- Electrical and Computer Engineering Department, Old Dominion University, Norfolk, VA, United States
Low temperature plasmas that can be generated at atmospheric pressure and at temperatures below 40°C have in the past couple of decades opened up a new frontier in plasma applications: biomedical applications. These plasma sources produce agents, such as reactive species (radicals and non-radicals), charged particles, photons, and electric fields, which have impactful biological effects. Investigators have been busy elucidating the physical and biochemical mechanisms whereby low temperature plasma affects biological cells on macroscopic and microscopic scales. A thorough understanding of these mechanisms is bound to lead to the development of novel plasma-based medical therapies. This mini review introduces the reader to this exciting multidisciplinary field of research.
Introduction
Plasma medicine is about using low temperature atmospheric pressure plasmas to generate controllable amounts of specific chemically reactive species that are transported to react with biological targets including cells and tissues. The remarkable achievement of this plasma application is that it took only about 25 years to take it from initial discovery, to fundamental scientific investigation stage, and finally to applications on actual patients. How did this happen in a relatively short time? A brief answer to this question is that although the field started in a rather modest and unexpected way, it did not take long for the plasma physics community to realize its great potential and its revolutionary promise. This was accentuated by the recruitment of health science experts (biochemists, microbiologists, etc.) who joined the various research endeavors and greatly advanced the ongoing research. Up until the present the mechanisms of action of plasma on cells and tissues are still not fully understood but the body of knowledge has been steadily growing and our understanding has expanded significantly to include a relatively good grasp on the physical and biochemical pathways whereby plasma impacts biological matter.
The field started in the mid-1990s by few proof of principle experiments which showed that low temperature plasma (LTP) possesses efficient bactericidal property [1–5]. It was realized from the very beginning that the reactive species generated by LTP, which include reactive oxygen species (ROS) and reactive nitrogen species (RNS) played a pivotal role in the observed biological outcomes [1, 6]. It also became quickly apparent that LTP can not only be used to inactivate pathogens, such as bacteria, on abiotic surfaces but it can also be used to disinfect biological tissues and therefore can be employed for wound healing. In due time these early bold ideas, backed by some preliminary experimental data, resonated strongly within the LTP research community, which by then (around 2005) realized what these new, promising but not fully explored applications meant and joined this emerging research field in droves. Consequently, advances and new milestones were reached at relatively “break neck” speed, and by the beginning of the second decade of the 2000s clinical trials on chronic wounds were conducted with some success [7]. In addition small doses of LTP were found to selectively kill cancer cells without harming healthy ones. This opened up another research avenue sometimes referred to as “plasma oncology.” Investigators from research labs around the world reported promising in vitro and in vivo results on the killing of various cancer cell lines (see review [8] and references therein). The cell lines included those associated with leukemia, carcinoma, breast cancer, brain cancer, prostate cancer, colorectal cancer, etc. [8]. In addition, more recently, cold plasma was used in Germany in limited preliminary trials as a palliative therapy for head and neck cancer patients [9]. The above described various efforts finally culminated in the US Food & Drug Administration (FDA) approval of the first clinical trials in the USA in 2019. This constitutes yet another major milestone for the efforts to develop novel LTP-based cancer therapies.
In this mini review, descriptions of LTP sources used in plasma medicine is first given, then some major medical applications are briefly described.
Cold Atmospheric Pressure Plasma Sources
Two types of plasma discharges have been used extensively in biomedical applications: The dielectric barrier discharge (DBD) and the non-equilibrium atmospheric pressure plasma jet (N-APPJ). Figure 1 illustrates two photographs showing a DBD ignited in argon gas (left photo) and the plasma plume emanating from a N-APPJ operated with helium (right photo).
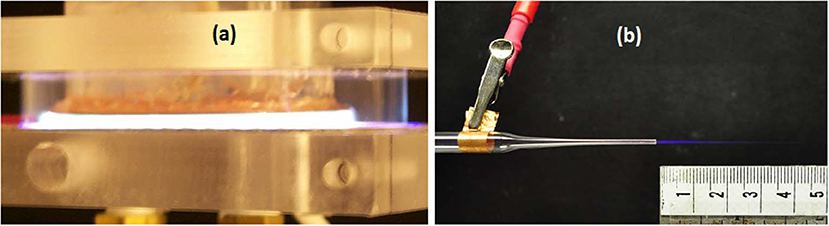
Figure 1. Two sources of low temperature atmospheric pressure plasma: Dielectric Barrier Discharge in argon driven by repetitive short duration (ns - μs) high voltage pulses (a); A micro-jet using helium as operating gas, generating a cold plasma plume about 2.5 cm in length (b).
Dielectric Barrier Discharge (DBD)
Dielectric Barrier Discharges are ideal for the generation of large volume non-equilibrium atmospheric pressure diffuse plasma. Extensive investigations allowed for a good understanding and improvement of their operation [10–23]. DBDs use a dielectric material, such as glass or alumina, to cover at least one of the electrodes. The electrodes are driven by high AC voltages in the kV range and at frequencies in the kHz. Plasmas generated by DBDs have been used for ozone generation, for material surface modification, as flow control actuators, etc. DBDs most recent domain of application has been in biomedicine after their successful early use in the mid-1990s to inactivate bacteria [1]. Today they are used in various biomedical applications including wound healing and the destruction of cancer cells and tumors [24–27].
Sinusoidal voltages with amplitudes in the kV range and frequencies of few the kHz were originally used to power DBDs. However, since the early 2000s it was found that repetitive high voltage short pulses (ns - μs) offered a more efficient way to enhance the chemistry of such discharges [28–30]. DBDs are able to maintain the non-equilibrium state of the plasma due surface charge accumulation on the dielectric surface as soon as a discharge is ignited. This creates an electrical potential that counteracts the externally applied voltage and results in a self-limited pulsed current waveform.
The electron energy distribution function (EEDF) defines/controls the chemistry in the plasma. Short repetitive high voltage pulses allow for preferential heating of the electrons population and therefore an increase of ionization and excitation [29]. Pulses with widths less than the characteristic time of the onset of the glow-to-arc transition maintain stable non-equilibrium low temperature plasma [29, 30].
To extend the operating frequency range below the kHz few methods were proposed. For example, Okazaki and co-workers used a dielectric wire mesh electrode to generate a discharge at a frequency of 50 Hz [16]. Laroussi and co-workers used a high resistivity layer/film to cover one of the electrodes in a device they referred to as the Resistive Barrier Discharge (RBD) [31]. The RBD can be operated with low frequencies extending all the way to DC. The film barrier usually has a resistivity of few MΩ.cm. The high resistivity film plays the role of a distributed resistive ballast which inhibits the discharge from localizing and the current from reaching high values.
Non-equilibrium Atmospheric Pressure Plasma Jets (N-APPJ)
Although plasma jets were previously employed for material processing applications [32, 33] biotolerant plasma jets developed specifically for plasma medicine have been in use only since the mid-2000s [34, 35]. These jets can emit low temperature plasma plumes in the surrounding air. Because they can maintain temperatures below 40°C, they can come in touch with soft matter, including biological tissues, without causing thermal damage. These plasma sources proved to be very useful for various applications including biomedical applications [26, 34, 35]. Because the plasma propagates away from the high voltage electrodes and into a region free from high voltage the plasma does not cause electrical shock/damage to the target cells or tissues. However, the plasma plume does exhibit a very high instantaneous and local electric field at its tip. This field plays a role in the propagation of the plasma plume and can also affect the treated target.
Investigators discovered that the plasma plumes generated by N-APPJs are not continuous volumes of plasma but discrete plasma packets/bullets propagating at high velocities, up to 105 m/s [36, 37]. The mechanisms governing the generation and propagation of these plasma bullets were reported by both experimental and modeling investigations [38–49]. A photoionization model was proposed by Lu and Laroussi who first investigated the dynamics of the plasma bullet [37]. Further investigations also showed that the high electrical field at the head of the plume plays a role in the propagation process. The average strength of this electric field was experimentally measured to be in the 10–30 kV/cm range [50–52].
The low temperature plasma sources described above produce chemically reactive species including reactive oxygen species (ROS) and reactive nitrogen species (RNS), which are known from redox biology to play important biological roles [53]. Other agents generated by these plasma sources are also suspected to play active roles in biological applications. These include charges particles (electrons and ions), UV and VUV radiation, and electric fields. For example the electric field can cause electroporation of cell membranes, allowing molecules (including ROS and RNS) to enter the cells and cause damage to the cell's internal organelles (including mitochondria) and macromolecules such as lipids, proteins, and DNA. To learn more about the physics and design of LTP sources the reader is referred to the following references [23, 27, 54–57].
Applications of Cold Plasma in Biology and Medicine
The early groundbreaking experiments using low temperature atmospheric pressure plasma for biomedical applications were conducted in a decade spanning from 1995 to 2004 [1–6, 58–60]. The earliest experiments involved the use of dielectric barrier discharge to inactivate bacteria on surfaces and in liquids [1, 58] and to generate pulsed plasma in saline solutions for surgical applications [61, 62]. Works on using cold plasma for the disinfection of wounds, enhancement of proliferation of fibroblasts, and cell detachment soon followed [25, 59, 60]. Eventually these seminal works attracted the interest of the low temperature plasma research community and the field witnessed a substantial growth in the years following 2005 and until the present. Applications in wound healing, dentistry, cancer treatment, etc. have since then been pursued in various laboratories and research centers around the world leading to a remarkable increase in the number of journal manuscripts on the topic and to the publication of several books [63–66].
The ability of cold atmospheric plasma to inactivate bacteria recently gained more relevance because modern society has been facing several serious healthcare challenges. Amongst these are: (1) Antibiotic resistant strains of bacteria such as Methicillin Resistant Staphylococcus aureus (MRSA) and Clostridium difficile (C-diff) are sources of hospital acquired infection (HAI), which can be fatal to patients with a compromised immune system; (2) Chronic wounds, such as diabetic ulcers, do not heal easily or at all, and one of the problems is the high level of infection caused by a spectrum of bacteria. The inability of conventional methods to satisfactorily deal with these problems necessitated the need for novel approaches based on new technologies. Cold atmospheric plasma has been shown to effectively inactivate bacteria such as MRSA and to greatly reduce the bioburden in infected chronic wounds, making it a very attractive technology that can be used to help overcome the challenges listed above. In 2010, the first clinical trials on the treatment of chronic wounds with cold atmospheric plasma took place and yielded encouraging results [7]. Today there are several plasma devices on the market which have been licensed as medical instruments and which can be used in medicine, including the treatment of various dermatological diseases.
LTP can be applied in two different ways. The first is what is referred to as “direct” exposure. In this mode of application the plasma comes in direct contact with the biological target and therefore all plasma-produced agents act on the cells/tissues. The second mode is what is referred to as “indirect” exposure. In this case only the afterglow of the plasma is used or the plasma is first used to activate a liquid medium then the plasma-activated liquid is applied on top of cells/tissues. One of the advantages of the latter is that the plasma activated liquid (PAL) can be stored and used at a later time, giving a degree of flexibility that direct exposure does not offer.
Direct Exposure
As mentioned earlier under direct exposure the biological target is subjected to all plasma agents including charged particles, photons, electric field, and reactive species. These agents act alone and/or in synergy to produce certain biological outcomes. In the case of bacteria inactivation, all the above agents were reported to play a role. Lysing of vegetative cells as well spores were reported after direct exposure to LTP, but cell death without lysis was reported as well for gram-positive bacteria [67, 68].
The inactivation of bacteria by LTP has several applications ranging from sterilization of heat sensitive medical tools, to the destruction of biofilms, to disinfection of wounds, to decontamination of liquids, food, and agricultural products.
Direct exposure has also been used in a non-lethal way to affect eukaryotic cell functions, by modulating cell signaling pathways [69], and in a lethal way for the destruction of cancer cells and tumors [70–74]. Experiments using various cell lines have been reported which showed that under a certain exposure dose LTP can kill cancer cells in a selective manner [70–74]. Investigators reported that LTP exposure leads to an increase in intracellular ROS concentrations. Since cancer cells are under high oxidative stress, the increase in ROS leads to severe redox imbalance, which can lead to one or more of the following: DNA damage, mitochondrial dysfunction, caspase activation, advanced state of oxidation of proteins, etc. Such acute stress ultimately leads to cancer cells death.
Indirect Exposure
In this section we limit the discussion to the case of plasma activated liquids (PAL). In this mode of exposure only long lived chemical species that diffuse and solvate into the aqueous state play a role. This eliminates the effects of photons, electric field, short lived species, and heat. Liquids that have been used include water to make plasma activated water (PAW) and biological culture media to make plasma activated media (PAM). The following discussion focuses on the use of PAM to destroy cancer cells. Over the past few years investigators have reported encouraging results on the use of PAM in vitro and in vivo to kill cancer cells and reduce tumors [75–81]. The anticancer characteristic of PAM has been attributed to the long lived species produced in the liquid phase after LTP exposure. These species include hydrogen peroxide, H2O2, nitrite, , nitrate, , peroxynitrite, ONOO−, and organic radicals.
The making of PAM involves the exposure of a liquid medium to an LTP source, most frequently the plasma plume of a plasma jet, for a certain length of time. Media used include Eagle's Minimum Essential Medium (EMEM), Dulbecco's Modified Eagle Medium (DMEM), Ringer's Lactate solution (RL), Roswell Park Memorial Institute medium (RPMI), with additives such as serum (e.g., bovine serum), glutamine, and antibiotics (e.g., mix of Penicillin/Streptomycin). As an example of producing PAM, a 24-well plate can be used where a few ml of fresh cell culture media is added to each well. Each well can be treated by LTP for a certain length of time, this way producing different PAMs with different “strengths.” To illustrate the effects of PAM on cancer cells the following work done at the author's laboratory is summarized [81].
In this experiment, to make PAM, 1 ml of fresh cell culture media (MEM) was added to each well of a 24 well plate. Each well was exposed to the plume of the plasma pencil (a pulsed plasma jet) for a designated time. After exposure, the media on top of cells grown in a 96-well plate was replaced by 100 μl of PAM. After PAM application, cells were stored at 37°C in a humidified incubator with 5% CO2. Media not exposed by LTP was used for the control sample. The cancer cell line used was SCaBER (ATCC® HTB3™) cell line from a urinary bladder tissue with squamous cell carcinoma. Cell viability was quantified at different times of incubations using The CellTiter 96® AQueous One Solution Cell Proliferation Assay (MTS) (Promega, Madison, MI, USA). To quantify the MTS assay results trypan blue exclusion assay was used [81]. Figure 2 shows the results.
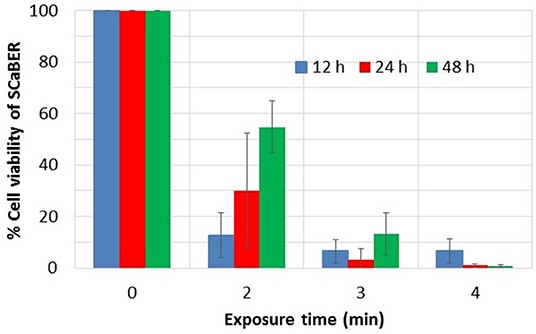
Figure 2. Viability of SCaBER cells after PAM treatment, using MTS assay. Exposure time indicates the time the liquid media was exposed to plasma to make PAM. Measurements were made after 12, 24, and 48 h of PAM application. Data is based on three independent experiments using two replications each. This figure is plotted based on data previously published in Mohades et al. [81].
As can be seen in Figure 2, PAM that was created by longer LTP treatment times causes a greater cell kill. PAM created with an exposure time greater than 3 min induces more than 90% cell reduction. However, for the 2 min case, over time the proliferation of live cells overtakes the destruction of cells and therefore an increase in viability at 24 and 48 h was observed. To investigate the role of the reactive species in the killing of SCaBER cells measurements of hydrogen peroxide, H2O2, produced in PAM were made. It was found that the concentration of H2O2 in PAM increased with exposure time and correlated well with the reduction in cell viability of SCaBER [81]. This was in agreement with works of various investigators which have shown the key role H2O2 plays in the anticancer efficacy of PAM. Recently Bauer proposed the hypothesis that H2O2 and nitrite lead to the generation of singlet oxygen (1O2) which causes inactivation of catalase [82]. Catalase, which is normally expressed on the membrane of cancer cells, protects them from intercellular ROS/ RNS signaling. With enough inactivation of catalase an influx of H2O2 via aquaporin occurs. Therefore the inactivation of the protective catalase causes ROS mediated signals that lead to apoptosis of malignant cells. Since healthy cells do not express catalase on their surface they are subject to an influx of ROS such as H2O2 or peroxynitrite. So if they are exposed to very high ROS concentrations they also can be damaged. Therefore, the applied dose of ROS/RNS has to be below a certain threshold to achieve selective killing of cancer cells.
Conclusion
The application of low temperature atmospheric pressure plasma in biomedicine opened up new frontiers in science and technology. On a scientific level, new fundamental knowledge (albeit still incomplete) regarding the interaction of plasma with soft matter has been created. Before the mid-1990s basic scientific understanding of the physical and biochemical effects of plasma on cells and tissues was simply missing. Today, 25 years later and after extensive and hectic scientific investigations, our knowledge has greatly grown and many of the mechanisms involved have been elucidated on the cellular and sub-cellular levels. This allowed remarkable advances in the quest of developing novel plasma-based therapies to overcome various healthcare challenges. The recent approval of the US Food & Drug Administration of clinical trials using plasma for cancer treatment is a critical milestone and a sign that low temperature plasma may be on its way to be accepted as a promising and exciting healthcare technology. To learn more about the future directions of the field the reader is referred to references [83, 84]. In addition, low temperature plasma has obvious merits as a viable technology for space medicine. As deep-space long-duration space travel becomes a reality it is crucial to have available adequate methods to meet medical emergencies in space. In this context plasma offers a practical “energy-based” and “dry” technology that can replace perishable drugs.
Author Contributions
The author confirms being the sole contributor of this work and has approved it for publication.
Conflict of Interest
The author declares that the research was conducted in the absence of any commercial or financial relationships that could be construed as a potential conflict of interest.
References
1. Laroussi M. Sterilization of contaminated matter with an atmospheric pressure plasma. IEEE Trans Plasma Sci. (1996) 24:1188–91. doi: 10.1109/27.533129
2. Kelly-Wintenberg K, Montie TC, Brickman C, Roth JR, Carr AK, Sorge K, et al. Room temperature sterilization of surfaces and fabrics with a one atmosphere uniform glow discharge plasma. J Indust Microbiol Biotechnol. (1998) 20:69–74. doi: 10.1038/sj.jim.2900482
3. Laroussi M, Saylor G, Glascock B, McCurdy B, Pearce ME, Bright NG, et al. Images of biological samples undergoing sterilization by a glow discharge at atmospheric pressure. IEEE Trans Plasma Sci. (1999) 27:34−5. doi: 10.1109/27.763016
4. Herrmann HW, Henins I, Park J, Selwyn GS. Decontamination of Chemical and Biological Warfare (CBW) agents using an atmospheric pressure plasma jet. Phys Plasmas. (1999) 6:2284–9. doi: 10.1063/1.873480
5. Laroussi M, Alexeff I, Kang W. Biological decontamination by non-thermal plasmas. IEEE Trans Plasma Sci. (2000) 28:184–8. doi: 10.1109/27.842899
6. Laroussi M. Non-Thermal decontamination of biological media by atmospheric pressure plasmas: review, analysis, and prospects. IEEE Trans Plasma Sci. (2002) 30:1409–15. doi: 10.1109/TPS.2002.804220
7. Isbary G, Morfill G, Schmidt HU, Georgi M, Ramrath K, Heinlin J, et al. A first prospective randomized controlled trial to decrease bacterial load using cold atmospheric argon plasma on chronic wounds in patients. Br J Dermatol. (2010) 163:78. doi: 10.1111/j.1365-2133.2010.09744.x
8. Laroussi M. From killing bacteria to destroying cancer cells: twenty years of plasma medicine. Plasma Process Polym. (2014) 11:1138–41. doi: 10.1002/ppap.201400152
9. Metelmann HR, Nedrelow DS, Seebauer C, Schuster M, von Woedtke T, Weltmann K-D, et al. Head and neck cancer treatment and physical plasma. Clin Plasma Med. (2015) 3:17–23. doi: 10.1016/j.cpme.2015.02.001
10. Bartnikas R. Note on discharges in helium under AC conditions. J Appl Phys D Appl Phys. (1968) 1:659. doi: 10.1088/0022-3727/1/5/417
11. Donohoe KG. The development and characterization of an atmospheric pressure nonequilibrium plasma chemical reactor. (Ph.D Thesis). California Institute of Technology, Pasadena, CA, United States (1976).
12. Kanazawa S, Kogoma M, Moriwaki T, Okazaki S. Stable glow at atmospheric pressure. J Phys D Appl Phys. (1988) 21:838. doi: 10.1088/0022-3727/21/5/028
13. Yokoyama T, Kogoma M, Moriwaki T, Okazaki S. The mechanism of the stabilized glow plasma at atmospheric pressure. J Phys D Appl Phys. (1990) 23:1128. doi: 10.1088/0022-3727/23/8/021
14. Massines F, Mayoux C, Messaoudi R, Rabehi A, Ségur P. Experimental study of an atmospheric pressure glow discharge application to polymers surface treatment. In: Proceeding of GD-92, Vol. 2. Swansea (1992). p. 730–3.
15. Roth JR, Laroussi M, Liu C. Experimental generation of a steady-state glow discharge at atmospheric pressure. In: Proceeding of IEEE International Confrence Plasma Science. Tampa, FL (1992). p. 170−1.
16. Okazaki S, Kogoma M, Uehara M, Kimura Y. Appearance of a stable glow discharge in air, argon, oxygen and nitrogen at atmospheric pressure using a 50 Hz source. J Phys D Appl Phys. (1993) 26:889. doi: 10.1088/0022-3727/26/5/025
17. Massines F, Rabehi A, Decomps P, Gadri RB, Ségur P, Mayoux C. Experimental and theoretical study of a glow discharge at atmospheric pressure controled by a dielectric barrier. J Appl Phys. (1998) 8:2950. doi: 10.1063/1.367051
18. Gherardi N, Gouda G, Gat E, Ricard A, Massines F. Transition from glow silent discharge to micro-discharges in nitrogen gas. Plasma Sources Sci Technol. (2000) 9:340. doi: 10.1088/0963-0252/9/3/312
19. Gheradi N, Massines F. Mechanisms controlling the transition from glow silent discharge to streamer discharge in nitrogen. IEEE Trans Plasma Sci. (2001) 29:536. doi: 10.1109/27.928953
20. Shi JJ, Deng XT, Hall R, Punnett JD, Kong M. Three modes in a radio frequency atmospheric pressure glow discharge. J Appl Phys. (2003) 94:6303. doi: 10.1063/1.1622110
21. Massines F, Gherardi N, Naude N, Segur P. Glow and townsend dielectric barrier discharge in various atmosphere. Plasma Phys Contrl Fusion. (2005) 47:B557. doi: 10.1088/0741-3335/47/12B/S42
22. Kogelschatz U. Silent discharges for the generation of ultraviolet and vacuum ultraviolet excimer radiation. Pure Appl Chem. (1990) 62:1667. doi: 10.1351/pac199062091667
23. Kogelschatz U, Eliasson B, Egli W. Dielectric-Barrier discharges: principle and applications. J Physique IV. (1997) 7:47. doi: 10.1051/jp4:1997405
24. Fridman G, Friedman G, Gutsol A, Shekhter AB, Vasilets VN, Fridman A. Applied plasma medicine. Plasma Process Polym. (2008) 5:503. doi: 10.1002/ppap.200700154
25. Laroussi M. Low temperature plasmas for medicine? IEEE Trans Plasma Sci. (2009) 37:714. doi: 10.1109/TPS.2009.2017267
26. Weltmann KD, Kindel E, von Woedtke T, Hähnel M, Stieber M, Brandenburg R. Atmospheric-pressure plasma sources: prospective tools for plasma medicine. Pure Appl Chem. (2010) 82:1223. doi: 10.1351/PAC-CON-09-10-35
27. Laroussi M, Lu XP, Keidar M. Perspective: the physics, diagnostics, and applications of atmospheric pressure low temperature plasma sources used in plasma medicine. J. Appl Phys. (2017) 122:020901. doi: 10.1063/1.4993710
28. Mildren RP, Carman RJ. Enhanced performance of a dielectric barrier discharge lamp using short-pulsed excitation. J Phys D Appl Phys. (2001) 34:3378. doi: 10.1088/0022-3727/34/1/101
29. Stark R, Schoenback KH. Electron heating in atmospheric pressure glow discharges. J Appl Phys. (2001) 89:3568. doi: 10.1063/1.1351546
30. Laroussi M, Lu XP, Kolobov V, Arslanbekov R. Power consideration in the pulsed dbd at atmospheric pressure. J Applied Phys. (2004) 6:3028. doi: 10.1063/1.1777392
31. Laroussi M, Alexeff I, Richardson JP, Dyer FF. The resistive barrier discharge. IEEE Trans Plasma Sci. (2002) 30:158. doi: 10.1109/TPS.2002.1003972
32. Koinuma H, Ohkubo H, Hashimoto T. Development and application of a microbeam plasma generator. Appl Phys Lett. (1992) 60:816. doi: 10.1063/1.106527
33. Janca J, Klima M, Slavicek P, Zajickova L. HF plasma pencil – new source for plasma surface processing. Surf Coating Technol. (1999) 116–119:547–51. doi: 10.1016/S0257-8972(99)00256-X
34. Laroussi M, Lu XP. Room temperature atmospheric pressure plasma plume for biomedical applications. Appl Phys Letts. (2005) 87:113902. doi: 10.1063/1.2045549
35. Laroussi M, Akan T. Arc-free atmospheric pressure cold plasma jets: a review. Plasma Process Polym. (2007) 4:777–88. doi: 10.1002/ppap.200700066
36. Teschke M, Kedzierski J, Finantu-Dinu EG, Korzec D, Engemann J. High-Speed photographs of a dielectric barrier atmospheric pressure plasma jet. IEEE Trans Plasma Sci. (2005) 33:310. doi: 10.1109/TPS.2005.845377
37. Lu XP, Laroussi M. Dynamics of an atmospheric pressure plasma plume generated by submicrosecond voltage pulses. J Appl Phys. (2006) 100:063302. doi: 10.1063/1.2349475
38. Mericam-Bourdet N, Laroussi M, Begum A, Karakas E. Experimental investigations of plasma bullets. J Phys D Appl Phys. (2009) 42:055207. doi: 10.1088/0022-3727/42/5/055207
39. Sands BL, Ganguly BN, Tachibana K. A streamer-like atmospheric pressure plasma jet. Appl Phys Lett. (2008) 92:151503. doi: 10.1063/1.2909084
40. Walsh JL, Kong MG. Contrasting characteristics of linear-field and cross-field atmospheric plasma jets. Appl Phys Lett. (2008) 93:111501. doi: 10.1063/1.2982497
41. Xiong Q, Lu XP, Liu J, Xian Y, Xiong Z, Zou F, et al. Temporal and spatial resolved optical emission behaviors of a cold aatmospheric pressure plasma jet. J Appl Phys. (2009) 106:083302. doi: 10.1063/1.3239512
42. Karakas E, Koklu M, Laroussi M. Correlation between helium mole fraction and plasma bullet propagation in low temperature plasma jets. J Phys D Appl Phys. (2010) 43:155202. doi: 10.1088/0022-3727/43/15/155202
43. Naidis GV. Modeling of plasma bullet propagation along a helium jet in ambient air. J Phys D Appl Phys. (2011) 44:215203. doi: 10.1088/0022-3727/44/21/215203
44. Naidis GV. Modeling of streamer propagation in atmospheric pressure helium plasma jets. J Phys D Appl Phys. (2010) 43:402001. doi: 10.1088/0022-3727/43/40/402001
45. Yousfi M, Eichwald O, Merbahi N, Jomma N. Analysis of ionization wave dynamics in low-temperature plasma jets from fluid modeling supported by experimental investigations. Plasma Sources Sci Technol. (2012) 21:045003. doi: 10.1088/0963-0252/21/4/045003
46. Boeuf JP, Yang L, Pitchford L. Dynamics of guided streamer (plasma bullet) in a helium jet in air at atmospheric pressure. J Phys D Appl Phys. (2013) 46:015201. doi: 10.1088/0022-3727/46/1/015201
47. Karakas E, Laroussi M. Experimental studies on the plasma bullet propagation and its inhibiton. J Appl Phys. (2010) 108:063305. doi: 10.1063/1.3483935
48. Jarrige J, Laroussi M, Karakas E. Formation and dynamics of the plasma bullets in a non-thermal plasma jet: influence of the high voltage parameters on the plume characteristics. Plasma Sources Sci Technol. (2010) 19:065005. doi: 10.1088/0963-0252/19/6/065005
49. Sakiyama Y, Graves DB, Jarrige J, Laroussi M. Finite element analysis of ring-shaped emission profile in plasma bullets. Appl Phys Lett. (2010) 96:041501. doi: 10.1063/1.3298639
50. Begum A, Laroussi M, Pervez MR. Atmospheric pressure helium/air plasma jet: breakdown processes and propagation phenomenon. AIP Adv. (2013) 3:062117. doi: 10.1063/1.4811464
51. Stretenovic GB, Krstic IB, Kovacevic VV, Obradovic AM, Kuraica MM. Spatio-temporally resolved electric field measurements in helium plasma jet. J Phys D Appl Phys. (2014) 47:102001. doi: 10.1088/0022-3727/47/10/102001
52. Sobota A, Guaitella O, Garcia-Caurel E. Experimentally obtained values of electric field of an atmospheric pressure plasma jet impinging on a dielectric surface. J Phys D Appl Phys. (2013) 46:372001. doi: 10.1088/0022-3727/46/37/372001
53. Lu X, Naidis GV, Laroussi M, Reuter S, Graves DB, Ostrikov K. Reactive species in non-equilibrium atmospheric pressure plasma: generation, transport, and biological effects. Phys Rep. (2016) 630:1–84. doi: 10.1016/j.physrep.2016.03.003
54. Lietz AM, Kushner MJ. Molecular admixtures and impurities in atmospheric pressure plasma jets. J Appl Phys. (2018) 124:153303. doi: 10.1063/1.5049430
55. Winter J, Brandenburg R, Weltmann KD. Atmospheric pressure plasma jets: an overview of devices and new directions. Plasma Sources Sci Technol. (2015) 24:054001. doi: 10.1088/0963-0252/24/6/064001
56. Becker KH, Shoenbach KH, Eden JG. Microplasmas and applications. J Phys D Appl Phys. (2006) 39:R55. doi: 10.1088/0022-3727/39/3/R01
57. Lietz AM, Kushner MJ. Electrode configurations in atmospheric pressure plasma jets: production of reactive species. Plasma Sources Sci Technol. (2018) 27:105020. doi: 10.1088/1361-6595/aadf5b
58. Laroussi M. Sterilization of liquids using a plasma glow discharge. US Patent # 5,876,663 (1999).
59. Shekhter AB, Kabisov RK, Pekshev AV, Kozlov NP, Perov Yu L. Experimental and clinical validation of plasmadynamic therapy of wounds with nitric oxide. Bull Exp Biol Med. (1998) 126:829–34. doi: 10.1007/BF02446923
60. Stoffels E, Flikweert AJ, Stoffels WW, Kroesen GMW. Plasma needle: a non-destructive atmospheric plasma source for fine surface treatment of biomaterials. Plasma Sources Sci Technol. (2002) 11:383–88. doi: 10.1088/0963-0252/11/4/304
61. Stalder KR, Woloszko K, Brown IG, Smith CD. Repetitive plasma discharges in saline solutions. Appl Phys Lett. (2001) 79:4503. doi: 10.1063/1.1429752
62. Woloszko K, Stalder KR, Brown IG. Plasma characteristics of repetitively-pulsed electrical discharges in saline solutions used for surgical procedures. IEEE Trans Plasma Sci. (2002) 30:1376–83. doi: 10.1109/TPS.2002.801612
63. Laroussi M, Kong M, Morfill G, Stolz W. Plasma Medicine: Applications of Low-Temperature Gas Plasmas in Medicine and Biology. Cambridge: Cambridge Univ. Press. (2012).
65. Metelmann HR, von Woedtke T, Weltmann KD. Comprehensive Clinical Plasma Medicine. Berlin: Springer (2018).
66. Toyokuni S, Ikehara Y, Kikkawa F, Hori M. Plasma Medical Science. Cambridge, MA: Academic Press (2018).
67. Laroussi M, Richardson JP, Dobbs FC. Effects of non-equilibrium atmospheric pressure plasmas on the heterotrophic pathways of bacteria and on their cell morphology. Appl Phys Lett. (2002) 81:772–4. doi: 10.1063/1.1494863
68. Laroussi M, Mendis DA, Rosenberg M. Plasma interaction with microbes. N J Phys. (2003) 5:41.1–10. doi: 10.1088/1367-2630/5/1/341
69. Barekzi N, Laroussi M. Fibropblasts cell morphology altered by low temperature atmospheric pressure plasma. IEEE Trans Plasma Sci. (2014) 42:2738. doi: 10.1109/TPS.2014.2315787
70. Keidar M, Walk R, Shashurin A, Srinivasan P, Sandler A, Dasgupta S, et al. Cold plasma selectivity and the possibility of paradigm shift in cancer therapy. Br J Cancer. (2011) 105:1295. doi: 10.1038/bjc.2011.386
71. Barekzi N, Laroussi M. Effects of low temperature plasmas on cancer cells. Plasma Process Polym. (2013) 10:1039. doi: 10.1002/ppap.201300083
72. Keidar M, Shashurin A, Volotskova O, Stepp MA, Srinivasan P, Sandler A, et al. Cold atmospheric plasma in cancer therapy. Phys Plasmas. (2013) 20:057101. doi: 10.1063/1.4801516
73. Köritzer J, Boxhammer V, Schäfer A, Shimizu T, Klämpfl TG, Li YF, et al. Restoration of sensitivity in chemo-resistant glioma cells by cold atmospheric plasma. PLoS ONE. (2013) 8:e64498. doi: 10.1371/journal.pone.0064498
74. Vandamme M, Robert E, Pesnele S, Barbosa E, Dozias S, Sobilo J, et al. Antitumor effects of plasma treatment on U87 glioma xenografts: preliminary results. Plasma Process Polym. (2010) 7:264–73. doi: 10.1002/ppap.200900080
75. Tanaka H, Mizuno M, Ishikawa K, Nakamura K, Kajiyama H, Kano H, et al. Plasma-activated medium selectively kills glioblastoma brain tumor cells by down-regulating a survival signaling molecule, AKT kinase. Plasma Med. (2011) 1:265. doi: 10.1615/PlasmaMed.2012006275
76. Utsumi F, Kjiyama H, Nakamura K, Tanaka H, Mizuno M, Ishikawa K, et al. Effect of indirect nonequilibrium atmospheric pressure plasma on anti-proliferative activity against chronic chemo-resistant ovarian cancer cells in vitro and in vivo. PLoS ONE. (2013) 8:e81576. doi: 10.1371/journal.pone.0081576
77. Tanaka H, Mizuno M, Ishikawa K, Takeda K, Nakamura K, Utsumi F, et al. Plasma medical science for cancer therapy: toward cancer therapy using nonthermal atmospheric pressure plasma. IEEE Trans Plasma Sci. (2014) 42:3760. doi: 10.1109/TPS.2014.2353659
78. Mohades S, Barekzi N, Laroussi M. Efficacy of low temperature plasma against scaber cancer cells. Plasma Process Polym. (2014) 11:1150–55. doi: 10.1002/ppap.201400108
79. Laroussi M, Mohades S, Barekzi N. Killing of adherent and non-adherent cancer cells by the plasma pencil. Biointerphases. (2015) 10:029410. doi: 10.1116/1.4905666
80. Mohades S, Laroussi M, Sears J, Barekzi N, Razavi H. Evaluation of the effects of a plasma activated medium on cancer cells. Phys Plasmas. (2015) 22:122001. doi: 10.1063/1.4933367
81. Mohades S, Barekzi N, Razavi H, Maramuthu V, Laroussi M. Temporal evaluation of antitumor efficiency of plasma activated media. Plasma Process Polym. (2016) 13:1206. doi: 10.1002/ppap.201600118
82. Bauer G. Cold atmospheric plasma and plasma activated medium: antitumor cell effects with inherent synergistic potential. Plasma Med. (2019) 9:57–88. doi: 10.1615/PlasmaMed.2019029462
83. Weltmann KD, Kolb JF, Holub M, Uhrlandt D, Šimek M, Ostrikov K, et al. The future of plasma science and technology. Plasma Process Polym. (2019) 16:e1800118. doi: 10.1002/ppap.201800118
Keywords: plasma discharge, cold plasma, plasma jet, plasma medicine, cells, tissues, bacteria, cancer
Citation: Laroussi M (2020) Cold Plasma in Medicine and Healthcare: The New Frontier in Low Temperature Plasma Applications. Front. Phys. 8:74. doi: 10.3389/fphy.2020.00074
Received: 19 January 2020; Accepted: 03 March 2020;
Published: 20 March 2020.
Edited by:
Gianpiero Colonna, Italian National Research Council, ItalyReviewed by:
Milan Simek, Institute of Plasma Physics (ASCR), CzechiaKazuo Takahashi, Kyoto Institute of Technology, Japan
Copyright © 2020 Laroussi. This is an open-access article distributed under the terms of the Creative Commons Attribution License (CC BY). The use, distribution or reproduction in other forums is permitted, provided the original author(s) and the copyright owner(s) are credited and that the original publication in this journal is cited, in accordance with accepted academic practice. No use, distribution or reproduction is permitted which does not comply with these terms.
*Correspondence: Mounir Laroussi, bWxhcm91c3NAb2R1LmVkdQ==