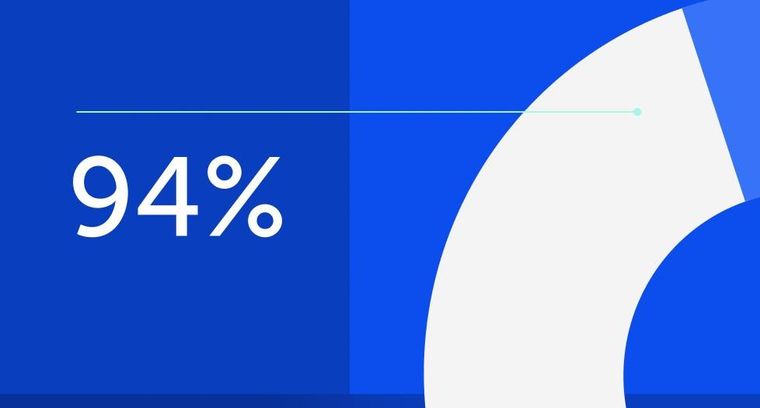
94% of researchers rate our articles as excellent or good
Learn more about the work of our research integrity team to safeguard the quality of each article we publish.
Find out more
REVIEW article
Front. Phys., 30 September 2020
Sec. Medical Physics and Imaging
Volume 8 - 2020 | https://doi.org/10.3389/fphy.2020.568027
This article is part of the Research TopicApplied Nuclear Physics at AcceleratorsView all 57 articles
Cognitive dysfunction induced by ionizing radiation remains a major concern in radiation therapy as well as in space mission projects. Both fields require sophisticated approaches to improve protection of the brain and its neuronal circuits. Radiation therapy related research focusses on advanced techniques imposing maximal effect on the tumor while minimizing toxicity to the surrounding tissue. Research for example has led to the revival of spatially fractionated radiation therapy (SFRT) and the advent of FLASH radiotherapy. To investigate the influence of the space radiation environment on brain cells, low dose, high LET radiation in addition to simulated microgravity have to be studied. Both research areas, however, call for cutting-edge cellular systems that faithfully resemble the architecture of the human brain, its development and its regeneration to understand the mechanisms of radiation-induced neurotoxicity and their prevention. In this review, we discuss the proposed mechanisms of neurotoxicity such as the loss of complexity within the neuronal networks, vascular changes, or neuroinflammation. We compare the current in vivo and in vitro studies of neurotoxicity including animal models, animal and human neural stem cells, and neurosphere models. Particularly, we will address the new and promising technique of generating human brain organoids and their potential use in radiation biology.
Humans are unavoidably exposed to ionizing radiation (IR) from environmental and artificial sources [1]. The severity of radiation effects on the human organism depends on the dose and quality of radiation. High-LET (linear energy transfer) charged particles like carbon (12C) ions lead, due to densely ionization events, to more severe damage compared to sparsely ionizing low-LET radiation, e.g., X- and γ-rays [2]. Regarding radiation impacts, the human brain is divided into different sub-structures at risk [3], but the effects of IR on the brain are still poorly understood. Thus, additional research is required in the fields of radiation therapy and space research for adapted risk assessment and for the development of adequate shielding methods [4, 5]. Cell [6] and animal models [7] provided first insights into the mechanisms underlying radiation-induced neurotoxicity such as impaired connectivity and neuronal function that govern cognitive capabilities. However, these models lack the unique and complex architecture of the human brain, e.g., expanded neuronal cell diversity of millions of neurons organized in distinct functional regions, allowing higher cognitive abilities in humans [8]. So-called cerebral brain organoids exhibit several key features of the in vivo brain architecture and cell complexity and thus offer a more realistic microenvironment to investigate the impact of different noxae on the human brain [9]. This innovative model may improve our understanding of the mechanisms of radiation-induced late effects and enable the development of adequate countermeasures. Furthermore, brain organoids could be helpful to test the impact of novel irradiation modalities like SFRT and FLASH therapy before they are applied to the clinics.
The present knowledge of IR effects on the human brain is based primarily on data from epidemiological studies, particularly on Japanese atomic bomb survivors, as well as on cancer patients treated with radiotherapy. However, the affected persons were exposed at different ages and to different radiation qualities that influenced the varying outcomes of the radiation impacts [10, 11].
Epidemiological studies of prenatally exposed atomic-bomb survivors of Hiroshima and Nagasaki demonstrate that IR has, dependent on the dose and developmental stage, adverse effects on the developing brain. These manifest themselves in reduced head volumes in ~42 % of the children irradiated in utero with doses from 0.5 to 0.99 Gy at gestational weeks 8–15 [12]. Additionally, exposure to 0.5–0.99 Gy negatively affected the neurocognition, shown in a decline in school performances in 38 % [13] and a reduction of 21–29 points in intelligence quotient (IQ)-tests per 1 Gy absorbed dose for individuals irradiated at gestational weeks 8–15 [14]. These studies demonstrate the harmful effects of IR on the developing brain, particularly at critical stages of neocortical development, such as gestational weeks 8–15. Furthermore, a cohort study of children that underwent conventional photon-radiotherapy with doses of 0.01–2.8 Gy during infancy (<18 months) due to cutaneous hemangioma, displayed cognitive impairments at the age of 18/19 years. These effects were noticeable as a decrease in high school attendance at doses greater than 0.1 Gy as well as a dose-response relation for cognitive performance measured by cognitive tests aimed at learning ability, and logical reasoning [15]. IR-induced long-term effects also have been reported for pediatric and adult patients with primary or metastatic brain tumors receiving 4–65 Gy cranial photon-radiotherapy [11]. Adverse effects become apparent ≥6 month after treatment and manifest themselves in progressive impairments, which are comparable with Alzheimer's disease, such as deficits in memory, executive function, sustained attention, processing speed and learning, leading to a reduction of the patient's quality of life [11, 16, 17]. The extend of the radiation damage depends on age at irradiation, total dose, fractionation, and field size and the combination with other noxae, e.g., chemotherapeutics [11, 17]. Children are more strongly affected than adults due to the higher radio-sensitivities of the developing brain and a longer lifespan. It was shown that children that received 20–55 Gy of craniospinal photon radiotherapy due to central nervous system (CNS) malignancies at the age of ≤ 3 years displayed intellectual disability (Ø = −1.34 IQ-points per year) compared to non-irradiated children (Ø = + 0.91 IQ-points per year) [18]. This phenomenon was observable after the completion of therapy and during the follow-up time of ~7.5 years. Also, photon irradiation of adult patients with primary, supratentorial brain tumors verifiably led to radiation-induced cognitive deficits, measured by worse results in experimental memory tests after fractionated radiotherapy with a total dose to the tumor of about 46–63 Gy [19]. Altogether, these studies confirm conventional treatment related impairments at any age of patients. Even though photon-based radiotherapy has been continually improved and still remains the standard modality for the treatment of brain tumors, particle-based radiotherapy that mostly relies on protons and carbon ions and enables a more efficient treatment of brain cancer patients, came into focus [20]. In contrast to photons, particle irradiation exhibits an advantageous dose distribution due to a unique absorption profile in the tissue with lower entrance doses and well-defined depth range with maximum dose deposition and increasing relative biological effectiveness (RBE) at the end of their range, called Bragg Peak. This depth-dose profile enables precise irradiation of deep-seated tumors while sparing the surrounding healthy tissue [21]. The use of protons and heavy ions to treat cancer was invented by researchers of the Lawrence Berkeley Laboratory, CA, USA in 1958 (Berkeley “synchrocyclotron”) [22]. However, it was first implemented clinically in Japan, in 1994, using the Heavy Ion Medical Accelerator in Chiba (HIMAC) [23]. In Europe, a patient pilot study (1997–2008) with 12C-ions at the Heavy Ion Synchrotron (SIS18) at GSI Helmholtz Center for Heavy Ion Research, Darmstadt (Germany), showed a promising outcome of tumor control and minimal toxicity for irradiation of skull base chordomas and chondrosarcomas [21, 24]. It led to the transfer of particle radiotherapy from physics laboratories to the Heidelberg Ion-Beam Therapy (HIT) Center that was opened in 2009. Due to a decreased neurotoxicity and increased success rate of particle therapy, the number of facilities and thus the number of patients treated with protons and 12C-ions steadily increased in recent years1. Meanwhile, 104 particle therapy facilities are in clinical operation worldwide2. For protons, reduced neurotoxic effect compared to photons was demonstrated by the studies of Kahalley et al. [25, 26]. Pediatric patients treated for brain tumors with protons showed less neuropsychological impairments in terms of intelligence, perceptual reasoning, processing speed, and working memory than those treated with X-rays [25, 26]. However, considering that continuously improved diagnostics and radiation treatments lead to an increased number of patients with longer lifespan and thus a higher risk for developing treatment-related late effects, the need for adequate model systems and suitable irradiation modalities arises.
Cognitive deficits are also a major concern in long-term space mission beyond the shielding of the Earth's magnetosphere as reviewed by Cucinotta et al. [27]. Radiation-induced neurotoxicity is of particular interest, because the radiation environment in space differs significantly from the terrestrial. Terrestrial radiation exposure result mainly from radon-emitted, low energy alpha particles, and sparsely ionizing radiations, i.e., X-, β-, or γ-rays, while in deep space high-energy protons, helium, and heavier ions predominate. Therefore, space radiation induced biological damages can differ from those experienced on Earth. Moreover, effects of other spaceflight relevant factors, such as microgravity or disturbed circadian rhythm, may synergistically impair brain function. During long-term space missions the estimated total body equivalent dose for astronauts amounts to 1–2 mSv per day [28]. Potential CNS risks are reduced motor functions, neurocognitive deficits, probability of the occurrence of Alzheimer's disease and premature aging [27]. As an example, a NASA study on identical twins indicates the potentially harmful effects of galactic cosmic radiation on the human brain. The space-twin, that participated in a one-year mission, demonstrated a post-flight decline in cognitive performance and speed, which persisted during the whole observation time of 6 months, compared to the twin that stayed on Earth [29]. These risks may compromise astronauts' behavior under emergency conditions in deep space and justify the need of improved shielding methods. As the space radiation field and its effects are rather complex, it is difficult to predict the consequences for astronauts. Innovative accelerator facilities such as those available at FAIR/GSI can be used to simulate this mixed radiation field allowing a deeper insight into the CNS risk associated with cosmic radiation as well as the development of improved shielding methods. In summary, studying the effects of IR on the human brain is highly relevant, not only in context of risk assessment in radiotherapy, but also in terms of space research with a focus on shielding technologies.
Because the molecular and cellular mechanisms underlying IR-induced neurocognitive deficits are still largely unknown, in vitro or in vivo models of the brain's neurophysiology were developed to address this topic.
One of the earliest neuronal cell types are so-called neural stem cells (NSCs). These multipotent, dividing stem cells exist in the developing, embryonic brain as well as in the adult brain of mammalian organisms and represent the early precursors of the CNS-generating neurons and glia cells (astrocytes and oligodendrocytes) [30]. In rodents, two special niches of high-density cell division have been identified: the subventricular zone (SVZ) lining the lateral forebrain ventricles and the subgranular layer of the dentate gyrus (DG) in the hippocampus. In both sites of the rodent brain lifelong neurogenesis, i.e., differentiation of self-renewing NSCs into neurons and glia, takes place. These newly born neurons and glia cells then mature and migrate into the cortical, neuronal circuits to participate in cognitive functions like learning and memory [31, 32]. Whether and how adult human neurogenesis takes part, is highly debated and a challenging research topic [33, 34]. De novo formation of human neurons has been analyzed using doublecortin (DCX) or PSA-NCAM as markers for intermediate progenitor cells and early immature neurons (often dubbed “neuroblasts”). However, the source of these cells may not be embryonic-like NSCs but rather astrocytic cells [35]. Thus, human NSCs derived from embryonic/pluripotent stem cells may not reflect adult neurogenesis and its radiosensitivity, yet, they exhibit features of cancer stem cells [36] and therefore still contribute significantly to the radiation biology field. Consequently, mammalian NSCs were isolated from fetal or adult brain or differentiated from pluripotent stem cells, cultured as two-dimensional (2D) monolayers and used as in vitro models for radiation effects on neurogenesis [37]. Likewise, the more mature neurons can be isolated from human brain or can be differentiated in vitro from human NSCs. By applying various differentiation and growth factors, neurons of distinct subtypes including GABAergic, dopaminergic, and motor neurons were already generated and successfully used for studying neuronal functionality, synaptic plasticity, and injury [38]. However, these 2D-cell cultures do not consider the three-dimensionality of brain tissue. As a more realistic model system, neurospheres (NS), i.e., three-dimensional (3D) aggregates of several neural and neuronal progenitor types, derived from NSCs in suspension, were established. Within the NS, neural cells are able to self-renew, generate various neuronal and glial subtypes at different stages of maturation [39] and display neuronal function in the form of spontaneously generated action potentials [40]. An organ-like microenvironment with some degree of structural or organizational integrity can be achieved, as shown by Merz et al. [41], by culturing rodent and human tissue slices of about 300 μm at an air-liquid interface. These slices even display the tissues' natural 3D-architecture up to 6 months. Finally, animal models, especially rodents, are used to investigate the radiation effects on the brain. The advantages of using animals as model organisms include, besides physiological similarity to humans, the entire vascularization and the complex biochemical and biomechanical microenvironment of the whole mammalian brain [7, 42].
As seen from Table 1, several studies focused on the potential effects of sparsely IR on hippocampal neurogenesis. The observed radiation effects include a dose-dependent loss of NSCs [43, 44, 46, 47], a decreased proliferation rate of surviving NSCs [41, 43, 46–48, 53], impairments in the differentiation capability into neuronal and glial cells [44] and morphological changes in dendritic structure and neurite length [45, 50] leading to changes in synaptic transmission and therefore to a disturbed neuronal plasticity. Interestingly, these effects already occur at doses of about 1–2 Gy. This is of particular interest, because fractionated radiotherapy is usually performed with 1–3 Gy per fraction [68]. As seen from studies by Isono et al. [47], Morini et al. [48], and Yokota et al. [46], the effects on the NSCs were enhanced when irradiation was performed with particles, e.g., 12C-ions. These results verify the high radiation-sensitivity of non-differentiated neural stem and progenitor cells and correlate with the radiation-sensitive stage of early embryonic neurogenesis. Despite differences in neurogenesis between humans and rodents, as discussed above, there are some similarities in the radiation response, e.g., activation of microglia [51, 52, 56, 58]. Astrocyte and endothelium mediated secondary vascular abnormalities and the subsequent disruption of the blood brain barrier cause this activation of microglia, the phagocytic cells of the CNS. This induces chronic neuroinflammation via the release of pro-inflammatory cytokines, which also leads to degenerative changes in the white matter [51, 56, 57, 69]. In vitro and in vivo studies have shown that IR can further lead to an increase of oxidative stress producing DNA-damage and causes apoptotic cell death in neuronal, glial, and endothelial cell types in NSCs and NS [44, 49] as well as in rats [59]. In rodents, the radiation-induced loss of glial oligodendrocyte progenitor cells followed by demyelination of neurons ends up with white matter necrosis [59], which is also seen in humans [70]. These impacts on mammalian brains may contribute to impairments in behavior and to memory deficits as assessed by the Barnes maze [55, 60] and visual discrimination tests [54] and may also mirror cognitive defects seen in humans.
Studies analyzing the effects of space-relevant 56Fe-ions on rodents (see Table 1) have shown that doses <1 Gy impair neurogenesis as well as neuronal function [62, 63] and can cause cognitive deficits. The irradiated animals exhibit persistent hippocampal and cortical based performance decrements in memory and behavioral tests [60, 61, 64, 65]. Comparative studies demonstrated that even exposure to 0.2 Gy 56Fe (1 GeV/u) resulted in cognitive impairments whereas X-ray exposure did not result in memory deficits until doses > 10 Gy indicating a high effectiveness of Fe (estimated RBE ~ 50) [60]. Additionally, mice that were exposed to protons with doses as low as 0.1–0.5 Gy also showed a decreased hippocampal cell proliferation and deficits in neurogenesis and synaptic plasticity [66]. These findings demonstrate the stronger effect of particle irradiation compared to photons and strengthen concerns about potential cognitive changes after space mission. However, the animal studies have been performed predominantly with 56Fe-ions and therefore do neither represent the full complexity of the space radiation environment, nor the continuous irradiation over several weeks and months in space.
In summary, these findings demonstrate the complex and dynamic effects of radiation on multiple cell types of the brain, including NSCs, oligodendrocytes, astrocytes, microglia, and neurons as well as vascular endothelial cells (Figure 1). Furthermore, comparative studies demonstrated the greater effectiveness of space relevant 56Fe-ions on CNS cells in comparison to photons. These radiation mediated impacts may contribute to diverse brain damages and neurodegenerative diseases, even though the detailed mechanisms of cognitive impairments remain largely unknown.
The model systems listed above, particularly the in vitro ones, have disadvantages. Despite the progress that has been made in understanding the hallmarks of brain development and neurogenesis as well as in investigating the neurotoxicity of IR using of 2D-cell cultures, these cell systems display distinct differences in morphology, metabolism, and differentiation compared to the in vivo situation [6]. Specifically, the NSC monolayer system only allows investigating IR effects on the very early and primitive neurogenesis as discussed above. Therefore, they neither address processes in the adult brain nor are they capable of reflecting the complex cell-layering and diversity seen in the human brain's architecture. The same applies to neurons cultivated in vitro as monolayers. In contrast, 3D-neurospheres contain cells at multiple stages of differentiation but lack cell-organization and hierarchical complexity found in the human brain in vivo [71]. A more relevant model is presented by human brain slice cultures from postmortem tissue or resected tissue from operations [72]. In vitro, their morphology and physiological characteristics can be partly preserved for up to 50 days [73]. However, there are striking differences between postmortem and resected slices in the processing of slices and transfer to the in vitro conditions, neuronal survival has to be sustained by elaborate culture conditions and finally procurement of human brain slices is challenging and impedes larger studies. In radiation biology, rodent studies are frequently used allowing first insights into the molecular mechanisms underlying cognitive dysfunction. However, apart from the ethical discussions on the use of animals in research, mice, and humans display species-specific differences, that speak against the use of animals as a model [42]. In addition to obvious differences in brain size and architecture due to the folding of the human brain, particularly the existence of neural stem cells, called outer radial glia, residing in the outer subventricular zone, and a greater diversity in neuronal cell types [74–76] sets the human brain apart from other mammalian ones. Notably, even between homologous human and mouse cell types, single-nucleus RNA-sequencing analyses verified significant differences in gene expression [8]. All these main features of the human brain allow for higher cognitive functions, but also justify the need for a more adapted and adequate brain model to investigate the molecular and functional effects of radiation in various brain regions and cell types. Some of the disadvantages of the discussed models can be overcome by human brain organoids that will be discussed in the following chapter.
In recent years, so-called brain or cerebral organoids, differentiated from human embryonic stem cells (hESCs) or patient-relevant induced pluripotent stem cells (iPSCs), came into focus as a novel in vitro tool in regenerative medicine/disease modeling [77], whereas the potential for radiation biology is not yet exploited. Organoids are 3D spontaneously self-organized, organ-like structures that are able to develop into various interdependent brain regions containing different organ-specific cell types arranged in distinct layers (Figure 2). In contrast to rodent models, these organoids exhibit several key features of the human in vivo brain organogenesis like an independent progenitor layer with the unique neural stem cells called outer radial glia. In addition, it has already been reported that animal models failed to recapitulate the symptoms of human neurological diseases, such as micro- and macrocephaly while human organoids have this ability. Therefore, they provide a novel predictive preclinical tool to investigate the detailed molecular mechanisms underlying congenital, cognitive diseases and the effects of potential neuro-therapeutics in functional human tissues [78]. Brain organoids thus provide the opportunity to model unique features of early human brain development, as well as human neurogenesis and neuro-regeneration [9, 79], and may allow a better understanding of the mechanisms underlying ionizing radiation-induced cognitive impairments and disease.
Figure 2. Immunocytochemical staining of a cerebral organoid slice showing human brain-like hierarchical organization with the radial glia marker PAX6 (Paired box 6, red) in the inner ventricular layer of the brain lobes. Nuclei stained with Hoechst 33342 (blue). Scale bar: 200 μm.
Mimicking in vivo patterning by supplementing external patterning factors, organoids of diverse functional brain regions, including organoids of the ventral forebrain [80, 81], midbrain [82, 83], hypothalamus [84], cerebellum [85], and pituitary [86] were generated in suspension. Culture conditions were improved by using spinning reactors and orbital shakers at moderate throw and speed, which provide enhanced nutrient and oxygen supply and culture time without causing adverse shear stress. Such sustained suspension culture leads to an enhanced differentiation process of neurons compared to those derived in 2D-monolayers and the formation of active neuronal networks [84, 87–89]. In addition, different region-specific spheroids, such as cortical spheroids of the dorsal and ventral forebrain, can be fused to generate so-called “assembloids” (Figure 3). Consequently, migration of interneurons and the interactions between different brain regions or different cell types can be tracked [90–93]. Other studies demonstrated the incorporation of microglia as the brains innate immune cells into the organoid-model [94, 95]. This allows investigating the effects of combined therapies, e.g., radio- and immunotherapy, on the human CNS. Another approach is the generation of brain tumors in normal organoids as a realistic microenvironment [96] that will permit a better understanding of tumor and normal tissue interaction in response to IR. Yet, radiobiological studies using brain organoids have not been published. However, brain organoids were recently implemented in ground-based experiments investigating the effects of microgravity on neural progenitor cell fate [97]. They showed an altered gene expression of rostral-caudal and cortical markers that may influence brain structure and physiology, indicating the usability of brain organoids in space radiation research to identify the mechanisms affecting brain function and, e.g., in the development of new shielding methods to protect the brain's neuronal circuits. In our own studies, we were able to reproducibly show radiation impacts such as apoptosis and necrosis changing the structure and composition of cerebral organoids (unpublished data).
Figure 3. Pluripotent, embryonic stem cell (ESCs) -based 2D-, 3D cell- and organoid systems as in vitro tools for studying the effects of ionizing radiation on the human brain.
But even if organoids currently are viewed as state-of-the-art in vitro models of the human brain, there are still challenges to be overcome. Because of the lack of endothelial cells and thus vascularization, organoids will develop a necrotic core and terminal maturation and differentiation is limited. Therefore, Giandomenico et al. [98] chose an alternative approach for an improved oxygen supply cultivating organoid slices at an air-liquid interface. These organoid slices demonstrated an increased survival rate and an extensive axon outgrowth reminiscent of nerve tracts. Another issue is the lack or scarcity of glial cell populations. A new differentiation approach uses more complex differentiation factors to generate mature oligodendrocytes in brain organoids [99]. Nevertheless, the batch variations and thus heterogeneity of the generated organoids still poses a problem for statistically significant and expressive results, although there are new methods for generating more homogeneous organoids by using microfilaments as floating scaffolds [100]. Despite these improvements, it is technically very challenging to obtain homogeneous batches and the organoids show statistical variabilities also seen in animal experiments; therefore, more sophisticated bioengineering techniques are presently explored. One approach is the use of 3D bio printing technologies to produce consistent scaffolds as a basis of organoid formation. A further challenge is the adaptation of standardized analytical protocols such as immunochemical staining procedures to 3D-brain organoids. In the same line, single-cell mRNA sequencing [88] techniques have been proven more meaningful than standard polymerase chain reactions (PCR). Despite these challenges, brain organoids represent a versatile model system allowing a variety of studies that will improve the understanding of radiation impacts on the human brain at any stage of development and regeneration.
New accelerators such as the Facility for Antiproton and Ion Research (FAIR) in Darmstadt, Germany, that is to be completed in 2025, can produce heavy ion beams up to around 10 GeV/n as compared to 1 GeV/n with the current setting (reviewed in [101]). This is particularly relevant for ground-based studies of possible galactic cosmic radiation (GCR) effects, as part of the particle flux in the GCR exceeds 1 GeV/n [102]. However, very few studies to date have addressed high-energy ranges > 1 GeV/n. Meaningful risk assessment specifically of interplanetary missions therefore will rely on those state of the art accelerator facilities and brain organoids can serve as a high throughput biological risk model. While astronauts are subject to chronic irradiation, accelerator-based experiments normally use acute exposure due to beamtime constraints, even though long-term experiments are technically feasible. High beam intensities, delivered by facilities like FAIR, are also of interest for the use of ultra-high dose rate (FLASH) radiotherapy with protons or potentially heavy ions such as carbon. This experimental radiation modality can reduce neurotoxic effects in the healthy tissue by increasing the dose rate to ≥40 Gy/s. Even though the detailed mechanisms underlying the greater radioresistance are still unknown, acute oxygen depletion within the irradiated tissue or chromatin remodeling is discussed as possible cause for the increased effectiveness [103]. Here again, organoids serve as ideal models due to their innate oxygen gradient within their various layers from the hypoxic core to the normoxic outer layer of the cortical plate. An initial study of a first patient with subcutaneous T-cell lymphoma confirmed the benefits of FLASH radiation by demonstrating a complete response of the tumor and minimal normal tissue toxicities [104]. However, the remaining studies focus mainly on animal models, e.g. Montay-Gruel et al. [205] demonstrated that spatial memory of mice is preserved after 10 Gy FLASH-whole-brain irradiation with mean dose rates above 100 Gy/s, whereas 10 Gy whole-brain irradiation at a conventional dose rate (0.1 Gy/s) impairs spatial memory [105]. Due to the limited number of suitable accelerator facilities providing the necessary technology to perform FLASH irradiations [106] and the lack of suitable human biological models, studies regarding FLASH radiotherapy are rare and translation to the clinics remains a challenge. High throughput, organoid based studies could shed light on the molecular mechanisms of the observed effects, particularly the contribution of hypoxia, and overcome these challenges.
Of particular interest in radiation therapy is also the use of ions other than protons and carbon. Helium ions for instance are discussed to be more suitable than protons due to an improved RBE in the Bragg-Peak region and an improved oxygen enhancement ratio [107] and therefore may be especially beneficial for pediatric patients [108] in terms of long-term side effects particularly to the brain. Research accelerator facilities offer the possibility to test these rarely used ions and mixed ion modalities (proton, helium, oxygen, carbon) on their neurotoxic behavior and to ensure risk-free implementation into the clinics.
Another approach to improve radiotherapy outcome is the so-called spatially fractionated radiotherapy (SFRT), the inhomogeneous irradiation with a stack or grid of small radiation beams. Depending on the beam spot size, these are known as spatially fractionated mini- or microbeam SFRT. Photon SFRT with a spot size in the mm range was first introduced more than a century ago to reduce skin necrosis and is used clinically (in combination with conventional radiotherapy) for many years [109]. In contrast, microbeam SFRT is still in a pre-clinical stage. In animal models, extremely high doses of hundreds of Gy delivered by microbeam photon SFRT were tolerated well e.g., by rat brain [110] and spinal cord [111], raising hopes that spatially fractionated radiotherapy allows dose escalation and thus improved tumor control without severe normal tissue complications. Compared to photon SFRT, SFRT with protons, and potentially heavier accelerated ions, combines the advantages of SFRT and particle therapy (reviewed in [112]) and is well tolerated by brain tissue in a rat animal model [113]. Recent clinical proof-of-concept studies and constant technical improvements enable the transition of proton SFRT to clinical application [114–116]. Photon SFRT and especially proton or heavy ion SFRT have great therapeutic potential, allowing tumor dose escalation with good normal tissue tolerance. However, the biological mechanisms behind SFRT are not fully understood. In this rapidly developing field, human brain organoids, combining the advantages of a human brain architecture and a reliable in vitro system, can be a useful tool both in the exploration of new SFRT techniques as well as in the discovery of the biological mechanisms underlying SFRT.
Despite remaining challenges, brain organoids present the most realistic human in vitro brain model so far and have enormous potential to pave the way for new research findings in the field of radiation research. These model systems will allow meaningful research and improvements in the fields of cancer therapy (acute high dose exposure) and space radiation (chronic low dose exposure) protection while partly replacing and streamlining time-consuming and ethically controversial animal studies. Furthermore, in contrast to the clinical facilities, new research facilities such as FAIR offer the implementation of varying and more complex experimental designs due to more flexible changes in irradiation conditions. Combining both, state of the art biological models and state of the art accelerators will enable us to address issues in radiation biology in an unprecedented fashion.
CS: data acquisition and manuscript writing. CH, SR, and IS: data acquisition, manuscript writing, and review. MD: manuscript review. All authors contributed to the article and approved the submitted version.
This work was funded by the Bundesministerium für Bildung und Forschung (BMBF) within the Brain Radiation Assay project (02NUK049A).
The authors declare that the research was conducted in the absence of any commercial or financial relationships that could be construed as a potential conflict of interest.
The authors thank all the members of the working group for their valuable comments, helpful critiques, and discussions.
1. United Nations Scientific Committee on the Effects of Atomic Radiation. Sources and Effects of Ionizing Radiation. 1st ed. New York, NY: United Nations; 2010
2. Krämer M, Kraft G. Track structure and DNA damage. Adv Space Res. (1994) 14:151–9. doi: 10.1016/0273-1177(94)90465-0
3. Scoccianti S, Detti B, Gadda D, Greto D, Furfaro I, Meacci F, et al. Organs at risk in the brain and their dose-constraints in adults and in children: a radiation oncologist's guide for delineation in everyday practice. Radiother Oncol. (2015) 114:230–8. doi: 10.1016/j.radonc.2015.01.016
4. Soussain C, Ricard D, Fike JR, Mazeron J-J, Psimaras D, Delattre J-Y. CNS complications of radiotherapy and chemotherapy. Lancet. (2009) 374:1639–51. doi: 10.1016/S0140-6736(09)61299-X
5. Durante M, Cucinotta FA. Physical basis of radiation protection in space travel. Rev. Mod. Phys. (2011) 83:1245–81. doi: 10.1103/RevModPhys.83.1245
6. Duval K, Grover H, Han L-H, Mou Y, Pegoraro AF, Fredberg J, Chen Z. Modeling physiological events in 2D vs. 3D cell culture. Physiology (Bethesda). (2017) 32:266–77. doi: 10.1152/physiol.00036.2016
7. Verhaegen F, Granton P, Tryggestad E. Small animal radiotherapy research platforms. Phys Med Biol. (2011) 56:R55–83. doi: 10.1088/0031-9155/56/12/R01
8. Hodge RD, Bakken TE, Miller JA, Smith KA, Barkan ER, Graybuck LT, et al. Conserved cell types with divergent features in human versus mouse cortex. Nature. (2019) 573:61–8. doi: 10.1038/s41586-019-1506-7
9. Lancaster MA, Renner M, Martin C-A, Wenzel D, Bicknell LS, Hurles ME, et al. Cerebral organoids model human brain development and microcephaly. Nature. (2013) 501:373–9. doi: 10.1038/nature12517
10. Hladik D, Tapio S. Effects of ionizing radiation on the mammalian brain. Mutat Res. (2016) 770:219–30. doi: 10.1016/j.mrrev.2016.08.003
11. Crossen JR, Garwood D, Glatstein E, Neuwelt EA. Neurobehavioral sequelae of cranial irradiation in adults: a review of radiation-induced encephalopathy. J Clin Oncol. (1994) 12:627–42. doi: 10.1200/JCO.1994.12.3.627
12. Otake M, Schull WJ. Radiation-related small head sizes among prenatally exposed A-bomb survivors. Int J Radiat Biol. (1993) 63:255–70. doi: 10.1080/09553009314550341
13. Yoshimaru H, Otake M, Fujikoshi Y, Schull WJ. Effect on school performance of prenatal exposure to the Hiroshima atomic bomb. Nihon Eiseigaku Zasshi. (1991) 46:747–54. doi: 10.1265/jjh.46.747
14. Schull WJ, Otake M, Yoshimaru H. Effect on Intelligence Test Score of Prenatal Exposure to Ionizing Radiation in Hiroshima and Nagasaki. Hiroshima: Radiation Effects Research Foundation (1988).
15. Hall P, Adami H-O, Trichopoulos D, Pedersen NL, Lagiou P, Ekbom A, et al. Effect of low doses of ionising radiation in infancy on cognitive function in adulthood: Swedish population based cohort study. BMJ. (2004) 328:19. doi: 10.1136/bmj.328.7430.19
16. Meyers CA, Brown PD. Role and relevance of neurocognitive assessment in clinical trials of patients with CNS tumors. J Clin Oncol. (2006) 24:1305–9. doi: 10.1200/JCO.2005.04.6086
17. Roddy E, Mueller S. Late effects of treatment of pediatric central nervous system tumors. J Child Neurol. (2016) 31:237–54. doi: 10.1177/0883073815587944
18. Fouladi M, Gilger E, Kocak M, Wallace D, Buchanan G, Reeves C, et al. Intellectual and functional outcome of children 3 years old or younger who have CNS malignancies. J Clin Oncol. (2005) 23:7152–60. doi: 10.1200/JCO.2005.01.214
19. Armstrong CL, Shera DM, Lustig RA, Phillips PC. Phase measurement of cognitive impairment specific to radiotherapy. Int J Radiat Oncol Biol Phys. (2012) 83:e319–24. doi: 10.1016/j.ijrobp.2011.12.083
20. Durante M, Loeffler JS. Charged particles in radiation oncology. Nat Rev Clin Oncol. (2010) 7:37–43. doi: 10.1038/nrclinonc.2009.183
21. Kraft G. Tumor therapy with heavy charged particles. Prog Particle Nucl Phys. (2000) 45:S473–544. doi: 10.1016/S0146-6410(00)00112-5
22. Lawrence JH, Tobias CA, Born JL, Mccombs RK, Roberts JE, Anger HO, et al. Pituitary irradiation with high-energy proton beams: a preliminary report. Cancer Res. (1958) 18:121–34
23. Kitagawa A, Fujita T, Muramatsu M, Biri S, Drentje AG. Review on heavy ion radiotherapy facilities and related ion sources (invited). Rev Sci Instrum. (2010) 81:02B909. doi: 10.1063/1.3268510
24. Schulz-Ertner D, Nikoghosyan A, Thilmann C, Haberer T, Jäkel O, Karger C, et al. Results of carbon ion radiotherapy in 152 patients. Int J Radiat Oncol Biol Phys. (2004) 58:631–40. doi: 10.1016/j.ijrobp.2003.09.041
25. Kahalley LS, Peterson R, Ris MD, Janzen L, Okcu MF, Grosshans DR, et al. Superior intellectual outcomes after proton radiotherapy compared with photon radiotherapy for pediatric medulloblastoma. J Clin Oncol. (2020) 38:454–61. doi: 10.1200/JCO.19.01706
26. Kahalley LS, Ris MD, Grosshans DR, Okcu MF, Paulino AC, Chintagumpala M, et al. Comparing intelligence quotient change after treatment with proton versus photon radiation therapy for pediatric brain tumors. J Clin Oncol. (2016) 34:1043–9. doi: 10.1200/JCO.2015.62.1383
27. Cucinotta FA, Alp M, Sulzman FM, Wang M. Space radiation risks to the central nervous system. Life Sci Space Res. (2014) 2:54–69. doi: 10.1016/j.lssr.2014.06.003
28. Cucinotta FA, Durante M. Cancer risk from exposure to galactic cosmic rays: implications for space exploration by human beings. Lancet Oncol. (2006) 7:431–5. doi: 10.1016/S1470-2045(06)70695-7
29. Garrett-Bakelman FE, Darshi M, Green SJ, Gur RC, Lin L, Macias BR, et al. The NASA Twins Study: A multidimensional analysis of a year-long human spaceflight. Science. (2019) 364:eaau8650. doi: 10.1126/science.aau8650
30. Götz M, Nakafuku M, Petrik D. Neurogenesis in the developing and adult brain-similarities and key differences. Cold Spring Harb Perspect Biol. (2016) 8:a018853. doi: 10.1101/cshperspect.a018853
31. Doetsch F, Caillé I, Lim DA, García-Verdugo JM, Alvarez-Buylla A. Subventricular zone astrocytes are neural stem cells in the adult mammalian brain. Cell. (1999) 97:703–16. doi: 10.1016/S0092-8674(00)80783-7
32. Palmer TD, Takahashi J, Gage FH. The adult rat hippocampus contains primordial neural stem cells. Mol Cell Neurosci. (1997) 8:389–404. doi: 10.1006/mcne.1996.0595
33. Bergmann O, Spalding KL, Frisén J. Adult neurogenesis in humans. Cold Spring Harb Perspect Biol. (2015) 7:a018994. doi: 10.1101/cshperspect.a018994
34. Kempermann G, Gage FH, Aigner L, Song H, Curtis MA, Thuret S, et al. Human adult neurogenesis: evidence and remaining questions. Cell Stem Cell. (2018) 23:25–30. doi: 10.1016/j.stem.2018.04.004
35. Magnusson JP, Frisén J. Stars from the darkest night: unlocking the neurogenic potential of astrocytes in different brain regions. Development. (2016) 143:1075–86. doi: 10.1242/dev.133975
36. Reinhard J, Brösicke N, Theocharidis U, Faissner A. The extracellular matrix niche microenvironment of neural and cancer stem cells in the brain. Int J Biochem Cell Biol. (2016) 81:174–83. doi: 10.1016/j.biocel.2016.05.002
37. Gage FH. Mammalian neural stem cells. Science. (2000) 287:1433–8. doi: 10.1126/science.287.5457.1433
38. Yan Y, Shin S, Jha BS, Liu Q, Sheng J, Li F, et al. Efficient and rapid derivation of primitive neural stem cells and generation of brain subtype neurons from human pluripotent stem cells. Stem Cells Transl Med. (2013) 2:862–70. doi: 10.5966/sctm.2013-0080
39. Monni E, Congiu T, Massa D, Nat R, Diana A. Human neurospheres: from stained sections to three-dimensional assembly. Transl Neurosci. (2011) 2:43–8. doi: 10.2478/s13380-011-0007-4
40. Mayer M, Arrizabalaga O, Lieb F, Ciba M, Ritter S, Thielemann C. Electrophysiological investigation of human embryonic stem cell derived neurospheres using a novel spike detection algorithm. Biosens Bioelectron. (2018) 100:462–8. doi: 10.1016/j.bios.2017.09.034
41. Merz F, Müller M, Taucher-Scholz G, Rödel F, Stöcker H, Schopow K, et al. Tissue slice cultures from humans or rodents: a new tool to evaluate biological effects of heavy ions. Radiat Environ Biophys. (2010) 49:457–62. doi: 10.1007/s00411-010-0293-1
42. Perlman RL. Mouse models of human disease: An evolutionary perspective. Evol Med Public Health. (2016) 2016:170–6. doi: 10.1093/emph/eow014
43. Etienne O, Roque T, Haton C, Boussin FD. Variation of radiation-sensitivity of neural stem and progenitor cell populations within the developing mouse brain. Int J Radiat Biol. (2012) 88:694–702. doi: 10.3109/09553002.2012.710927
44. Acharya MM, Lan ML, Kan VH, Patel NH, Giedzinski E, Tseng BP, Limoli CL. Consequences of ionizing radiation-induced damage in human neural stem cells. Free Radic Biol Med. (2010) 49:1846–55. doi: 10.1016/j.freeradbiomed.2010.08.021
45. Katsura M, Cyou-Nakamine H, Zen Q, Zen Y, Nansai H, Amagasa S, et al. Effects of chronic low-dose radiation on human neural progenitor cells. Sci Rep. (2016) 6:20027. doi: 10.1038/srep20027
46. Yokota Y, Wada Y, Funayama T. Distinct modes of death in human neural stem and glioblastoma cells irradiated with carbon-ion radiation and gamma-rays. Int J Radiat Biol. (2020) 96:172–8. doi: 10.1080/09553002.2020.1683639
47. Isono M, Yoshida Y, Takahashi A, Oike T, Shibata A, Kubota Y, et al. Carbon-ion beams effectively induce growth inhibition and apoptosis in human neural stem cells compared with glioblastoma A172 cells. J Radiat Res. (2015) 56:856–61. doi: 10.1093/jrr/rrv033
48. Morini J, Babini G, Barbieri S, Baiocco G, Ciocca M, Ivaldi GB, et al. A comparison between X-ray and carbon ion irradiation in human neural stem cells. Radiat Prot Dosimetry. (2019) 183:102–6. doi: 10.1093/rpd/ncy231
49. Tseng BP, Giedzinski E, Izadi A, Suarez T, Lan ML, Tran KK, et al. Functional consequences of radiation-induced oxidative stress in cultured neural stem cells and the brain exposed to charged particle irradiation. Antioxid Redox Signal. (2014) 20:1410–22. doi: 10.1089/ars.2012.5134
50. Duman JG, Dinh J, Zhou W, Cham H, Mavratsas VC, Paveškovic M, et al. Memantine prevents acute radiation-induced toxicities at hippocampal excitatory synapses. Neuro Oncol. (2018) 20:655–65. doi: 10.1093/neuonc/nox203
51. Monje ML, Vogel H, Masek M, Ligon KL, Fisher PG, Palmer TD. Impaired human hippocampal neurogenesis after treatment for central nervous system malignancies. Ann Neurol. (2007) 62:515–20. doi: 10.1002/ana.21214
52. Menzel F, Kaiser N, Haehnel S, Rapp F, Patties I, Schöneberg N, et al. Impact of X-irradiation on microglia. Glia. (2018) 66:15–33. doi: 10.1002/glia.23239
53. Merz F, Gaunitz F, Dehghani F, Renner C, Meixensberger J, Gutenberg A, et al. Organotypic slice cultures of human glioblastoma reveal different susceptibilities to treatments. Neuro Oncol. (2013) 15:670–81. doi: 10.1093/neuonc/not003
54. Hanbury DB, Peiffer AM, Dugan G, Andrews RN, Cline JM. Long-term cognitive functioning in single-dose total-body gamma-irradiated rhesus monkeys (Macaca mulatta). Radiat Res. (2016) 186:447–54. doi: 10.1667/RR14430.1
55. Raber J, Rola R, LeFevour A, Morhardt D, Curley J, Mizumatsu S, et al. Radiation-induced cognitive impairments are associated with changes in indicators of hippocampal neurogenesis. Radiat Res. (2004) 162:39–47. doi: 10.1667/RR3206
56. Monje ML, Mizumatsu S, Fike JR, Palmer TD. Irradiation induces neural precursor-cell dysfunction. Nat Med. (2002) 8:955–62. doi: 10.1038/nm749
57. Mizumatsu S, Monje ML, Morhardt DR, Rola R, Palmer TD, Fike JR. Extreme sensitivity of adult neurogenesis to low doses of X-irradiation. Cancer Res. (2003) 63:4021–7.
58. Hellström NAK, Björk-Eriksson T, Blomgren K, Kuhn HG. Differential recovery of neural stem cells in the subventricular zone and dentate gyrus after ionizing radiation. Stem Cells. (2009) 27:634–41. doi: 10.1634/stemcells.2008-0732
59. Panagiotakos G, Alshamy G, Chan B, Abrams R, Greenberg E, Saxena A, et al. Long-term impact of radiation on the stem cell and oligodendrocyte precursors in the brain. PLoS ONE. (2007) 2:e588. doi: 10.1371/journal.pone.0000588
60. Britten RA, Davis LK, Johnson AM, Keeney S, Siegel A, Sanford LD, et al. Low (20 cGy) doses of 1 GeV/u (56)Fe—particle radiation lead to a persistent reduction in the spatial learning ability of rats. Radiat Res. (2012) 177:146–51. doi: 10.1667/rr2637.1
61. Shukitt-Hale B, Casadesus G, McEwen JJ, Rabin BM, Joseph JA. Spatial learning and memory deficits induced by exposure to iron-56-particle radiation. Radiat Res. (2000) 154:28–33. doi: 10.1667/0033-7587(2000)154[0028:slamdi]2.0.co;2
62. Denisova NA, Shukitt-Hale B, Rabin BM, Joseph JA. Brain signaling and behavioral responses induced by exposure to 56 Fe-particle radiation. Radiat Res. (2002) 158:725–34. doi: 10.1667/0033-7587(2002)158[0725:BSABRI]2.0.CO;2
63. Rola R, Fishman K, Baure J, Rosi S, Lamborn KR, Obenaus A, et al. Hippocampal neurogenesis and neuroinflammation after cranial irradiation with (56)Fe particles. Radiat Res. (2008) 169:626–32. doi: 10.1667/RR1263.1
64. Lonart G, Parris B, Johnson AM, Miles S, Sanford LD, Singletary SJ, Britten RA. Executive function in rats is impaired by low (20 cGy) doses of 1 GeV/u (56)Fe particles. Radiat Res. (2012) 178:289–94. doi: 10.1667/RR2862.1
65. Haley GE, Yeiser L, Olsen RHJ, Davis MJ, Johnson LA, Raber J. Early effects of whole-body (56)Fe irradiation on hippocampal function in C57BL/6J mice. Radiat Res. (2013) 179:590–6. doi: 10.1667/RR2946.1
66. Sweet TB, Panda N, Hein AM, Das SL, Hurley SD, Olschowka JA, et al. Central nervous system effects of whole-body proton irradiation. Radiat Res. (2014) 182:18–34. doi: 10.1667/RR13699.1
67. Parihar VK, Pasha J, Tran KK, Craver BM, Acharya MM, Limoli CL. Persistent changes in neuronal structure and synaptic plasticity caused by proton irradiation. Brain Struct Funct. (2015) 220:1161–71. doi: 10.1007/s00429-014-0709-9
68. Seegenschmiedt MH, Micke O, Muecke R. Radiotherapy for non-malignant disorders: state of the art and update of the evidence-based practice guidelines. Br J Radiol. (2015) 88:20150080. doi: 10.1259/bjr.20150080
69. Reinhold HS, Calvo W, Hopewell JW, van den Berg AP. Development of blood vessel-related radiation damage in the fimbria of the central nervous system. Int J Radiat Oncol Biol Phys. (1990) 18:37–42. doi: 10.1016/0360-3016(90)90264-K
71. Jensen JB, Parmar M. Strengths and limitations of the neurosphere culture system. Mol Neurobiol. (2006) 34:153–62. doi: 10.1385/MN:34:3:153
72. Qi X-R, Verwer RWH, Bao A-M, Balesar RA, Luchetti S, Zhou J-N, Swaab DF. Human brain slice culture: a useful tool to study brain disorders and potential therapeutic compounds. Neurosci Bull. (2019) 35:244–52. doi: 10.1007/s12264-018-0328-1
73. Verwer RWH, Hermens WTJMC, Dijkhuizen P, ter Brake O, Baker RE, Salehi A, et al. Cells in human postmortem brain tissue slices remain alive for several weeks in culture. FASEB J. (2002) 16:54–60. doi: 10.1096/fj.01-0504com
74. Lui JH, Hansen DV, Kriegstein AR. Development and evolution of the human neocortex. Cell. (2011) 146:18–36. doi: 10.1016/j.cell.2011.06.030
75. Fish JL, Dehay C, Kennedy H, Huttner WB. Making bigger brains-the evolution of neural-progenitor-cell division. J Cell Sci. (2008) 121:2783–93. doi: 10.1242/jcs.023465
76. Wang X, Tsai J-W, LaMonica B, Kriegstein AR. A new subtype of progenitor cell in the mouse embryonic neocortex. Nat Neurosci. (2011) 14:555–61. doi: 10.1038/nn.2807
77. Di Lullo E, Kriegstein AR. The use of brain organoids to investigate neural development and disease. Nat Rev Neurosci. (2017) 18:573–84. doi: 10.1038/nrn.2017.107
78. Wang H. Modeling Neurological Diseases With Human Brain Organoids. Front Synaptic Neurosci. (2018) 10:15. doi: 10.3389/fnsyn.2018.00015
79. Lancaster MA, Knoblich JA. Generation of cerebral organoids from human pluripotent stem cells. Nat Protoc. (2014) 9:2329–40. doi: 10.1038/nprot.2014.158
80. Sakaguchi H, Kadoshima T, Soen M, Narii N, Ishida Y, Ohgushi M, et al. Generation of functional hippocampal neurons from self-organizing human embryonic stem cell-derived dorsomedial telencephalic tissue. Nat Commun. (2015) 6:8896. doi: 10.1038/ncomms9896
81. Kadoshima T, Sakaguchi H, Nakano T, Soen M, Ando S, Eiraku M, Sasai Y. Self-organization of axial polarity, inside-out layer pattern, and species-specific progenitor dynamics in human ES cell-derived neocortex. Proc Natl Acad Sci USA. (2013) 110:20284–9. doi: 10.1073/pnas.1315710110
82. Jo J, Xiao Y, Sun AX, Cukuroglu E, Tran H-D, Göke J, et al. Midbrain-like organoids from human pluripotent stem cells contain functional dopaminergic and neuromelanin-producing neurons. Cell Stem Cell. (2016) 19:248–57. doi: 10.1016/j.stem.2016.07.005
83. Monzel AS, Smits LM, Hemmer K, Hachi S, Moreno EL, van Wuellen T, et al. Derivation of human midbrain-specific organoids from neuroepithelial stem cells. Stem Cell Reports. (2017) 8:1144–54. doi: 10.1016/j.stemcr.2017.03.010
84. Qian X, Nguyen HN, Song MM, Hadiono C, Ogden SC, Hammack C, et al. Brain-region-specific organoids using mini-bioreactors for modeling ZIKV exposure. Cell. (2016) 165:1238–54. doi: 10.1016/j.cell.2016.04.032
85. Muguruma K, Nishiyama A, Kawakami H, Hashimoto K, Sasai Y. Self-organization of polarized cerebellar tissue in 3D culture of human pluripotent stem cells. Cell Rep. (2015) 10:537–50. doi: 10.1016/j.celrep.2014.12.051
86. Suga H, Kadoshima T, Minaguchi M, Ohgushi M, Soen M, Nakano T, et al. Self-formation of functional adenohypophysis in three-dimensional culture. Nature. (2011) 480:57–62. doi: 10.1038/nature10637
87. Paşca AM, Sloan SA, Clarke LE, Tian Y, Makinson CD, Huber N, et al. Functional cortical neurons and astrocytes from human pluripotent stem cells in 3D culture. Nat Methods. (2015) 12:671–8. doi: 10.1038/nmeth.3415
88. Quadrato G, Nguyen T, Macosko EZ, Sherwood JL, Min Yang S, Berger DR, et al. Cell diversity and network dynamics in photosensitive human brain organoids. Nature. (2017) 545:48–53. doi: 10.1038/nature22047
89. Sakaguchi H, Ozaki Y, Ashida T, Matsubara T, Oishi N, Kihara S, Takahashi J. Self-organized synchronous calcium transients in a cultured human neural network derived from cerebral organoids. Stem Cell Rep. (2019) 13:458–73. doi: 10.1016/j.stemcr.2019.05.029
90. Bagley JA, Reumann D, Bian S, Lévi-Strauss J, Knoblich JA. Fused cerebral organoids model interactions between brain regions. Nat Methods. (2017) 14:743–51. doi: 10.1038/nmeth.4304
91. Birey F, Andersen J, Makinson CD, Islam S, Wei W, Huber N, et al. Assembly of functionally integrated human forebrain spheroids. Nature. (2017) 545:54–9. doi: 10.1038/nature22330
92. Song L, Yuan X, Jones Z, Griffin K, Zhou Y, Ma T, Li Y. Assembly of human stem cell-derived cortical spheroids and vascular spheroids to model 3-D brain-like tissues. Sci Rep. (2019) 9:5977. doi: 10.1038/s41598-019-42439-9
93. Xiang Y, Tanaka Y, Patterson B, Kang Y-J, Govindaiah G, Roselaar N, et al. Fusion of regionally specified hPSC-derived organoids models human brain development and interneuron migration. Cell Stem Cell. (2017) 21:383–98.e7. doi: 10.1016/j.stem.2017.07.007
94. Lin Y-T, Seo J, Gao F, Feldman HM, Wen H-L, Penney J, et al. APOE4 Causes widespread molecular and cellular alterations associated with Alzheimer's disease phenotypes in human iPSC-derived brain cell types. Neuron. (2018) 98:1141–54.e7. doi: 10.1016/j.neuron.2018.05.008
95. Ormel PR, Vieira de Sá R, van Bodegraven EJ, Karst H, Harschnitz O, Sneeboer MAM, et al. Microglia innately develop within cerebral organoids. Nat Commun. (2018) 9:4167. doi: 10.1038/s41467-018-06684-2
96. Bian S, Repic M, Guo Z, Kavirayani A, Burkard T, Bagley JA, et al. Genetically engineered cerebral organoids model brain tumor formation. Nat Methods. (2018) 15:631–9. doi: 10.1038/s41592-018-0070-7
97. Mattei C, Alshawaf A, D'Abaco G, Nayagam B, Dottori M. Generation of neural organoids from human embryonic stem cells using the rotary cell culture system: effects of microgravity on neural progenitor cell fate. Stem Cells Dev. (2018) 27:848–57. doi: 10.1089/scd.2018.0012
98. Giandomenico SL, Mierau SB, Gibbons GM, Wenger LMD, Masullo L, Sit T, et al. Cerebral organoids at the air-liquid interface generate diverse nerve tracts with functional output. Nat Neurosci. (2019) 22:669–79. doi: 10.1038/s41593-019-0350-2
99. Marton RM, Miura Y, Sloan SA, Li Q, Revah O, Levy RJ, et al. Differentiation and maturation of oligodendrocytes in human three-dimensional neural cultures. Nat Neurosci. (2019) 22:484–91. doi: 10.1038/s41593-018-0316-9
100. Lancaster MA, Corsini NS, Wolfinger S, Gustafson EH, Phillips AW, Burkard TR, et al. Guided self-organization and cortical plate formation in human brain organoids. Nat Biotechnol. (2017) 35:659–66. doi: 10.1038/nbt.3906
101. Durante M, Golubev A, Park W-Y, Trautmann C. Applied nuclear physics at the new high-energy particle accelerator facilities. Phys Rep. (2019) 800:1–37. doi: 10.1016/j.physrep.2019.01.004
102. NCRP. Information Needed to Make Radiation Protection Recommendations for Space Missions Beyond Low-Earth Orbit: Recommendations of the National Council on Radiation Protection and Measurements. Bethesda, MD: National Council on Radiation Protection and Measurements (2006).
103. Durante M, Bräuer-Krisch E, Hill M. Faster and safer? FLASH ultra-high dose rate in radiotherapy. Br J Radiol. (2018) 91:20170628. doi: 10.1259/bjr.20170628
104. Bourhis J, Sozzi WJ, Jorge PG, Gaide O, Bailat C, Duclos F, et al. Treatment of a first patient with FLASH-radiotherapy. Radiother Oncol. (2019) 139:18–22. doi: 10.1016/j.radonc.2019.06.019
105. Montay-Gruel P, Petersson K, Jaccard M, Boivin G, Germond J-F, Petit B, et al. Irradiation in a flash: Unique sparing of memory in mice after whole brain irradiation with dose rates above 100Gy/s. Radiother Oncol. (2017) 124:365–9. doi: 10.1016/j.radonc.2017.05.003
106. Wilson JD, Hammond EM, Higgins GS, Petersson K. Ultra-high dose rate (FLASH) radiotherapy: silver bullet or fool's gold? Front Oncol. (2019) 9:1563. doi: 10.3389/fonc.2019.01563
107. Tessonnier T, Mairani A, Chen W, Sala P, Cerutti F, Ferrari A, et al. Proton and helium ion radiotherapy for meningioma tumors: a Monte Carlo-based treatment planning comparison. Radiat Oncol. (2018) 13:2. doi: 10.1186/s13014-017-0944-3
108. Knäusl B, Fuchs H, Dieckmann K, Georg D. Can particle beam therapy be improved using helium ions? A planning study focusing on pediatric patients. Acta Oncol. (2016) 55:751–9. doi: 10.3109/0284186X.2015.1125016
109. Mohiuddin M, Fujita M, Regine WF, Megooni AS, Ibbott GS, Ahmed MM. High-dose spatially-fractionated radiation (GRID): a new paradigm in the management of advanced cancers. Int J Radiat Oncol Biol Phys. (1999) 45:721–7. doi: 10.1016/S0360-3016(99)00170-4
110. Slatkin DN, Spanne P, Dilmanian FA, Gebbers JO, Laissue JA. Subacute neuropathological effects of microplanar beams of x-rays from a synchrotron wiggler. Proc Natl Acad Sci USA. (1995) 92:8783–7. doi: 10.1073/pnas.92.19.8783
111. Dilmanian FA, Zhong Z, Bacarian T, Benveniste H, Romanelli P, Wang R, et al. Interlaced x-ray microplanar beams: a radiosurgery approach with clinical potential. Proc Natl Acad Sci USA. (2006) 103:9709–14. doi: 10.1073/pnas.0603567103
112. Meyer J, Eley J, Schmid TE, Combs SE, Dendale R, Prezado Y. Spatially fractionated proton minibeams. Br J Radiol. (2019) 92:20180466. doi: 10.1259/bjr.20180466
113. Prezado Y, Jouvion G, Hardy D, Patriarca A, Nauraye C, Bergs J, et al. Proton minibeam radiation therapy spares normal rat brain: long-term clinical, radiological and histopathological analysis. Sci Rep. (2017) 7:14403. doi: 10.1038/s41598-017-14786-y
114. Henry T, Ureba A, Valdman A, Siegbahn A. Proton grid therapy. Technol Cancer Res Treat. (2017) 16:749–57. doi: 10.1177/1533034616681670
115. Lansonneur P, Mammar H, Nauraye C, Patriarca A, Hierso E, Dendale R, et al. First proton minibeam radiation therapy treatment plan evaluation. Sci Rep. (2020) 10:7025. doi: 10.1038/s41598-020-63975-9
Keywords: ionizing radiation, brain, neurotoxicity, X-rays, heavy ions, radiotherapy, space research, brain organoids
Citation: Schielke C, Hartel C, Durante M, Ritter S and Schroeder IS (2020) Solving the Issue of Ionizing Radiation Induced Neurotoxicity by Using Novel Cell Models and State of the Art Accelerator Facilities. Front. Phys. 8:568027. doi: 10.3389/fphy.2020.568027
Received: 01 June 2020; Accepted: 24 August 2020;
Published: 30 September 2020.
Edited by:
Udo Jochen Birk, University of Applied Sciences Graubünden, SwitzerlandReviewed by:
Lembit Sihver, Vienna University of Technology, AustriaCopyright © 2020 Schielke, Hartel, Durante, Ritter and Schroeder. This is an open-access article distributed under the terms of the Creative Commons Attribution License (CC BY). The use, distribution or reproduction in other forums is permitted, provided the original author(s) and the copyright owner(s) are credited and that the original publication in this journal is cited, in accordance with accepted academic practice. No use, distribution or reproduction is permitted which does not comply with these terms.
*Correspondence: Insa S. Schroeder, aS5zY2hyb2VkZXJAZ3NpLmRl
Disclaimer: All claims expressed in this article are solely those of the authors and do not necessarily represent those of their affiliated organizations, or those of the publisher, the editors and the reviewers. Any product that may be evaluated in this article or claim that may be made by its manufacturer is not guaranteed or endorsed by the publisher.
Research integrity at Frontiers
Learn more about the work of our research integrity team to safeguard the quality of each article we publish.