, Bert C. Respiratory motion management in particle therapy. Med Phys (2010). 37(2):449–60. doi:10.1118/1.3250856
74. Friedrich T. Proton RBE dependence on dose in the setting of hypofractionation. Br J Radiol (2020). 93(1107):20190291. doi:10.1259/bjr.20190291
75. Hong TS, Wo JY, Yeap BY, Ben-Josef E, McDonnell EI, Blaszkowsky LS, et al. . Multi-Institutional phase II study of high-dose hypofractionated proton beam therapy in patients with localized, unresectable hepatocellular carcinoma and intrahepatic cholangiocarcinoma. J Clin Oncol (2016). 34(5):460–8. doi:10.1200/jco.2015.64.2710
76. Quinn TJ, Hamstra D. Hypofractionation in prostate cancer using proton beam. Int J Radiat Oncol Biol Phys (2019). 105(4):723–6. doi:10.1016/j.ijrobp.2019.08.006
77. Favaudon V, Caplier L, Monceau V, Pouzoulet F, Sayarath M, Fouillade C, et al. . Ultrahigh dose-rate FLASH irradiation increases the differential response between normal and tumor tissue in mice. Sci Transl Med (2014). 6(245):245ra93. doi:10.1126/scitranslmed.3008973
78.Schillo, M. Global industrial development of accelerators for charged particle therapy. in: The 5th International Particle Accelerator Conference (IPAC 2014); 2014 Jul; Dresden, Germany. Geneva, Switzerland: JACoW (2014). p. 5. Available from: https://inspirehep.net/literature/1313907 (Accessed September 20, 2020).
79. Berger MJ, Inokuti M, Andersen HH, Bichsel H, Powers D, Seltzer SM, et al. . Report 49. J ICRU (1993). os25(2):NP. doi:10.1093/jicru/os25.2.report49
80. Rizzoglio V, Adelmann A, Baumgarten C, Frey M, Gerbershagen A, Meer D, et al. . Evolution of a beam dynamics model for the transport line in a proton therapy facility. Phys Rev Accel Beams (2017). 20(12). doi:10.1103/physrevaccelbeams.20.124702
81.Varian medical systems inc. Flash forward TM - konsortium.. (2020). Available from: https://www.varian.com/de/about-varian/research/flashforward-consortium (Accessed September 20, 2020).
82. Dollinger G, Faestermann T. Physics at the Munich tandem accelerator laboratory. Nucl Phys News (2018). 28(1):5–12. doi:10.1080/10619127.2018.1427405
83. Schippers JM, Dölling R, Duppich J, Goitein G, Jermann M, Mezger A, et al. . The SC cyclotron and beam lines of PSI’s new protontherapy facility PROSCAN. Nucl Instrum Methods Phys Res (2007). 261(1-2):773–6. doi:10.1016/j.nimb.2007.04.052
84. Liang Z, Chen W, Qin B, Liu X, Liu K, Zha J. Design of the energy selection system for proton therapy based on GEANT4. In: Proceedings of Cyclotrons; September 11–16, 2016; Zurich, Switzerland. Geneva, Switzerland; JACoW (2016). Available from: https://accelconf.web.cern.ch/cyclotrons2016/papers/mod01.pdf (Accessed September 20, 2020).
85. Coutrakon G, Slater JM, Ghebremedhin A. Design considerations for medical proton accelerators. In: Proceedings of the 1999 particle accelerator conference (cat. No.99CH36366); March 27–April 2, 1999; New York, United States. New York, NY; IEEE (1999). p. 11–5.
86. Jongen Y, Laycock S, Abs M, Amelia J-C, Beeckman W, Kleeven W, et al. . The proton therapy system for the NPTC: equipment description and progress report. Nucl Instrum Methods Phys Res (1996). 113(1-4):522–5. doi:10.1016/0168-583x(95)01319-9
87. Langner UW, Eley JG, Dong L, Langen K. Comparison of multi-institutional Varian ProBeam pencil beam scanning proton beam commissioning data. J Appl Clin Med Phys (2017). 18(3):96–107. doi:10.1002/acm2.12078
88. Perrin A. TRAVEL v4.07: user manual (2007). Available from: https://lombarda.web.cern.ch/DTL-linac4/Travel.pdf (Accessed April, 2007).
89. Sabaiduc C, Boiesan T, Carlson M, Du D, Piazza LAC, Ryikov V, et al. . Best 70P cyclotron comissioning at INFN LN Legnaro. In: Proceedings of Cyclotrons; September 11–16, 2016; Zurich, Switzerland. Geneva, Switzerland: JACoW (2016). 241–3. Available from: http://accelconf.web.cern.ch/cyclotrons2016/papers/tud04.pdf.
90. Denker A, Bundesmann J, Daerow T, Fanselow T, Hildebrand D, Hiller U, et al. . Status of HZB cyclotron. In: Proceedings of Cyclotrons; September 20, 2013; Vancouver, BC. Geneva, Switzerland: JACoW (2019). p. 254–6. Available from: https://accelconf.web.cern.ch/CYCLOTRONS2013/papers/moppt002.pdf (Accessed September 20, 2020).
91. Damato B, Kacperek A, Chopra M, Campbell IR, Errington RD. Proton beam radiotherapy of choroidal melanoma: the Liverpool-Clatterbridge experience. Int J Radiat Oncol Biol Phys (2005). 62(5):1405–11. doi:10.1016/j.ijrobp.2005.01.016
92. Bettega D, Calzolari P, Chauvel P, Courdi A, Herault J, Iborra N, et al. . Radiobiological studies on the 65 MeV therapeutic proton beam at Nice using human tumour cells. Int J Radiat Biol (2000). 76(10):1297–303. doi:10.1080/09553000050151565
93. Amaldi U, Braccini S, Puggioni P. High frequency linacs for hadrontherapy. Rev Accl Sci Tech (2009). 02(01):111–31. doi:10.1142/s179362680900020x
94. Hamm RW, Crandall KR, Potter JM. Preliminary design of a dedicated proton therapy linac. In: Conference record of the 1991; 2019 May 19–24; Melbourne, Australia. IEEE Particle Accelerator Conference (1991). p. 2583–5:4.
95. Degiovanni A. Future trends in linacs. In: Proceedings of the CAS-CERN accelerator school on accelerators for medical applications/CERN yellow reports: school proceedings; 2015 May 26–June 5; Vösendorf, Austria. CERN Yellow Reports (2017). p. 151:Vol. 1.
96. Ronsivalle C, Picardi L, Ampollini A, Bazzano G, Marracino F, Nenzi P, et al. . First acceleration of a proton beam in a side coupled drift tube linac. Europhys Lett (2015). 111(1):14002. doi:10.1209/0295-5075/111/14002
97.Degiovanni, A, Adam, J, Aguilera Murciano, D, Ballestrero, S, Benot-Morell, A, Bonomi, Ret al. . Status of the commissioning of the LIGHT prototype. in: The 9th International Particle Accelerator Conference; 2018 Jun; Vancouver, BC. Geneva, Switzerland: JACoW Publishing (2018). p. 4. Available from: https://inspirehep.net/literature/1691387 (Accessed September 20, 2020).
98. Picardi L, Ronsivalle C, Vignati A. TOP LINAC design; Progetto del TOP LINAC (1997). Report No.: ENEA Technical Report RT/INN/97/17. Available from: https://www.osti.gov/etdeweb/biblio/610871 (Accessed September 20, 2020).
99. De Martinis C, Giove D, Amaldi U, Berra P, Crandall K, Mauri M, et al. . Acceleration tests of a 3GHz proton linear accelerator (LIBO) for hadrontherapy. Nucl Instrum Methods Phys Res (2012). 681:10–5. doi:10.1016/j.nima.2012.04.017
100. Picardi L, Ampollini A, Bazzano G, Cisbani E, Ghio F, Montereali RM, et al. . Beam commissioning of the 35 MeV section in an intensity modulated proton linear accelerator for proton therapy. Phys Rev Accel Beams (2020). 23(2):20102. doi:10.1103/physrevaccelbeams.23.020102
101. Caldara M., Presentation a the OMA International Conference on Medical Accelerators and Particle Therapy. (2019). Available from: https://indico.cern.ch/event/803528/contributions/3378749/attachments/1902950/3141897/20190618_OMA_BD_Caldara.pdf (last access date: 17.10.2020)
102. Moser M, Greubel C, Carli W, Peeper K, Reichart P, Urban B, et al. . Transport of a high brightness proton beam through the Munich tandem accelerator. Nucl Instrum Methods Phys Res (2015). 348:34–42. doi:10.1016/j.nimb.2014.11.068
103. Amaldi U, Berra P, Crandall K, Toet D, Weiss M, Zennaro R, et al. . LIBO—a linac-booster for protontherapy: construction and tests of a prototype. Nucl Instrum Methods Phys Res (2004). 521(2-3):512–29. doi:10.1016/j.nima.2003.07.062
104. Degiovanni A, Amaldi U. Proton and carbon linacs for hadron therapy. (2014). p. 4. Report No.: CERN-ACC-2014-362. Available from: https://cds.cern.ch/record/2062622 (Accessed September 20, 2020).
105. Matinfar M, Ford E, Iordachita I, Wong J, Kazanzides P. Image-guided small animal radiation research platform: calibration of treatment beam alignment. Phys Med Biol (2009). 54(4):891–905. doi:10.1088/0031-9155/54/4/005
106. Kim MM, Irmen P, Shoniyozov K, Verginadis , Cengel KA, Koumenis C, et al. . Design and commissioning of an image-guided small animal radiation platform and quality assurance protocol for integrated proton and X-ray radiobiology research. Phys Med Biol (2019). 64(13):135013. doi:10.1088/1361-6560/ab20d9
107. Ford E, Emery R, Huff D, Narayanan M, Schwartz J, Cao N, et al. . An image-guided precision proton radiation platform for preclinical in vivo research. Phys Med Biol (2017). 62(1):43–58. doi:10.1088/1361-6560/62/1/43
108. Siegel R, DeSantis C, Virgo K, Stein K, Mariotto A, Smith T, et al. . Cancer treatment and survivorship statistics, 2012. CA A Cancer J Clin (2012). 62(4):220–411. doi:10.3322/caac.21149
109. Hall EJ. Intensity-modulated radiation therapy, protons, and the risk of second cancers. Int J Radiat Oncol Biol Phys (2006). 65(1):1–7. doi:10.1016/j.ijrobp.2006.01.027
110. Chapman TR, Ermoian RP. Proton therapy for pediatric cancer: are we ready for prime time?. Future Oncol (2017). 13(1):5–8. doi:10.2217/fon-2016-0373
111. Pettmann B, Henderson CE. Neuronal cell death. Neuron (1998). 20(4):633–47. doi:10.1016/s0896-6273(00)81004-1
112. Fletcher DA, Theriot JA. An introduction to cell motility for the physical scientist. Phys Biol (2004). 1(1-2):T1–10. doi:10.1088/1478-3967/1/1/t01
113.McGovern Medical School at UTHealth Department of Neurobiology and Anatomy. Neuroscience Online an electronic textbook from the neurosciences (1997). Available from: https://nba.uth.tmc.edu/neuroscience/ (Accessed May 15, 2020).
114. Werner-Wasik M, Paulus R, Curran WJ, Byhardt R. Acute esophagitis and late lung toxicity in concurrent chemoradiotherapy trials in patients with locally advanced non-small-cell lung cancer: analysis of the radiation therapy oncology group (RTOG) database. Clin Lung Canc (2011). 12(4):245–51. doi:10.1016/j.cllc.2011.03.026
115. Greten TF, Malek NP, Schmidt S, Arends J, Bartenstein P, Bechstein W, et al. . Diagnosis of and therapy for hepatocellular carcinoma. Z Gastroenterol (2013). 51(11):1269–326 [in German, with English summary]. doi:10.1055/s-0033-1355841
Keywords: proton minibeam radio therapy, spatial fractionation, linear accelerator, preclinic, irradiation facility, pencil beam scanning, proton therapy
Citation: Datzmann G, Sammer M, Girst S, Mayerhofer M, Dollinger G and Reindl J (2020) Preclinical Challenges in Proton Minibeam Radiotherapy: Physics and Biomedical Aspects. Front. Phys. 8:568206. doi: 10.3389/fphy.2020.568206
Received: 31 May 2020; Accepted: 22 September 2020;
Published: 26 November 2020.
Edited by:
Yolanda Prezado, INSERM U1021 Signalisation Normale et Pathologique de l’Embryon aux Thérapies Innovantes des Cancers, FranceReviewed by:
Pawel Olko, Institute of Nuclear Physics, PolandAvraham Dilmanian, Stony Brook University, United States
Copyright © 2020 Datzmann, Sammer, Girst, Mayerhofer, Dollinger and Reindl. This is an open-access article distributed under the terms of the Creative Commons Attribution License (CC BY). The use, distribution or reproduction in other forums is permitted, provided the original author(s) and the copyright owner(s) are credited and that the original publication in this journal is cited, in accordance with accepted academic practice. No use, distribution or reproduction is permitted which does not comply with these terms.
*Correspondence: Gerd Datzmann, Z2VyZEBkYXR6bWFubi5ldQ==