- Dipartimento di Chimica e Tecnologie Chimiche, Università della Calabria, Rende, Italy
The photophysical properties of some monomeric and dimeric BODIPY systems were investigated at the density functional theory level and herein reported. In particular, the absorption spectra were fully characterized, low energy singlet and triplet excited states were discussed also focusing on the energy difference gaps between them and computing the spin-orbit couplings values for the possible intersystem crossing channels. The heavy atom effect of iodine substituents on the photophysical properties of a monomer and on a dimer under investigation was also estimated. Results obtained on the considered compounds allow us to predict which is the most promising candidate to be suggested as a photosensitizer in photodynamic therapy.
Introduction
In the last decade the BF2-chelated dipyrromethenes, known as BODIPYs, have been proposed for many applications in materials science and biotechnology because of their excellent stability, arising from the strongly electron-withdrawing BF2 group, intense absorption band in the near-infrared region, sharp emissions and quantum fluorescence yields [1–3]. Through appropriate modifications which permit a strong reduction in the fluorescence quantum yields while enhancing singlet-to-triplet intersystem crossing, BODIPY and their aza-derivatives have emerged as excellent photosensitizers in photodynamic therapy (PDT) [4–7]. PDT is a very old medical practice, especially employed in dermatology, but it is only recently being developed as a minimally invasive therapy in the treatment of different kinds of tumors [8–12]. Moreover, its clinical efficiency is currently under exploration for other diseases such as infections, cardiovascular and wounds healing [13–15]. The main cytotoxic agent in PDT is the excited singlet molecular oxygen generated in situ through the so-called photodynamic process. In the medical practice, after the administration and selective accumulation of the photosensitizer (PS) in the target tissue, the region is locally irradiated with an appropriate light source able to promote the excitation of the PS from the ground (S0) to an excited singlet electronic state Sn. In systems with low fluorescence quantum yield, the energy of the S1 can be transferred to an excited triplet state, T1, through a non-radiative S1→T1 intersystem crossing (ISC) process. If the energy of that state is greater than that required to excite the oxygen, instead of decaying in the ground state S0, the T1 can transfer its energy to trigger the O2 conversion (the so-called type II reaction). Consequently, the ability of a photosensitizer to generate singlet oxygen is strictly related to the generation of a high energy lying excited triplet T1 able to excite the molecular oxygen (0.98 eV) and to the occurrence of an efficient singlet-triplet ISC process. To satisfy these criteria, BODIPY dyes proposed for PDT can be modified following two ways: (i) including a heavy atom (I, Br, or a nontoxic metal) in their structures; (ii) performing a covalent dimerization. The first strategy exploits the heavy atoms to enhance the efficiency of the intersystem crossing whose constant depends on the amplitude of the spin-orbit couplings between the singlet and triplet states (the so-called heavy atom effect).
As for the second class of strategy, the mechanism through which the dimers should work is not clear from the few investigations hitherto appeared in the literature [16–19]. Very recently, BODIPY monomers and dimers with and without heavy atom in their structures were synthetized and their photophysical properties were accurately measured [16]. Using the experimental evidences given in that article as a starting point, we have undertaken a careful theoretical study of the structural and electronic characteristics of these systems (see Scheme 1) by using the density functional theory (DFT) and its time-dependent formulation (TDDFT). In particular, we determined the geometries of ground and excited states, the absorption features, the singlet-triplet energy gaps, and the spin orbit coupling matrix elements.
Computational Details
The investigation was performed by using the Density Functional Theory level (DFT) and its time-dependent density functional linear response formulation (TDDFT) as implemented in Gaussian09 code [20]. Geometry optimization (without imposing constrains) and harmonic vibrational frequencies were done by using the B3LYP [21, 22] exchange-correlation functional in conjunction with the 6-31G* basis set for all the atoms excepts Iodine, for which the SSD pseudopotential [23] was employed. The lowest 20 vertical excitation energies were calculated by TDDFT on the previously optimized geometries by adding a diffuse function to the basis set. Solvent effects were evaluated by using the non-equilibrium implementation [24] of the polarizable continuum model [25]. The dichloromethane (ε = 8.93) in which the experimental UV-Vis spectra were measured [16], was considered as solvent.
Spin–orbit matrix elements were computed with DALTON code [26] by using B3LYP functional and cc-pVDZ basis set for all the atoms except iodine, for which the coupled pseudopotential was considered. The atomic-mean field approximation [27] was used for the systems without iodine atoms while, for those in which this heavy atom is present, the Spin–Orbit Coupling Operators for Effective Core Potentials with an effective nuclear charge [28] was employed.
The spin–orbit couplings (SOCs) were evaluated according to the following formula:
where ĤSO is the spin–orbit Hamiltonian.
Results and Discussion
The main geometrical parameters resulting from the optimization process of examined systems, are shown in Table 1, while the coordinates of the obtained minima are listed in the Supplementary Information section (Tables S1–S6). For TMBDP-dimer, a comparison is possible with the X-ray structure recently reported [19]. The agreement with the experimental counterparts for bond lengths and valence angles, which do not vary significantly among the studied compounds, is very satisfactory. The more interesting parameter is the torsional angle Ψ that determines the position of the two linked BODIPY moieties. Values of 36.8, 86.9, and 75.3° for BDP-dimer, TMBDP-dimer and I2-TMBDP-dimer were obtained, respectively. This trend accounts for the high steric hindrance of the four methyl groups that force the two BODIPY sub-units to be almost orthogonal to each other in both TMBDP and I2-TMBDP dimers. This means that in these two molecules the electron delocalization is practically precluded as also confirmed by the shape of computed HOMO and LUMO orbitals, which shows a lack of mixing between the two orthogonal π moieties. Similar results were previously obtained, at CASSCF level of theory, for the TMBDP-dimer [19].
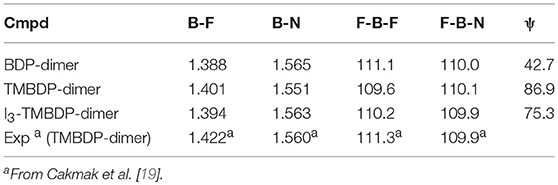
Table 1. Selected bond lengths [Å] and angles [deg] calculated at the B3LYP/6-31G* level for the dimers studied.
The computed TDDFT excitation energies for singlet and triplet states are collected in Table 2 together with the available experimental and previous theoretical results. As a general trend, we note that the TDDFT method underestimates the wavelengths of the singlet excited states by about 80 nm. This behavior was previously observed in other previous studies on BODIPY-like systems [29–32]. In a recent work, Le Guennic et al. have discussed the possible sources of this error and proposed a correction based on coupled cluster computations [33]. The agreement seems to be satisfactory for the T1 energies. In fact, the available experimental transition energy value determined for I3-TMBDP-dimer [16] is close to that obtained at TDDFT level (1.59 vs. 1.50 eV). On the other hand, the S1 and T1 excitation values, computed at CASSCF level, for TMBDP-dimer, are significantly overestimated with respect to the experimental ones (see Table 2). From that table, one can readily see that in proceeding from the monomeric to the dimeric systems, the absorption wavelength is red-shifted. Also, the iodinated compounds suffer a small red shift in comparison with the analogs without iodine substituents. The S1 oscillator strength in the monomeric systems assumes high values, while in the dimeric structures, it seems to be almost silent. The electronic structure of S1 was previously studied at both DFT [16] and CASCF [19] levels. In the former study, a photo induced electron transfer is suggested on the basis of the HOMO and LUMO orbital composition of the S0 optimized ground state, while in the latter study, S1 wave function is proposed to derive by a double substitution and natural orbital occupation numbers show four odd electrons in four similar orbitals. Our computed S1 frontier orbital picture is very similar to that reported by Zhang [16] for the ground state. Of course, we cannot exclude that the higher level Interaction Configuration computations might better characterize the electronic structure of these systems. The lowest lying T1 excitations are due to HOMO → LUMO transitions in the monomeric systems (BDP, TMBDP and I2- TMBDP) while, in the dimers, also other Gouterman molecular orbital play an important role (see Table 2).
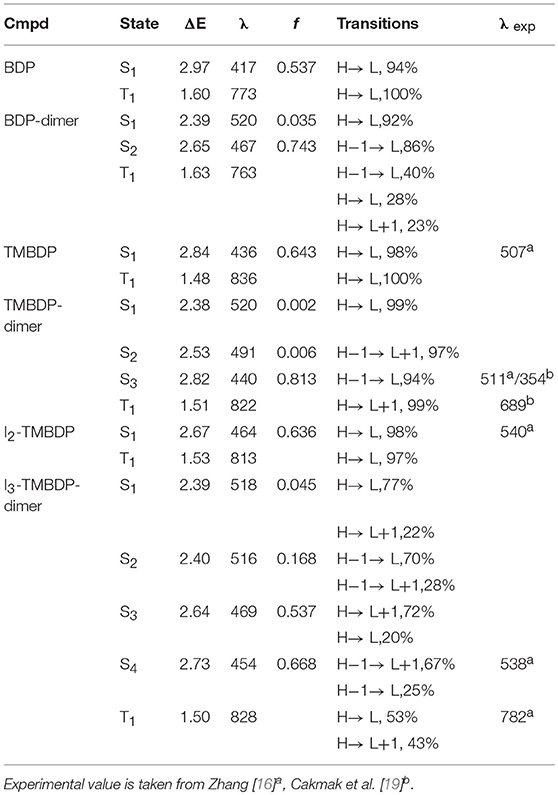
Table 2. Vertical excitation energies, ΔE (eV), λmax (nm), oscillator strengths f and main transitions for studied compounds computed in dichloromethane by using B3LYP/6-31+G*.
As previously mentioned, an efficient PDT photosensitizer must possess a ΔES0−T1 energy gap higher than 0.98 eV to excite the molecular oxygen from its ground to the highly cytotoxic excited singlet state. All the studied molecules have energy gaps sensibly higher than 0.98 eV and hence are in principle good candidates as PDT drugs (see Table 2).
The computed spin-orbit coupling constants for the transitions involving the S1 and the T1, together with the corresponding singlet-triplet energy gaps and the available singlet oxygen quantum yields (ΦΔ) are reported in Table 3. For BDP and its tetrametilate analog (TMBDP), the |〈ΨS1|Ĥso|ΨT1〉| spin orbit coupling matrix element has a negligible magnitude. I In the corresponding dimeric form the coupling increases, attaining a magnitude of about 1 cm−1. IIn the iodine containing systems, the SOCs become 9.44 and 8.67 cm−1 for I2-TMBDP and I3-TMBDP-dimer, respectively. The small variation of the SOCs in proceeding from the monomeric (BDP, TMBDP) to their respective dimeric forms (BDP-dimer, TMBDP-dimer), is attributable to the slight variation of the molecular orbitals involved in the transitions (see Figure 1). As in other systems previously studied [34–41], the presence of the heavy atom substantially increases the spin-orbit coupling matrix elements. This is due to the so-called heavy atom effect. Looking at the Figure 1, one can note that in the case of the compound I2-TMBDP, the HOMO→ LUMO transition has a π-π nature, while the one involved in T1 has a π-n nature since the LUMO orbital is essentially composed by the iodine lone pairs. This is in agreement with the El Sayed rules [42] for which spin-orbit couplings increase if there is a change in the composition of the molecular orbitals involved in a given transition. For I3-TMBDP-dimer the situation is different since the excited state T1 is due to two transitions: HOMO → LUMO (53%) and HOMO → LUMO+1 (43%) (see Table 2). In the former circumstance, HOMO and LUMO orbitals are localized each on the BODIPY fragments and the resulting transition, thus, has a π-π character (See Figure 2). Nevertheless, LUMO + 1 is mainly localized on the lone pairs of iodine atoms. Consequently, the second transition is π-n in nature and, according to the El Sayed rules, contributes to increasing the value of the relative SOC.
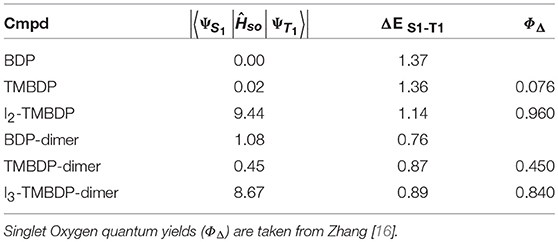
Table 3. Spin–orbit matrix elements (cm−1) and singlet-triplet energy gaps (eV) calculated at the B3LYP/6-31G(d) level of theory.
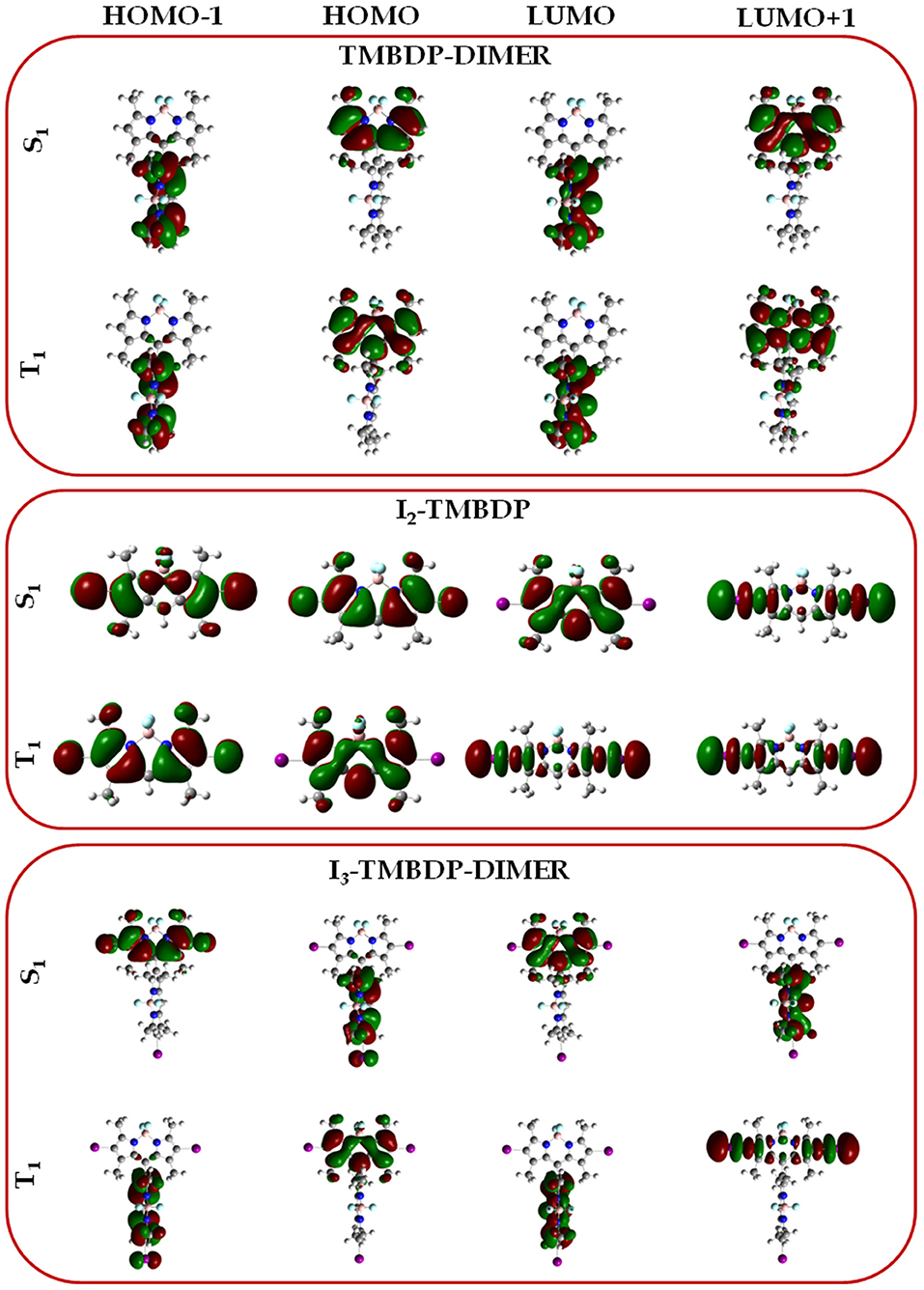
Figure 1. Molecular orbital composition for the excited states involved in the singlet and triplet excitation for TMBDP-dimer, I2-TMBDP, and I3-TMBDP-dimer systems.
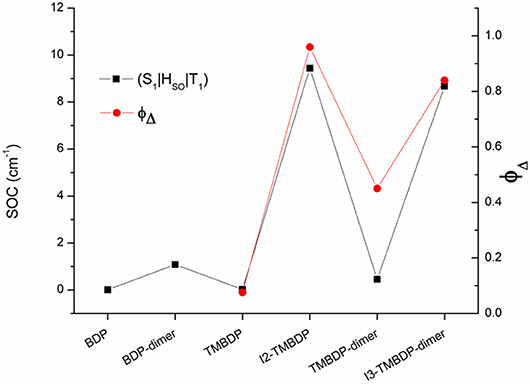
Figure 2. Computed SOCs (cm−1) for S1-T1 radiationless transitions and measured ΦΔ (from [16]) for the investigated systems.
In order to verify whether our SOCs values agree with the available experimental data, we have plotted (Figure 2) the computed SOCs and the available experimental singlet oxygen quantum yields [16]. Inspection of the Figure shows that the correlation between the two set of data is quite good and the behaviors are very similar. In fact, as the values of the spin-orbit coupling constant increase, there is an increase in the production of singlet oxygen. The only significant deviation was found for the TMBDP-dimer, for which the calculated SOCs don't follow the same trend of the experimental singlet oxygen quantum yields. Anyway, it is worth of note that, according to the Fermi Golden Rule [43], the intersystem crossing kinetics is directly related to the spin-orbit matrix elements but it also depends on the Franck–Condon weighted density of states (FCWD), not considered in our investigation. For the TMBDP-dimer, thus, we can speculate that the missed FCWD could play a role in the determination of the ISC kinetic constant.
According to the Kasha rule [44], the transition Si → S1 (with i > 1) should be a very fast process, and triplet state population should start from the lowest singlet exited state (S1). For those systems for which different paths are possible, we can suggest the most feasible ISC pathways for the population of the lowest triplet states on the basis of the obtained SOC values. In all the examined cases, the favored process implies the fast decay via IC in the low-lying excited singlet state with subsequent ISC to the T1 triplet states, as follows:
The fastest process occurs in iodinated systems for which high SOC values were computed for the S1-T1 channel, which should ensure an efficient S1-T1 intersystem crossing.
Conclusions
In this DFT-based investigation, the main photophysical properties required to propose BODIPY dimers as possible photosensitizers in photodynamic therapy were explored. On the basis of our study, the following conclusions can be outlined:
- All the examined systems show absorption wavelengths that fall in the lower part of the so-called therapeutic window (500–600 nm).
- The spin-orbit coupling constants for BDP and TMBDP dimeric compounds were found to be slightly higher than those computed for their corresponding monomers. Their magnitudes are close to that of FOSCAN®, which is already used in PDT medical protocols -The systems with the largest SOC values are those containing iodine heavy atoms. Because these structures possess a more efficient singlet-triplet intersystem crossing, they are also expected to more efficiently produce the singlet O2 () species, which is the main cytotoxic agent in PDT based on type II reactions.
We hope that our investigation can stimulate further experimental studies on these promising systems.
Author Contributions
MA, BD, NR, ES, MT equally contributed to the present version of the paper. MA, BD, NR, ES, MT analyzed the results, edited and reviewed equally the manuscript and approved it for publication.
Conflict of Interest Statement
The authors declare that the research was conducted in the absence of any commercial or financial relationships that could be construed as a potential conflict of interest.
Acknowledgments
Financial support from the Università degli Studi della Calabria-Dipartimento di Chimica e Tecnologie Chimiche (CTC) is acknowledged.
Supplementary Material
The Supplementary Material for this article can be found online at: https://www.frontiersin.org/articles/10.3389/fphy.2018.00143/full#supplementary-material
References
1. Kowada T, Maeda H, Kikuchi K. BODIPY-based probes for the fluorescence imaging of biomolecules in living cells. Chem Soc Rev. (2015) 44:4953–72. doi: 10.1039/C5CS00030K
2. Boens N, Leen V, Dehaen W. Fluorescent indicators based on BODIPY. Chem Soc Rev. (2012) 41:1130–72. doi: 10.1039/C1CS15132K
3. Loudet A, Burgess K. BODIPY dyes and their derivatives: syntheses and spectroscopic properties. Chem Rev. (2007) 107:4891–932. doi: 10.1021/cr078381n
4. Kamkaew A, Lim SH, Lee HB, Voon Kiew L, Chung LY, Burgess K. BODIPY dyes in photodynamic therapy. Chem Soc Rev. (2013) 42:77–88. doi: 10.1039/C2CS35216H
5. Zhao J, Xu K, Yang W, Wang Z, Zhong F. The triplet excited state of Bodipy: formation, modulation and application. Chem Soc Rev. (2015) 44:8904–39. doi: 10.1039/C5CS00364D
6. Awuahab SG, You Y. Boron dipyrromethene (BODIPY)-based photosensitizers for hotodynamic therapy. RSC Adv. (2012) 2:11169–83. doi: 10.1039/c2ra21404k
7. Gorman A, Killoran J, O'Shea C, Kenna T, Gallagher WM, O'Shea DF. In vitro demonstration of the heavy-atom effect for photodynamic therapy. J Am Chem Soc. (2004) 126:10619–31. doi: 10.1021/ja047649e
8. Dabrowski JM, Arnaut LG. Photodynamic therapy (PDT) of cancer: From local to systemic treatment. Photochem Photobiol Sci. (2015) 14:1765–80. doi: 10.1039/C5PP00132C
9. Quirk BJ, Brandal G, Donlon S, Vera JC, Mang TS, Foy AB, et al. Photodynamic therapy (PDT) for malignant brain tumors–where do we stand? Photodiagn Photodyn Ther. (2015) 12:530–44. doi: 10.1016/j.pdpdt.2015.04.009
10. Dolmans DEJGJ, Fukumura D, Jain RK. Photodynamic therapy for cancer. Nat Rev Cancer (2003) 3:380–7. doi: 10.1038/nrc1071
11. Yano S, Hirohara S, Obata M, Hagiya Y, Ogura SI, Ikeda A, et al. Current states and future views in photodynamic therapy. J Photochem Photobiol C (2011) 12:46–67. doi: 10.1016/j.jphotochemrev.2011.06.001
12. Agostinis P, Berg K, Cengel KA, Foster TH, Girotti AW, Gollnick SO, et al. Photodynamic therapy of cancer: an update. CA Cancer J Clin. (2011) 61:250–81. doi: 10.3322/caac.20114
13. Babilas P, Schreml S, Landthaler M, Szeimies RM. Photodynamic therapy in dermatology: state-of-the-art. Photodermatol Photoimmunol Photomed. (2010) 26:118–32. doi: 10.1111/j.1600-0781.2010.00507.x
14. Kossodo S, LaMuraglia GM. Clinical potential of photodynamic therapy in cardiovascular disorders. Am J Cardiovasc Drugs (2001) 1:15–21. doi: 10.2165/00129784-200101010-00002
15. Garrier J, Bezdetnaya L, Barlier C, Grafe S, Guillemin F, D'Hallewin MA. Foslip®-based photodynamic therapy as a means to improve wound healing. Photodiagn Photodyn Ther. (2011) 8:321–7. doi: 10.1016/j.pdpdt.2011.06.003
16. Zhang X-F. BODIPY photosensitizers based on PET and heavy atom effect: a comparative study on the efficient formation of excited triplet state and singlet oxygen in BODIPY dimers and monomers. J Photochem Photobiol A Chem. (2018) 355:431–43. doi: 10.1016/j.jphotochem.2017.07.019
17. Zhang X-F, Yang X. Photosensitizer that selectively generates singlet oxygen in nonpolar environments: photophysical mechanism and efficiency for a covalent BODIPY dimer. J Phys Chem B (2013) 117:9050–5. doi: 10.1021/jp405102m
18. Pang W, Zhang X-F, Zhou J, Yu C, Hao E, Jiao L. Modulating the singlet oxygen generation property of meso-beta directly linked BODIPY dimers. Chem Commun. (2012) 48:5437–9. doi: 10.1039/c2cc30915g
19. Cakmak Y, Kolemen S, Duman S, Dede Y, Dolen Y, Kilic B, et al. Designing excited states: theory-guided access to efficient photosensitizers for photodynamic action. Angew Chem. (2011) 123:12143–7. doi: 10.1002/ange.201105736
20. Frisch MJ, Trucks GW, Schlegel HB, Scuseria GE, Robb MA, Cheeseman JR, et al. Gaussian 09, Revision D.01. Wallingford, CT: Gaussian, Inc. (2009).
21. Becke AD. Density-functional thermochemistry. III. The role of exact exchange. J Chem Phys. (1993) 98:5648–52. doi: 10.1063/1.464913
22. Lee C, Yang W, Parr RG. Development of the Colle-Salvetti correlation-energy formula into a functional of the electron density. Phys Rev B Condens Matter Mater Phys. (1988) 37:7859. doi: 10.1103/PhysRevB.37.785
23. Andrae D, Haussermann U, Dolg M, Stoll H, Preuss H. Energy-adjusted ab initio pseudopotentials for the second and third row transition elements. Theor Chim Acta (1990) 77:123–41. doi: 10.1007/BF01114537
24. Cossi M, Barone V. Solvent effect on vertical electronic transitions by the polarizable continuum model. J Chem Phys. (2000) 112:2427–35. doi: 10.1063/1.480808
25. Tomasi J, Menucci B, Cammi R. Quantum mechanical continuum solvation models. Chem Rev. (2005) 105:2999–3094. doi: 10.1021/cr9904009
26. DALTON. A Molecular Electronic Structure Program. Release Dalton 2011. Available online at: http://daltonprogram.org/ (Accessed February 24, 2016).
27. Ruud K, Schimmelpfennig B, Ågren H. Internal and external heavy-atom effects on phosphorescence radiative lifetimes calculated using a mean-field spin-orbit Hamiltonian. Chem Phys Lett. (1999) 310:215–21. doi: 10.1016/S0009-2614(99)00712-5
28. Koseki S, Schmidt MW, Gordon MS. Effective nuclear charges for the first- through third-row transition metal elements in spin–orbit calculations. J Phys Chem A (1998) 102:10430–6. doi: 10.1021/jp983453n
29. De Simone BC, Mazzone G, Pirillo J, Russo N, Sicilia E. Halogen atom effect on the photophysical properties of substituted aza-BODIPY derivatives. Phys Chem Chem Phys. (2017) 19:2530–6. doi: 10.1039/C6CP07874E
30. Quartarolo AD, Sicilia E, Russo N. On the potential use of squaraine derivatives as photosensitizers in photodynamic therapy: a TDDFT and RICC2 survey. J Chem Theory Comput. (2009) 5:1849–57. doi: 10.1021/ct900199j
31. De Simone BC, Mazzone G, Russo N, Sicilia E, Toscano M. Excitation energies, singlet–triplet energy gaps, spin–orbit matrix elements and heavy atom effects in BOIMPYs as possible photosensitizers for photodynamic therapy: a computational investigation. Phys Chem Chem Phys. (2018) 20:2656–61. doi: 10.1039/C7CP06763A
32. Alberto ME, De Simone BC, Mazzone G, Quartarolo AD, Russo N. Theoretical determination of electronic spectra and intersystem spin–orbit coupling: the case of isoindole-BODIPY dyes. J Chem Theory Comput. (2014) 10:4006–13. doi: 10.1021/ct500426h
33. Le Guennic B, Scalmani G, Frisch MJ, Laurent AD, Jacquemin D. Investigating the optical properties of BOIMPY dyes using ab initio tools. Phys Chem Chem Phys. (2017) 19:10554–61. doi: 10.1039/C7CP01190C
34. Ji S, Ge J, Escudero D, Wang Z, Zhao J, Jacquemin D. Molecular structure–intersystem crossing relationship of heavy-atom-free BODIPY triplet photosensitizers. J Org Chem. (2015) 80:5958–63. doi: 10.1021/acs.joc.5b00691
35. Ponte F, Mazzone G, Russo N, Sicilia E. BODIPY for photodynamic therapy applications: computational study of the effect of bromine substitution on 1O2 photosensitization. Journal of Mol Model. (2018) 24:183. doi: 10.1007/s00894-018-3727-3
36. De Simone BC, Mazzone G, Russo N, Sicilia E, Toscano M. Computational investigation of the influence of halogen atoms on the photophysical properties of tetraphenylporphyrin and its Zinc(II) complexes. J Phys Chem A (2018) 122:2809–15. doi: 10.1021/acs.jpca.8b00414
37. De Simone BC, Mazzone G, Russo N, Sicilia E, Toscano M. Metal atom effect on the photophysical properties of Mg(II), Zn(II), Cd(II), and Pd(II) tetraphenylporphyrin complexes proposed as possible drugs in photodynamic therapy. Molecules (2017) 22:1093. doi: 10.3390/molecules22071093
38. Alberto ME, De Simone BC, Mazzone G, Marino T, Russo N. Photophysical properties of free and metallated mesosubstituted tetrabenzotriazaporphyrin from density functional theory investigation. Dyes Pigm. (2015) 120:335–9. doi: 10.1016/j.dyepig.2015.04.032
39. Pirillo J, Mazzone G, Russo N, Bertini L. Photophysical properties of S, Se and Te-substituted deoxyguanosines: insight into their ability to act as chemotherapeutic agents. J Chem Inf Model. (2017) 57:234–42. doi: 10.1021/acs.jcim.6b00486
40. Alberto ME, Iuga C, Quartarolo AD, Russo N. Bisanthracene Bis(dicarboxylic imide)s as potential photosensitizers in Photodynamic therapy. A theoretical investigation. J Chem Inf Model. (2013) 53:2334–40. doi: 10.1021/ci4003365
41. Alberto ME, Russo N, Adamo C. Synergistic effects of metals in a promising RuII–PtIIAssembly for a combined anticancer approach: theoretical exploration of the photophysical properties. Chem Eur J (2016) 22:9162–8. doi: 10.1002/chem.201601089
42. El-Sayed MA. Triplet state. Its radiative and nonradiative properties. Acc Chem Res. (1968) 1:8–16. doi: 10.1021/ar50001a002
43. Marian CM. Spin–orbit coupling and intersystem crossing in molecules. Wiley Interdiscip Rev Comput Mol Sci. (2012) 2:187–203. doi: 10.1002/wcms.83
Keywords: TDDFT, PDT, BODIPY, heavy atom effect, spin-orbit coupling
Citation: Alberto ME, De Simone BC, Russo N, Sicilia E and Toscano M (2018) Can BODIPY Dimers Act as Photosensitizers in Photodynamic Therapy? A Theoretical Prediction. Front. Phys. 6:143. doi: 10.3389/fphy.2018.00143
Received: 10 October 2018; Accepted: 30 November 2018;
Published: 14 December 2018.
Edited by:
Yanfang Feng, Harvard Medical School, United StatesReviewed by:
Mohammad Ahsan Saad, Massachusetts General Hospital, United StatesPaul Cumming, University of Bern, Switzerland
Copyright © 2018 Alberto, De Simone, Russo, Sicilia and Toscano. This is an open-access article distributed under the terms of the Creative Commons Attribution License (CC BY). The use, distribution or reproduction in other forums is permitted, provided the original author(s) and the copyright owner(s) are credited and that the original publication in this journal is cited, in accordance with accepted academic practice. No use, distribution or reproduction is permitted which does not comply with these terms.
*Correspondence: Bruna Clara De Simone, YnJ1bmEuZGVzaW1vbmVAdW5pY2FsLml0
Nino Russo, bnJ1c3NvQHVuaWNhbC5pdA==