- Institut de Minéralogie, Physique des Matériaux et Cosmochimie, Sorbonne Université, CNRS, Paris, France
While the field of noble gas reactivity essentially belongs to chemistry, Earth and planetary sciences have brought a different perspective to the field. Indeed, planetary interiors are natural high pressure (P) and high temperature (T) laboratories, where conditions exist where bonding of the heaviest noble gases may be induced thermodynamically through volume reduction (Le Châtelier's principle). Earth and planetary sciences besides generate numerous and precise observations such as the depletion of the terrestrial and martian atmospheres in xenon, pointing to the potential for Xe to be sequestered at depth, potentially induced by its reactivity. More generally, this paper will review the advances on noble gas reactivity at the extreme P-T conditions found within planetary interiors from experiments and theoretical investigations. This review will cover the synthesis of cage compounds, stoichiometric oxides and metals, and non-stoichiometric compounds where noble gases are only minor or trace elements but could be essential in solving some Earth and planetary puzzles. An apparent trend in noble gas reactivity with P emerges. In the case of Xe which is the most documented, metals are synthesized above 150 GPa, i.e., at terrestrial core conditions, stoichiometric Xe-oxides between 50 and 100 GPa, i.e., in the P-T range of the Earth's lower mantle, but Xe-O high energy bonds may also form under the modest pressures of the Earth's crust (<1 GPa) in non-stoichiometric compounds. Most planetary relevant noble gas compounds found are with xenon, with only a few predicted helium compounds, the latter having no or very little charge transfer between helium and neighboring atoms.
1. Introduction
The inertness of noble gases is the cornerstone of noble gas geochemistry. Helium (He), neon (Ne), argon (Ar), krypton (Kr), and xenon (Xe) are all important markers of a variety of planetary processes, ranging from Earth's accretion, degassing of the atmosphere, vigor of mantle convection, to tracking underground nuclear tests. Noble gas abundances and isotopic ratios have thus been measured in a large variety of samples (atmosphere, fluids, rocks) and from different geological contexts, from Archean rocks over 2.5 Gy old [1, 2] to Martian meteorites [3] and comets [4]. Besides the Earth and Mars, the only other planetary atmosphere probed so far is that of Jupiter [5]. Future missions are targeted at measuring noble gases in other atmospheres with pre-selected NASA DAVINCI+ mission to Venus and SPRITE mission to Saturn, noble gases being presented as critical to understanding the formation and evolution of giant planets.
From these measurements, planetary accretion reservoirs and processes may be traced. Only Ne has preserved a solar signature in some terrestrial samples, a signature interpreted as a proof for the existence of a deep magma ocean (i.e., partially or fully molten Earth in equilibrium with a primary solar-like atmosphere) within the first hundred My of the Earth's formation [6, 7]. Heavier Kr and Xe noble gases overall have a chondritic signature in terrestrial and martian samples, with a minor cometary contribution [4, 8]. Jupiter is the only other planet who's atmosphere has been studied in detail. It is slightly depleted in He, strongly in Ne [5], and enriched by a factor of 2.5–2.7 in heavy noble gases (Ar, Kr, Xe) compared to solar abundances [9], as expected from trapping in amorphous ice and pointing to a major cometary contribution to Jupiter.
Noble gases are also widely used to trace planetary processes. For instance, the iodine-plutonium-xenon system is used to date the formation of the atmosphere [10, 11] due to the very short half-lifes of 129I (17 My) and 244Pu (82 My). Helium and Ar isotopes constrain mantle degassing and convection over much longer time scales [12–14] owing to the longer half-life of their parent isotopes (for instance 1.25 Ga for 40K). None of Kr stable isotopes is purely radiogenic, preventing its use to constrain chronology of the atmosphere evolution. It was pointed out as early as 1970 that Xe is under abundant in the atmospheres of the Earth and Mars relative to the chondritic abundance pattern [15], a depletion of up to 90% [10] and known as the “missing Xe” problem. Atmospheric Xe is furthermore strongly depleted in light isotopes [16], and this depletion in light Xe isotopes was progressive throughout the Archean [17]. The case is more debated for Ar, but the classical double-layered convection model [12] precisely required two separated layers in the mantle on the basis that an unsampled reservoir, such as the lower mantle, must be enriched in Ar to satisfy radiogenic 40Ar mass balance. Seismic tomography later showed that there is a mass flow from the upper to the lower mantle, but the need for an Ar-rich reservoir remains.
The most striking fact emerging from the current data set of noble gas analyses in natural samples remains the “missing Xe.” This paradox has launched a search for either ways to lose Xe to space [17–20] or to trap Xe at depth. The interiors of the Earth are indeed characterized by extreme P-T conditions, with a six-order magnitude increase in P and 3-order magnitude increase in T between the surface (105 Pa–287 K) and the core of the planet (365 GPa–5,000 K). Such conditions may induce chemical reactivity as negative reaction volume change under increased P may overcome enthalpic effects of otherwise prohibited reactions at ambient P. Natural samples do indicate higher Xe retention in particular contexts. Xe concentrations in near surface rocks are ~0.05 ppt (part per trillion) in basalts [21] and up to 0.2 ppb (part per billion) in deep sea siliceous fossils [22]. The latter samples have 132Xe/36Ar ratios of up to 0.05, i.e., about thirty times higher than in mid-ocean ridge basalts [23, 24]. Xe enrichment over other noble gases is also reported in high P contexts such as mantle xenoliths exhumed by volcanism [25–28] and impact craters [29] by two orders of magnitude with the highest excess (132Xe/36Ar = 0.170) being reported in excavated lunar crustal rocks [30].
The initiation of high P solid-state chemistry of Xe was triggered by the discovery of its metallization at 135 GPa [31], yet the potential link to the missing Xe paradox took a decade to be established. Since then, there has been a continuous feedback between high P mineral physics and condensed matter physics in the search for new noble gas compounds. Foreseen as promising by Grochala [32] but then still experimentally unexplored, the high P chemistry of noble gases now extends to several atoms, an effort largely led by the perspective to understand noble gas reactivity at deep Earth's conditions. Interestingly, noble gases are archetypical pressure-transmitting media used in laser heated diamond-anvil cell experiments to reproduce hydrostatic deep Earth's conditions in an otherwise rather uniaxial compression apparatus, and thermally insulate the sample from the diamond anvils. This technical aspect has doubtless been a positive factor in elucidating some of the high P noble gas reactivity reviewed here, and that covers first retention of noble gases in rings, channels and cage structures, second noble gas oxides, third noble gas compounds with metals, and finally other types of high P noble gas compounds relevant of planetary interiors.
2. Retention of Noble Gases in Ring, Channel, and Cage Structures
2.1. Clathrates and Other Cage Compounds
Water ice is one of the most prevalent substances in the Solar System, with the majority of it existing at high P and T conditions in the interiors of giant planets. While clathrates hydrates have long been considered as carriers of nobles gases to the giant planets, a consensus emerges now for simple adsorption on amorphous ice [9]. On Earth, clathrate hydrates are found in pergelisols and oceanic margins. Noble gases are among the gases that stabilize clathrate hydrate structures through van der Waals interactions. Ar6·H2O was actually the first compound of a noble gas discovered in Villard [33]. All noble gases form clathrates by combination with water, with P-T stability field extending from He to Xe [34]. However, even Xe hydrates are stable only up to 2.5 GPa [35].
Cyclo-silicates can host large amount of noble gases in the channels and ring sites of their crystal structures. These minerals are not occurrences of noble gas reactivity at depth, but are essential carriers of noble gas recycling via subduction of lithospheric plates [36, 37], from the surface reservoirs (atmosphere, oceans) to the deep Earth where reactions may take place. Natural occurrences of He and Ar in cyclo-silicates are abnormally high [38]. He, Ne and Ar occupy ring sites in amphibole [37], a mineral formed in the altered oceanic crust, and experimentally measured solubilities are up to four orders of magnitude higher than for other silicates at similar run P (0.17 GPa). Ring sites are formed by a pair of opposing six-membered (Si,Al) tetrahedra rings, with a ring inner diameter circa 5 Å. Noble gases sitting in these sites are thus surrounded by 12 neighboring oxygens at approximately 2.5 Å. Kr and Xe solubilities have not been investigated in amphibole, but are even higher than Ar solubility in serpentine (×2 for Kr, ×10 for Xe), another important mineral from the altered oceanic crust [39].
The very first attempts at testing Xe reactivity with silica [40] were guided by the fact that SiO2 high T phase cristobalite has large cages that could trap Xe atoms and favor chemical reactivity. However, when SiO2 was loaded as cristobalite, it systematically transformed to quartz, with the latter efficiently trapping Xe (cf. section 3). Xenon, far form stabilizing cristobalite, in fact destabilizes it. Instead, He proved much more interesting in this respect as cristobalite loaded in He as a diamond-anvil cell P-transmitting medium was shown to convert to He-cristobalite at 8 GPa instead of transforming to cristobalite-II at 1.6 GPa [41], its structure and stoichiometry (SiO2He) were solved (Figure 2).
2.2. Stuffed Amorphous Silicates
Noble gas solubility in molten silicates depend on their composition. In fact, noble gas solubility in vitreous silica has been used to characterize glass network geometry [44]. Noble gas retention in silicate melts has long been postulated as occurring by insertion in the interstitial voids [45], with the abundance depending on the melt's polymerization, itself constrained by the SiO2 content as tetrahedra polymerize as rings (see below). Silicate melts may accommodate larger amounts of dissolved noble gases under increased P until solubility reaches a plateau circa 5 GPa with for instance a maximum of 3 mol% Ar in silica-rich melts [46, 47]. Note that solubility drops at higher P were reported [48, 49] but these results were not reproduced [50], possibly due to incomplete melting of the samples at higher P in the first studies.
Noble gases affect the behavior of non-crystalline silicates under pressure. Up to 1–2 mol% He may dissolve into the interstitial voids in SiO2 glass under pressure [51, 52], resulting in a much less compressible glass [52], with a spatial scale of the medium-range order almost independent on P compared to SiO2 glass compressed in solid P-medium or compressed in H2. Similarly to He in compressed SiO2 glass, Xe was also found to affect the medium-range order with a sharpening of the first sharp diffraction peak on x-ray diffraction data, indicative of a stronger medium-range order [53]. More importantly, Xe-O bonds with a bond length of 2.05–2.10 Å and a coordination number of 12 were reported, indicative of the Xe insertion in six-membered rings (i.e., rings formed of six tetrahedra) rather than in interstitial voids. This ring structure of molten and glassy silicates is similar to that of cyclo-silicates, except that there is a statistical distribution of ring sizes, mostly from 4 to 10 tetrahedra depending on melt composition [54]. This distribution has been recently quantified in vitreous silica by neutron diffraction [55], the six-membered ring being the most prominent and having an inner diameter of 4.30 Å, consistent with the Xe-O bond length reported above. Krypton local environment in compressed melts, as investigated using x-ray absorption spectroscopy [56], is a shell of oxygen atoms located at 2.49 Å. This relatively short distance indicates some degree of covalency albeit less than for Xe-O bonds. It is however difficult to reach further conclusions, as the coordination number could not be calculated due to the lack of Kr oxide references that are necessary to process x-ray absorption spectroscopy data.
3. Noble Gas Oxides
The mineralogical constituents of the crust and mantle of terrestrial planets are silicates, with a predominance of framework silicates in the continental crust such as quartz and feldspars, and a predominance of olivine in the mantle that undergo phase transitions at depth, and decomposes into bridgmanite and magnesiowustite below 660 km. First steps toward establishing noble gas reactivity in planetary crust and mantle have been the synthesis of noble gas oxides, keeping in mind that it can only be firmly established between silicates and noble gases as trace elements for realistic implications to planetary interiors.
3.1. Stoichiometric Oxides
Xenon oxides have been known since the sixties with the synthesis of tetrahedral forms (XeO3 by Templeton et al. [57], XeO4 by Huston et al. [58]) by hydrolysis of xenon fluorides at cryogenic T, and more recently with the synthesis of XeO2 that has a different geometry with local square planar oxygen environment [59]. Although the local structure of XeO2 is square planar, Xe being bonded to four oxygens, its full crystallographic structure has not yet been resolved.
The first Xe oxide experimentally synthesized by use of high P was Xe2O6H6 (Figure 1), obtained by reaction between superionic ice and Xe above 50 GPa and 1,500 K upon laser heating in diamond-anvil cells [60], with platinum used as a laser coupler. Superionic ice is a high P-T phase forming at 50 GPa and characterized by a full mobility of the hydrogen atoms [62], while the oxygen lattice is identical to that of lower T phase X. Xe2O6H6 is metallic due to the Xe and O atoms, however the diffusivity of hydrogen atoms is similar to that of superionic ice. Pure Xe oxides were predicted to be stable against decomposition above 83 GPa by ab initio calculations: XeO, XeO2, and XeO3 [63], followed by Xe2O3 and more Xe-rich phases at even higher P [64]. Only Xe2O3 was observed experimentally albeit at slightly higher P (87 GPa instead of the predicted 75 GPa), along with Xe2O5 at 83 GPa [61]. Interesting fact: Xe may exist at different oxidation states in the same structure. That Xe oxidation is induced at significantly lower P with superionic ice compared to pure oxygen could be due to the reactivity of the very diffusive hydrogen with platinum, the formation of platinum hydride contributing to a larger volume reduction of the global reaction. In addition, the oxygen and xenon sublattices in Xe2O6H6 bears remarkable resemblance to those of η-O2 [65] and pure Xe, both having hexagonal closed-packed structures and the η-O2 being characterized by a high degree of charge transfer.
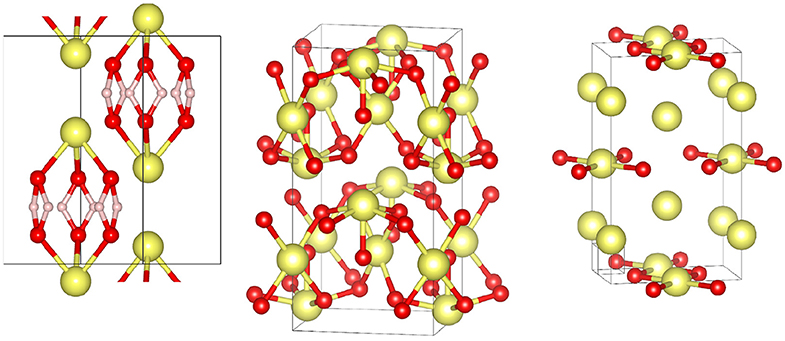
Figure 1. Experimentally observed and theoretically predicted xenon oxides. From left to right: Xe2O6H6 [60], Xe2O5, Xe2O3 [61]. Color code for atoms: xenon (yellow), oxygen (red), hydrogen atoms (light pink).
For krypton, only KrO has been predicted to be stable against decomposition above 300 GPa [66], and no Kr-compound has been synthesized experimentally. No argon oxide nor neon oxide have yet been documented to form at the conditions of planetary interiors, despite the visionary editorial by Abelson [67] citing ArH4 and ArO6 as examples of P-induced chemistry. But surprisingly, one helium oxide has been reported. On the basis of ab initio calculations, FeO2He, is predicted to be stable at core-mantle boundary conditions [68]. Unlike Xe-oxides and KrO discussed above, there is almost no charge transfer from He to surrounding atoms. Excess primordial He (i.e., 3He, while 4He has been produced throughout Earth's history by radioactive decay) are found in some basalts which rock source is identified as originating from very deep in the mantle [69]. Thermochemical piles are domes of both thermal and chemical origin in the deep mantle, rooted above the core-mantle boundary below central Pacific and south Atlantic-Africa [70], with locally partially molten zones (ultra-low velocity zones) at their very base. These piles might have been preserved during a large part if not all of Earth's history and could indeed by He-rich, providing a reasonable context for the formation of FeO2He. Besides, hydrogen bearing iron peroxide FeO2H [71] is also stable at the corresponding P-T conditions, or as partially dehydrogenated FeOOHx postulated as an important component of the ultra-low velocity zones [72]. Both FeO2He and FeO2H have a cubic structure with identical cell parameter of, respectively 4.32 Å at 135 GPa [68] and 4.33 Å at 133.5 GPa [73], but with different symmetries, Fm-3m vs. Pa-3.
3.2. Xenon as a Minor/Trace Element in Oxides
That Xe reacts with oxides at the conditions of the deep crust and mantle is a strong indication that it can be stored at depth. However, Xe is a trace element in planetary interiors (cf. section 1), therefore the relevant reactions are not those producing stoichiometric compounds but those where Xe retention occurs as a trace or minor element. This aspect considerably changes the energetics of the reaction, and consequently the P-T conditions at which they may occur. Xe trapping in the deep crust and mantle could solve the Xe paradox, and this occurs by Xe substitution to Si (cf. Figure 2) whereby Xe gets oxidized such as in hot compressed SiO2 quartz [74] or (Mg,Fe)2SiO4 olivine [75]. Xe-O bonds are best evidenced by new Raman and infra-red vibrational modes (cf. Figure 3) that appear under P upon heating above the melting curve of Xe, the reaction being thermodynamically favored by the volume reduction between reactants (i.e., liquid Xe and quartz or olivine) and products (i.e., Xe-doped quartz or Xe-doped olivine). Theoretical calculations have confirmed this mechanism for quartz [43, 76, 77] and olivine [42], and helped refine the crystal-chemistry of Xe in these minerals. Volume and cell-parameters vs. P relationships as well as Raman signature of Xe-doped silicates could be theoretically reproduced by substituting Xe to Si albeit in different geometries depending on the mineral: quasi-planar three-fold in olivine vs. linear two-fold (2 nearest O atoms at 1.99 Å) with 2 next nearest O neighbors in orthogonal plan at 2.27 Å in quartz [43]. Theoretically-derived solubility is up to 0.4 at% Xe in both phases [42, 43].
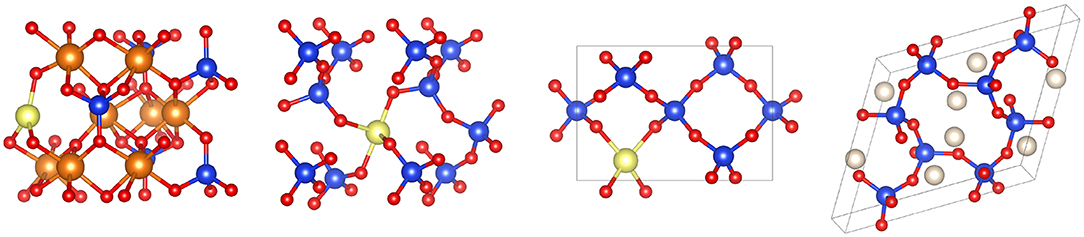
Figure 2. Experimentally synthesized noble gas-silicates. From left to right: Xe-doped olivine Mg2SiO4 [42], Xe-doped β-quartz [43], (Xe,Si)O2 phase for which Xe site occupancy is unknown [43], cristobalite-He [41]. Color code for atoms: magnesium (orange), xenon (yellow), oxygen (red), silicon (dark blue), helium (white).
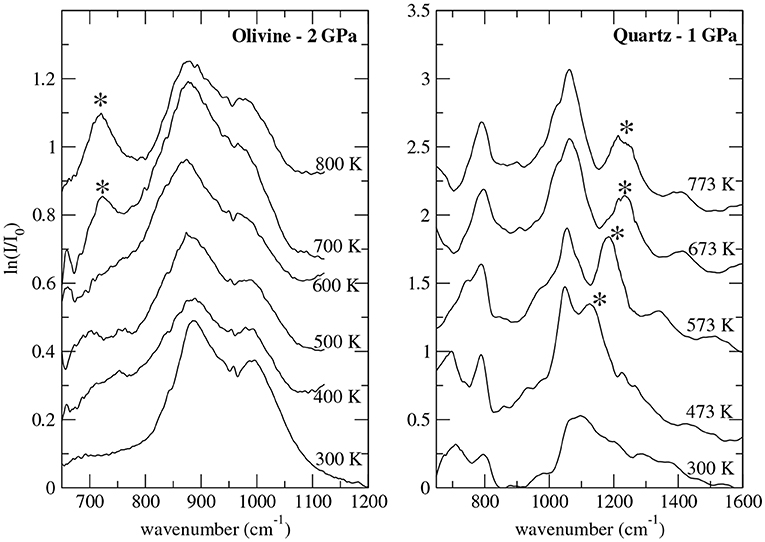
Figure 3. Infrared spectra on Xe-doped silicates under high P-T conditions generated using resistive-heating diamond-anvil cells. Left: olivine, right: quartz [43]. The asterisk sign designs new vibrational mode observed only upon heating and assigned to Xe-O bond.
Xe-doped quartz transforms into a new (Xe,Si)O2 phase upon increased heating above 1,700 K at 1 GPa [43]. (Xe,Si)O2 structure bears similarities to the predicted XeO2 structure [64] that is not stable at any of the P investigated but admittedly the best candidate for the ambient P XeO2 phase [59] as characterized by Raman spectroscopy. Both phases are orthorhombic with cell parameters within 10% difference, and have a square planar Xe local environment.
Xe reactivity with lower mantle minerals such as bridgmanite and ferropericlase has not been tested yet. At lower mantle conditions, silicon bonds to six nearest oxygen atoms in a octahedral geometry. It is therefore a promising area of research as Xe substitution to six-fold Si could lead to structures similar to ambient P Xe perovskites whereby Xe bonds to oxygens in octahedral sites [78].
4. Other High P Noble Gas Compounds Relevant of Planetary Interiors
4.1. Noble Gas Compounds Relevant to Planetary Fe Cores
The search for Xe reactivity with metals was initiated by the report of Xe metallization at 135 GPa [31], later refined to 155 GPa by means of electrical resistivity measurements [79]. Iron-xenon reactivity received most attention since the first paper by Caldwell et al. [80], showing no tendency from Xe to form a metal with Fe up to 150 GPa. Xe-metals reactivity was later shown to occur at higher P with Fe and Ni, first by alloying with Fe with up to 0.8 mol% Xe at the conditions of the terrestrial inner core [81]. Later, Xe-metal compounds were predicted from theoretical predictions [82] and observed in experiments with XeFe3 stable above 200 GPa and 2,000 K [83], conditions that are significantly reduced for XeNi3 to 150 GPa and 1,500 K [83, 84]. In these compounds, Fe and Ni act as oxidants, gaining electrons, and forming anions. This is yet another illustration of pressure-induced noble gas chemistry. Implications for Xe sequestration at depth within planetary cores are less convincing however, as Xe is missing from both martian and terrestrial atmospheres while the martian core extends to <40 GPa [85], i.e., far below the P-threshold required to induce Xe-Fe chemistry.
The latest development in this direction is the ArNi compound synthesized above 140 GPa upon laser heating (T>1,500 K) [86]. If such reaction extends to Fe, the Earth's core could be a deep Ar reservoir. ArNi is an intermetallic Laves phase, so there is no bonding but significant electron transfer between Ar and Ni atoms.
4.2. Noble Gas Compounds Relevant to Giant Planets Interiors
The range of applications in this subsection covers giant planets, which main constituents are H and He for Jupiter and Saturn, and planetary ices for Uranus and Neptune [87]. P-T conditions reach up to 4,000 GPa–20,000 K inside Jupiter [87], and 510 GPa–5,800 K inside smaller Uranus [88].
Helium is depleted from the atmosphere of Jupiter, and more importantly from that of Saturn, a depletion due to heavier He sedimenting inside the planets at P-T conditions where H2 and He are immiscible. Helium sedimentation may have sequestered Ne, which dissolves preferentially in He rather than in H2 [89]. Going beyond simple noble gas solubility in giant planets materials, other processes might sequester noble gases at depth. Van der Waals noble gas compounds with hydrogen have been synthesized: Ar(H2)2 [90] at 4.3 GPa and stable to at least 358 GPa [91], Kr(H2)4 at 5.3 GPa and stable to at least 50 GPa [92], and Xe(H2)8 at 4.8 GPa and stable to at least 255 GPa [93]. Raman and/or infra-red spectroscopies have shown that hydrogen is molecular (H2) in these compounds, freely rotating, with no indication of H-noble gas bonding.
Potential planetary He-compounds were predicted (Figure 4): NH3He above 45 GPa [95], HeH2O, and He2H2O [94] stable against decomposition at 2–8 and 8–92 GPa, respectively, and (H2O)2He above 296 GPa [96]. In none of these compounds does He form bond with other atoms. The propension of He to from compounds under high P without forming chemical bonds is explained by its insertion with ionic compounds and consequent stabilization of Coulomb interactions [94], besides the volume reduction associated with the reaction. Interestingly, like Xe2O6H6, He2H2O is superionic at high T above 40 GPa [94], with free mobility of He and protons. However, this occurs at 40 GPa only, 10 GPa lower than for pure ice. The presence of He therefore lowers the P at which superionicity occurs compared to pure water. This effect should be considered in modeling Uranus and Neptune interiors, superionic ices being the most likely candidates as the carriers of the internal planetary magnetic field.
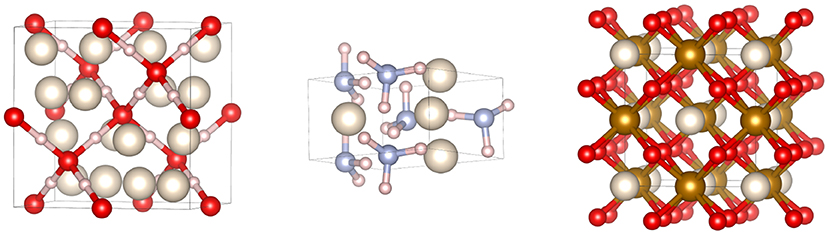
Figure 4. Noble gas compounds potentially relevant for giant planets interiors. From left to right: He2H2O [94], NH3He [95], and FeOOHe [68]. Color code for atoms: helium (white), oxygen (red), nitrogen (light blue), iron (gold), hydrogen (light pink).
5. Limitations and Future Directions in Investigating Noble Gas Reactivity in Planetary Processes
5.1. Current Limitations
Two important limitations of the study of noble gas retention mechanisms in minerals are conceptual. First, most measurements are carried out on samples synthesized at high P-T conditions but quenched to ambient conditions, i.e., far from thermodynamic equilibrium, potentially causing noble gases to exsolve. Second, solubility studies still assume neutral speciation and propose retention in defects with similar size as zero charge noble gas radii [97] such as oxygen vacancies [98, 99]. This is however unrealistic for noble gas solubilities above 1 at% as reported at lower mantle conditions for Ar in bridgmanite [98] and Kr in ferropericlase [99], oxygen defects concentrations being at least two orders of magnitude smaller [100, 101].
Even if these conceptual biases are lifted, technical limitations remain. For a chondritic Earth including 1.5% of volatile-rich late veneer [102], 90% of missing Xe [10] represents roughly 7.1013 kg of Xe. This translates into concentrations of 10 ppb if all that Xe is stored in the lower continental crust, and 0.05 ppb if stored in the upper mantle. These values are much smaller than the 0.4 at% Xe solubility in olivine and quartz [42, 43], which means that the missing Xe problem is easily solved by storage in silicates at depth. But these values are also much smaller than the detection limit of 0.1 at% for both x-ray diffraction and Raman spectroscopy [42], which is also the minimum concentration that can be handled by theoretical ab initio calculations. Neither experimental nor theoretical approaches can therefore be applied to natural levels of concentrations. Henry's law might nonetheless still be obeyed as ab initio study on Xe incorporation mechanisms in olivine [42] shows no change in the configuration of Xe with dilution ranging from 0.9 at% down to 0.1 at%; the presence of Xe is only felt locally as expected for a diluted defect and Xe atoms do not interact with each other. This argument justifies the relevance of theoretical calculations and experiments done with 0.1–1 wt% noble gas content to natural abundances.
5.2. Future Directions
Much more efforts have been devoted to understand Xe retention at depth than for lighter noble gases. However, the “missing Xe problem” requires a solution that only applies to Xe. Kr retention mechanism as a minor element has so far only been elucidated in compressed silicate melts [56], with the detection of Kr-O bonds having a lower degree of covalency than Xe-O bonds. Ar and Ne retention mechanisms will be very challenging to solve experimentally due to their lighter mass and consequent weak contribution to x-ray based signals. This could explain why these topics were so far never covered despite Ar and Ne being widely used P-transmitting media for x-ray diffraction measurements using laser-heated diamond anvil cells. Spectroscopic methods might be more straightforward to probe any noble gas induced vibration, although their use at combined high P and high T is still difficult.
Major x-ray synchrotron facilities are undergoing upgrades in this decade in order to build extremely brilliant sources with improved coherence by a factor of hundred. That should prove highly beneficial to the study of noble gas reactivity with planetary materials at more realistic conditions. This will for instance open the prospect of x-ray absorption spectroscopy measurements at below 0.1 at% concentrations. Increased photon flux will furthermore considerably shorten data collection times, allowing count-limited measurements (e.g., local structure by x-ray absorption or pdf analyses) on these diluted systems for smaller samples at lower mantle conditions using laser heated diamond-anvil cell experiments.
Last but not least, it will be crucial to evaluate the consequences of noble gas chemical reactivity at depth on isotopic fractionation during planetary differentiation processes in order to properly interpret the wealth of high precision isotopic data on natural samples.
6. Conclusion
A variety of noble gas compounds may form inside planets, and those potentially found in the Earth are summarized in Figure 5. If most of them are with Xe, even the most inert element of the periodic table, He, reacts at the extreme P-T conditions found in planetary interiors. While He-compounds do not involve He bonding to other atoms, different types of bonding are found in Xe-compounds, from covalent with Xe as an oxidizing agent (i.e., electron donner), to metallic with Xe as a reducing agent (i.e., electron taker). The main take-away message of this review is therefore that noble gas abundances measured in planetary atmospheres and rocks are certainly a very rich source of information, but they reflect not only planetary formation processes, origins and dynamics, but also P-induced noble gas chemistry at depth.
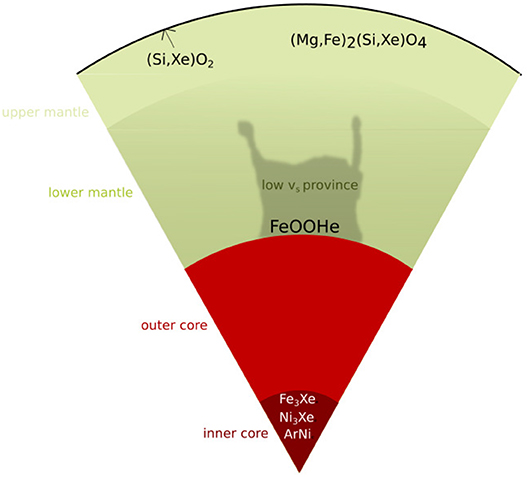
Figure 5. Cartoon of Earth's interiors and potential occurrence of noble gas-compounds at depth. Note that the Earth's crust (black crust) is too thin to be represented at scale.
As detailed in section 3, Xe trapping in the deep crust and upper mantle could solve the “missing Xe” problem in the atmospheres of the Earth and Mars, and this occurs by Xe substitution to Si whereby Xe gets oxidized such as in compressed SiO2 quartz [74]. This report stimulated further work in noble gas chemistry including the synthesis of the Graal compound XeO2 [59]. Earth sciences have thus brought a different perspective to the field, as Xe-O high energy bonds can be formed under the modest pressures of the Earth's crust in natural minerals. Reciprocally, the synthesis of Xe-water compounds by UV radiation [103] has motivated the investigation of Xe reactivity with water at extreme conditions [60], and the discovery of the Xe2O6H6 oxide stable at the conditions of the interiors of Uranus and Neptune. The search for new noble-gases compounds at high P-T conditions has also been fueled by the search for high energy compounds in condensed matter physics. The synergy between Earth and planetary sciences, high P condensed matter physics, and chemistry has been fruitful and should be pursued, in the perspective to discover new synthesis pathways and new types of noble gas compounds, with the wealth of potential societal benefits that follow such as storage of energy or better anesthetics in medicine [104]. A main effort will be to find ways to preserve the newly found compounds metastably back to room conditions, as none of these high P compounds is yet recoverable.
Future research perspectives could be directed at pursuing the synthesis of noble gas-doped phases instead of stoichiometric compounds, which proved to considerably lower the P-threshold of Xe bonding to O from 83 GPa in pure Xe-O system [61] down to 1 GPa in more complex silicate systems [43, 74]. Not only is this the mandatory way to adequately reproduce the chemistry of naturally very weakly abundant noble gases in terrestrial planets, but this is also a potential solution to recover metastable phases back to room P. In the opposite direction, the discovery of very large exoplanets (super Earths, super Neptunes, super Jupiters) opens the perspective to expand the exploratory P-range for noble gas chemistry at depth, as illustrated by the FeHe compound stable above 4,000 GPa, i.e., P greater than at the center of Jupiter [105].
Author Contributions
The author confirms being the sole contributor of this work and has approved it for publication.
Conflict of Interest
The author declares that the research was conducted in the absence of any commercial or financial relationships that could be construed as a potential conflict of interest.
Acknowledgments
SOLEIL synchrotron is acknowledged for provision of synchrotron radiation facilities at SMIS beamline, as well as Céline Crépisson and Francesco Capitani for collecting the data.
References
1. Pujol M, Marty B, Burgess R. Chondritic-like xenon trapped in Archean rocks: a possible signature of the ancient atmosphere. Earth Planet Sci Lett. (2011) 308:298–306. doi: 10.1016/j.epsl.2011.05.053
2. Pujol M, Marty B, Burgess R, Turner G, Philippot P. Argon isotopic composition of Archaean atmosphere probes early Earth geodynamics. Nature. (2013) 498:87–90. doi: 10.1038/nature12152
3. Gilmour JD, Whitby JA, Turner G. Xenon isotopes in irradiated ALH84001: evidence for shock-induced trapping of ancient Martian atmosphere. Geochim Cosmochim Acta. (1998) 62:2555–71. doi: 10.1016/S0016-7037(98)00165-3
4. Marty B, Altwegg K, Balsiger H, Bar-Nun A, Bekaert DV, Berthelier JJ, et al. Xenon isotopes in 67P/Churyumov-Gerasimenko show that comets contributed to Earth's atmosphere. Science. (2017) 356:1069–72. doi: 10.1126/science.aal3496
5. Niemann HB, Atreya SK, Carignan GR, Donahue TM, Haberman JA, Harpold DN, et al. The Galileo probe mass spectrometer: composition of jupiter's atmospheres. Science. (1996) 272:846–9. doi: 10.1126/science.272.5263.846
6. Harper CL. Evidence for 92gNb in the early solar system and evaluation of a new p-process cosmochronometer from 92gNb/92Mo. ApJ. (1996) 466:437–56. doi: 10.1086/177523
7. Williams CD, Mukhopadhyay S. Capture of nebular gases during Earth's accretion is preserved in deep-mantle neon. Nature. (2019) 565:78–81. doi: 10.1038/s41586-018-0771-1
8. Holland G, Cassidy M, Ballentine CJ. Meteorite Kr in Earth's Mantle suggests a late accretionary source for the atmosphere. Science. (2009) 326:1522–5. doi: 10.1126/science.1179518
9. Mahaffy PR, Niemann HB, Alpert A, Atreya SK, Demick J, Donahue TM, et al. Noble gas abundance and isotope ratios in the of Jupiter from the Galileo Probe Mass Spectrometer atmosphere. J Geophys Res. (2000) 105:15061–71. doi: 10.1029/1999JE001224
10. Ozima M, Podosek FA. Formation age of Earth from 129I/127I and 244Pu/238U systematics and the missing Xe. J Geophys Res. (1999) 104:25493–9. doi: 10.1029/1999JB900257
11. Avice G, Marty B. The iodine-plutonium-xenon age of the Moon-Earth system revisited. Philos Trans R Soc A. (2014) 372:20130260. doi: 10.1098/rsta.2013.0260
12. Allégre CJ, Staudacher T, Sarda P. Rare gas systematics: formation of the atmosphere, evolution and structure of the Earth's mantle. Earth Planet Sci Lett. (1986) 81:127–50. doi: 10.1016/0012-821X(87)90151-8
13. Allégre CJ, Hofmann AW, O'Nions RK. The Argon constraints on mantle structure. Geophys Res Lett. (1996) 23:3555–7. doi: 10.1029/96GL03373
14. Gonnermann HM, Mukhopadhyay S. Preserving noble gases in a convecting mantle. Nature. (2009) 459:560-U88. doi: 10.1038/nature08018
15. Anders E, Owen T. Mars and Earth: origin and abundance of volatiles. Science. (1977) 198:453–65. doi: 10.1126/science.198.4316.453
16. Krummenacher D, Merrihue CM, Pepin RO, Reynolds JH. Meteoritic krypton and barium versus the general isotopic anomalies in xenon. Geochim Cosmochim Acta. (1962) 26:231–49. doi: 10.1016/0016-7037(62)90014-5
17. Avice G, Marty B, Burgess R, Hofmann A, Philippot P, Zahnle K, et al. Evolution of atmospheric xenon and other noble gases inferred from Archean to Paleoproterozoic rocks. Geochim Cosmochim Acta. (2018) 232:82–100. doi: 10.1016/j.gca.2018.04.018
18. Pepin RO. Atmospheres on the terrestrial planets: clues to origin and evolution. Earth Planet Sci Lett. (2006) 252:1–14. doi: 10.1016/j.epsl.2006.09.014
19. Bekaert DV, Broadley MW, Delarue F, Avice G, Robert F, Marty B. Archean kerogen as a new tracer of atmospheric evolution: Implications for dating the widespread nature of early life. Sci Adv. (2018) 4:eaar2091. doi: 10.1126/sciadv.aar2091
20. Zahnle KJ, Gaseca M, Catling DC. Strange messenger: a new history of hydrogen on Earth, as told by xenon. Geochim Cosmochim Acta. (2018) 244:56–85. doi: 10.1016/j.gca.2018.09.017
21. Ozima M, Podosek F. A. (2002). Noble Gas Geochemistry, 2nd ed., Vol. 140, Cambridge, New York, NY, Melbourne, VIC: Cambridge University Press.
22. Matsuda JI, Matsubara K. Noble gases in silica and their implication for the terrestrial ‘missing' Xe. Geophys Res Lett. (1989) 16:81–4. doi: 10.1029/GL016i001p00081
23. Kunz J, Staudacher T, Allegre CJ. Plutonium-fission xenon found in earth's mantle. Science. (1998) 280:877–80. doi: 10.1126/science.280.5365.877
24. Moreira M, Kunz J, Allégre C. Rare gas systematics in popping rock: isotopic and elemental compositions in the upper mantle. Science. (1998) 279:1178–81. doi: 10.1126/science.279.5354.1178
25. Hennecke EW, Manuel OK. Noble gases in Hawaiian xenolith. Nature. (1975) 257:778–80. doi: 10.1038/257778b0
26. Kaneoka I, Takaoka N, Aoki KI. Rare gases in a phlogopite nodule and a phlogopite-bearing peridotite in South African kimberlites. Earth Planet. Sci. Lett. (1977) 136:181–6.
27. Poreda RJ, Farley KA. Rare gases in Samoan xenoliths. Earth Planet Sci Lett. (1992) 113:129–44. doi: 10.1016/0012-821X(92)90215-H
28. Czuppon G, Matsumoto T, Handler MR, Matsuda JI. Noble gases in spinel peridotite xenoliths from Mt Quincan, North Queensland, Australia: undisturbed MORB-type noble gases in the subcontinental lithospheric mantle. Chem Geol. (2009) 266:19–28. doi: 10.1016/j.chemgeo.2009.03.029
29. Kuroda PK, Sherrill RD, Jackson KC. Abundances and isotopic compositions of rare gases in granites. Geochem J. (1977) 11:75–90. doi: 10.2343/geochemj.11.75
30. Bekaert DV, Avice G, Marty B, Henderson B. Stepwise heating of lunar anorthosites 60025, 60215, 65315 possibly reveals an indigenous noble gas component on the Moon. Geochim Cosmochim Acta. (2017) 218:114–1315. doi: 10.1016/j.gca.2017.08.041
31. Goettel KA, Eggert JH, Silvera IF. Optical evidence for the metallization of xenon at 132(5) GPa. Phys Rev Lett. (1989) 62:665–8. doi: 10.1103/PhysRevLett.62.665
32. Grochala W. Atypical compounds of gases, which have been called noble. Chem Soc Rev. (2007) 36:1632–55. doi: 10.1039/b702109g
34. Dyadin YA, Aladko EY, Manakov AY, Zhurko FV, Mikina TV, Komarov VY, et al. Clathrate formation in water-noble gas (hydrogen) systems at high pressures. J Struct Chem. (1999) 40:790–5. doi: 10.1007/BF02903454
35. Sanloup C, Hemley RJ, Mao HK. Evidence for xenon silicates at high pressure and temperature. Geophys Res Lett. (2002) 29:1883. doi: 10.1029/2002GL014973
36. Holland G, Ballentine CJ. Seawater subduction controls the heavy noble gas composition of the mantle. Nature. (2006) 441:186–91. doi: 10.1038/nature04761
37. Jackson CRM, Parman SW, Kelley SP, Cooper RF. Noble gas transport into the mantle facilitated by high solubility in amphibole. Nat Geosci. (2013) 6:562–5. doi: 10.1038/ngeo1851
38. Saito K Jr, Dragon JC. Rare gases in cyclosilicates and cogenetic minerals. Chem Geol. (1984) 89:7891–901. doi: 10.1029/JB089iB09p07891
39. Krantz JA, Parman SW, Kelley SP. Recycling of heavy noble gases by subduction of serpentinite. Earth Planet Sci Lett. (2019) 521:120–7. doi: 10.1016/j.epsl.2019.06.007
40. Sanloup C, Mao HK, Hemley RJ. High-pressure transformations in xenon hydrates. Proc Natl Acad Sci USA. (2002) 99:25–8. doi: 10.1073/pnas.221602698
41. Matsui M, Tomoko S, Funamori N. Crystal structures and stabilities of cristobalite-helium phases at high pressures. Am Miner. (2014) 99:184–9. doi: 10.2138/am.2014.4637
42. Crépisson C, Blanchard M, Lazzeri M, Balan E, Sanloup C. New constraints on Xe incorporation mechanisms in olivine from first-principles calculations. Geochim Cosmochim Acta. (2018) 222:146–55. doi: 10.1016/j.gca.2017.10.028
43. Crépisson C, Sanloup C, Blanchard M, Hudspeth J, Glazyrin K, Capitani F. The Xe-SiO2 system at moderate pressure and high temperature. Geochem Geophys Geosyst. (2019) 20:992–1003. doi: 10.1029/2018GC007779
44. Shackelford JF, Mazaryk A. The interstitial structure of vitreous silica. J NonCryst Solids. (1978) 30:127–39. doi: 10.1016/0022-3093(78)90061-3
45. Carroll MR, Stolper EM. Noble gas solubilities in silicate melts and glasses: new experimental results for argon and the relationship between solubility and ionic porosity. Geochim Cosmochim Acta. (1993) 57:5039–51. doi: 10.1016/0016-7037(93)90606-W
46. Schmidt B, Keppler H. Experimental evidence for high noble gas solubilities in silicate melts under mantle pressures. Earth Planet Sci Lett. (2002) 195:277–90. doi: 10.1016/S0012-821X(01)00584-2
47. Sarda P, Guillot B. Breaking of Henry's law for noble gas and CO2 solubility in silicate melt under pressure. Nature. (2005) 436:95–8. doi: 10.1038/nature03636
48. Chamorro-Perez EM, Gillet P, Jambon A, MacMillan PF, Badro J. Low argon solubility in silicate melts at high pressure. Nature. (1998) 393:352–5. doi: 10.1038/30706
49. Bouhifd MA, Jephcoat A. Aluminium control of argon solubility in silicate melts under pressure. Nature. (2006) 439:961–4. doi: 10.1038/nature04583
50. Niwa K, Miyakawa C, Yagi T, Matsuda J. Argon solubility in SiO2 melt under high pressures: a new experimental result using laser-heated diamond anvil cell. Earth Planet Sci Lett. (2013) 363:1–8. doi: 10.1016/j.epsl.2012.12.014
51. Sato T, Funamori N, Yagi T. Helium penetrates into silica glass and reduces its compressibility. Nat Commun. (2011) 2:345. doi: 10.1038/ncomms1343
52. Shen G, Mei Q, Prakapenka VB, Lazor P, Sinogeikin S, Meng Y, et al. Effect of helium on structure and compression behavior of SiO2 glass. Proc Natl Acad Sci USA. (2011) 108:6004. doi: 10.1073/pnas.1102361108
53. Leroy C, Sanloup C, Bureau H, Schmidt BC, Konôpková Z, Raepsaet C. Bonding of xenon to oxygen in magmas at depth. Earth Planet Sci Lett. (2018) 484:103–10. doi: 10.1016/j.epsl.2017.12.019
54. Kohara S, Akola J, Morita H, Suzuya K, Weber JKR, Wilding MC, et al. Relationship between topological order and glass forming ability in densely packed enstatite and forsterite composition glasses. Proc Natl Acad Sci USA. (2011) 108:14780–5. doi: 10.1073/pnas.1104692108
55. Shi Y, Neuefeind J, Ma D, Page K, Lamberson LA, Smith NJ, et al. Ring size distribution in silicate glasses revealed by neutron scattering first sharp diffraction peak analysis. J Non-Cryst Sol. (2019) 516:71–81. doi: 10.1016/j.jnoncrysol.2019.03.037
56. Crépisson C, Sanloup C, Cormier L, Blanchard M, Hudspeth J, Rosa AD, et al. Kr environment in feldspathic glass and melt: a high pressure, high temperature X-ray absorption study. Chem Geol. (2018) 493:525–31. doi: 10.1016/j.chemgeo.2018.07.008
57. Templeton DH, Zalkin A, Forrester JD, Williamson SM. Crystal and molecular structure of xenon trioxide. J Am Chem Soc. (1963) 85:817. doi: 10.1021/ja00889a037
58. Huston JL, Studier MH, Cloth EN. Xenon tetroxide: mass spectrum. Science. (1964) 143:1161–2. doi: 10.1126/science.143.3611.1161-a
59. Brock DS, Schrobilgen GJ. Synthesis of the missing oxide of xenon, XeO2, and its implications for Earth's missing xenon. J Am Chem Soc. (2011) 133:6265–9. doi: 10.1021/ja110618g
60. Sanloup C, Bonev SA, Hochlaf M, Maynard-Casely HE. Reactivity of xenon with ice at planetary conditions. Phys Rev Lett. (2013) 110:265501. doi: 10.1103/PhysRevLett.110.265501
61. Dewaele A, Worth N, Pickard CJ, Needs RJ, Pascarelli S, Mathon O, et al. Synthesis and stability of xenon oxides Xe2O5 and Xe3O2 under pressure. Nat Chem. (2016) 8:784–90. doi: 10.1038/nchem.2528
62. Cavazzoni C, Chiarotti GL, Scandolo S, Tosatti E, Bernasconi M, Parrinello M. Superionic states of water and ammonia at giant planet conditions. Science. (1999) 283:44–6. doi: 10.1126/science.283.5398.44
63. Zhu Q, Jung DY, Oganov AR, Glass CW, Gatti C, Lyakhov AO. Stability of xenon oxides at high pressures. Nat Chem. (2013) 5:61–5. doi: 10.1038/nchem.1497
64. Hermann A, Schwerdtfeger P. Xenon suboxides stable under pressure. J Phys Chem Lett. (2014) 5:4336–42. doi: 10.1021/jz502230b
65. Lundegaard LF, Guillaume C, McMahon MI, Gregoryanz E, Merlini M. On the structure of high-pressure high-temperature η-O2. J Chem Phys. (2009) 130:164516. doi: 10.1063/1.3118970
66. Zaleski-Ejgierd P, Lata PM. Krypton oxides under pressure. Sci Rep. (2016) 6:18938. doi: 10.1038/srep18938
67. Abelson PH. Experimental technology. Science. (1999) 283:1263. doi: 10.1126/science.283.5406.1263
68. Zhang J, Lv J, Li H, Feng X, Lu C, Redfern SAT, et al. Rare helium-bearing compound FeO2He stabilized at deep-earth conditions. Phys Rev Lett. (2018) 121:255703. doi: 10.1103/PhysRevLett.121.255703
69. Jackson MG, Konter JG, Becker TW. Primordial helium entrained by the hottest mantle plumes. Nature. (2017) 542:340–3. doi: 10.1038/nature21023
70. Garnero EJ, McNamara AK. Structure and dynamics of earth's lower mantle. Science. (2008) 320:626–8. doi: 10.1126/science.1148028
71. Hu J, Mao H, Guo Q, Hemley RJ. FeO2 and FeOOH under deep lower-mantle conditions and Earth's oxygen-hydrogen cycles. Nature. (2016) 534:241–4. doi: 10.1038/nature18018
72. Liu J, Hu Q, Kim DY, Wu Z, Wang W, Xiao Y, et al. Hydrogen-bearing iron peroxide and the origin of ultralow-velocity zones. Nature. (2017) 551:494–7. doi: 10.1038/nature24461
73. Hua Q, Kim DY, Liu J, Meng Y, Yang L, Zhange WLM, et al. Dehydrogenation of goethite in Earth's deep lower mantle. Proc Natl Acad Sci USA. (2017) 114:1498–501. doi: 10.1073/pnas.1620644114
74. Sanloup C, Schmidt BC, Perez EC, Jambon A, Gregoryanz E, Mezouar M. Retention of xenon in quartz and Earth's missing xenon. Science. (2005) 310:1174–7. doi: 10.1126/science.1119070
75. Sanloup C, Schmidt BC, Gudfinnsson G, Dewaele A, Mezouar M. Xenon and argon: a contrasting behavior in olivine at depth. Geochim Cosmochim Acta. (2011) 75:6271–84. doi: 10.1016/j.gca.2011.08.023
76. Probert MIJ. An ab initio study of xenon retention in α-quartz. J Phys. (2010) 22:025501. doi: 10.1088/0953-8984/22/2/025501
77. Kalinowski J, Rasanen M, Gerber RB. Chemically-bound xenon in fibrous silica. Phys Chem Chem Phys. (2014) 16:11658–61. doi: 10.1039/C4CP01355G
78. Britvin SN, Kashtanov SA, Krivovichev SV, Chukanov NV. Xenon in rigid oxide frameworks: structure, bonding and explosive properties of layered perovskite K4Xe3O12. J Am Chem Soc. (2016) 138:13838–41. doi: 10.1021/jacs.6b09056
79. Eremets MI, Gregoryanz EA, Struzhkin VV, Mao H, Hemley RJ. Electrical conductivity of xenon at megabar pressures. Phys Rev Lett. (2000) 85:2797–800. doi: 10.1103/PhysRevLett.85.2797
80. Caldwell WA, Nguyen JH, Pfrommer BG, Mauri F, Louie SG, Jeanloz R. Structure, bonding and geochemistry of xenon at high pressures. Science. (1997) 277:930–3. doi: 10.1126/science.277.5328.930
81. Lee KM, Steinle-Neumann G. High-pressure alloying of iron and xenon: “Missing” Xe in the Earth's core? J Geophys Res. (2006) 111:B02202. doi: 10.1029/2005JB003781
82. Zhu L, Liu H, Pickard CJ, Zou G, Ma Y. Reactions of xenon with iron and nickel are predicted in the Earth's inner core. Nat Chem. (2014) 6:645–9. doi: 10.1038/nchem.1925
83. Stavrou E, Yao Y, Goncharov AF, Lobanov SS, Zaug JM, Liu H, et al. Synthesis of xenon and iron-nickel intermetallic compounds at earth's core thermodynamic conditions. Phys Rev Lett. (2018) 120:096001. doi: 10.1103/PhysRevLett.120.096001
84. Dewaele A, Ppin CM, Geneste G, Garbarino G. Reaction between nickel or iron and xenon under high pressure. High Press Res. (2016) 97:215504. doi: 10.1080/08957959.2016.1267165
85. Rivoldini A, Hoolst TV, Verhoeven O, Mocquet A, Dehant V. Geodesy constraints on the interior structure and composition of Mars. Icarus. (2011) 213:451–72. doi: 10.1016/j.icarus.2011.03.024
86. Adeleke AA, Kunz M, Greenberg E, Prakapenka VB, Yao Y, Stavrou E. A high-pressure compound of argon and nickel: noble gas in the earth's core? ACS Earth Space Chem. (2019) 3:2517–24. doi: 10.1021/acsearthspacechem.9b00212
87. Guillot T. Interior of giant planets inside and outside the Solar System. Science. (1999) 286:72–7. doi: 10.1126/science.286.5437.72
88. Redmer R, Mattsson TR, Nettelmann N, French M. The phase diagram of water and the magnetic fields of Uranus and Neptune. Icarus. (2011) 211:798–803. doi: 10.1016/j.icarus.2010.08.008
89. Wilson HF, Militzer B. Sequestration of noble gases in giant planet interiors. Phys Rev Lett. (2010) 104:121101. doi: 10.1103/PhysRevLett.104.121101
90. Loubeyre P, LeToullec R, Pinceaux JF. Compresson of Ar(H2) up to 175 GPa - A new path for the dissociation of molecular hydrogen. Phys Rev Lett. (1994) 72:1360–3. doi: 10.1103/PhysRevLett.72.1360
91. Ji C, Goncharov AF, Shukla V, Jena NK, Popov D, Li B, et al. Stability of Ar(H2)2 to 358 GPa. Proc Natl Acad Sci USA. (2017) 114:3596–600. doi: 10.1073/pnas.1700049114
92. Kleppe AK, Amboage M, Jephcoat AP. New high-pressure van der Waals compound Kr(H2)4 discovered in the krypton-hydrogen binary system. Sci Rep. (2014) 4989:4. doi: 10.1038/srep04989
93. Somayazulu M, Dera P, Goncharov AF, Gramsch SA, Liermann P, Yang W, et al. Pressure-induced bonding and compound formation in xenon-hydrogen solids. Nat Chem. (2009) 2:50–3. doi: 10.1038/nchem.445
94. Liu C, Gao H, Wang Y, Needs RJ, Pickard CJ, Sun J, et al. Multiple superionic states in helium-water compounds. Nat Phys. (2019) 15:1065–70. doi: 10.1038/s41567-019-0568-7
95. Bai Y, Liu Z, Botana J, Yan D, Lin HQ, Sun J, et al. Electrostatic force driven helium insertion into ammonia and water crystals under pressure. Commun Chem. (2019) 2:102. doi: 10.1038/s42004-019-0204-6
96. Liu HY, Yao YS, Klug DD. Stable structures of He and H2O at high pressure. Phys Rev B. (2015) 9:014102. doi: 10.1103/PhysRevB.91.014102
97. Brooker RA, Du Z, Blundy JD, Kelley SP, Allan NL, Wood BJ, et al. The 'zero charge' partitioning behaviour of noble gases during mantle melting. Nature. (2003) 423:738–41. doi: 10.1038/nature01708
98. Shcheka SS, Keppler H. The origin of the terrestrial noble-gas signature. Nature. (2012) 490:531–4. doi: 10.1038/nature11506
99. Rosa AD, Bouhifd MA, Morard G, Briggs R, Garbarino G, Irifune T, et al. Krypton storage capacity of the Earth's lower mantle. Earth Planet Sci Lett. (2020) 532:116032. doi: 10.1016/j.epsl.2019.116032
100. Hirsch LM, Shankland TJ. Equilibrium point defect concentrations in MgO: understanding the mechanisms of conduction and diffusion and the role of Fe impurities. J Geophys Res. (1991) 96:385–403. doi: 10.1029/90JB02175
101. Hirsch LM, Shankland TJ. Point defects in (Mg,Fe)SiO3 perovskite. Geophys Res Lett. (1991) 96:1305–8. doi: 10.1029/91GL01582
102. Dauphas N, Marty B. Inference on the nature and the mass of Earth's late veneer from noble metals and gases. J Geophys Res Planets. (2002) 107:JE001617. doi: 10.1029/2001JE001617
103. Khriachtchev L, Isokoski K, Cohen A, Räsänen M, Gerber RB. A small neutral molecule with two noble-gas atoms: HXeOXeH. J Am Chem Soc. (2008) 130:6114–8. doi: 10.1021/ja077835v
104. Franks NP, Dickinson R, de Sousa SLM, Hall AC, Lieb WR. How does xenon produce anaesthesia? Nature. (1998) 396:324. doi: 10.1038/24525
Keywords: xenon, krypton, argon, neon, helium, planetary interiors, high pressure
Citation: Sanloup C (2020) Noble Gas Reactivity in Planetary Interiors. Front. Phys. 8:157. doi: 10.3389/fphy.2020.00157
Received: 10 March 2020; Accepted: 15 April 2020;
Published: 08 May 2020.
Edited by:
Gabriel Merino, Centro de Investigacion y de Estudios Avanzados - Unidad Mérida, MexicoReviewed by:
Michael Ward Broadley, UMR7358 Centre de Recherches Pétrographiques et Géochimiques (CRPG), FranceGuochun Yang, Northeast Normal University, China
Copyright © 2020 Sanloup. This is an open-access article distributed under the terms of the Creative Commons Attribution License (CC BY). The use, distribution or reproduction in other forums is permitted, provided the original author(s) and the copyright owner(s) are credited and that the original publication in this journal is cited, in accordance with accepted academic practice. No use, distribution or reproduction is permitted which does not comply with these terms.
*Correspondence: Chrystele Sanloup, chrystele.sanloup@sorbonne-universite.fr