- Department of Biochemistry, Instituto de Química, Universidade de São Paulo, São Paulo, Brazil
Red light (600–700 nm, ∼2.1–1.8 eV) consists of a low-energy radiation with a high capacity to penetrate the skin and to induce stimulatory effects. These characteristics make this wavelength range very promising for light-based therapies. Aiming to discuss the mechanisms of action of photobiomodulation, we start by providing a broad perspective of the skin and of its interaction with light, focusing on the endogenous photosensitizers, on the formation of excited states and reactive oxidants, and on the activation of signaling effectors. The peculiar aspect of the photons in the red spectral range is that they are much less absorbed by the endogenous photosensitizers and consequently generate a lot fewer reactive oxidants (when compared with the other ranges of visible light), allowing the skin to mainly experience the consequences of several signaling pathways that are activated during the skin interaction with red-light photons. Indeed, the effects of red light in epithelial cells involve the control of metabolic responses, the modulation of several key genes and transcriptions factors, as well as the regulation of the intracellular nitric oxide stocks. In this article, we discuss how red light interacts with all these variables and end up causing a vigorous tissue activation. We also analyzed the effect of red-light photons on the nitric oxide homeostasis, with implications for the phototherapy of psoriasis. It is likely that several of the observations and mechanisms described for the interactions of red-light photons may also be occurring during and after the interaction with other photons with similar energy.
Interactions of red-light photons with the endogenous photosensitizers
The skin is made up of three layers: epidermis, dermis, and hypodermis. Light reaches different depths of the skin, depending on the wavelength of radiation, the optical properties of the skin, and on the characteristics of the absorbing species present in each skin layer. Endogenous absorbers, here called the endogenous photosensitizers (EP) are present in specific concentrations and locations. They absorb radiation and generate excited states and reactive oxidants that are ultimately responsible for the different skin responses during and after sun exposure or irradiation protocols (Bastos et al., 2023).
The propagation and penetration of light photons through the skin depend on several physical phenomena, including reflection, scattering, and absorption. Approximately 4%–7% of the incident photons are reflected by the skin surface, regardless of the incident wavelength and pigmentation. Most of light scattering is induced when incident photons interact with filamentous proteins and pigments. Keratin, collagen, melanin, and hemoglobin (by light absorption) are the main skin molecules affecting light penetration in human tissue. However, many others also contribute by absorbing light. The main EP present in human skin include small molecules, such as vitamins (nicotinamide, flavin, folate), amino acids, cofactors, bilirubin, β-carotene and macromolecules, such as proteins, nucleic acids, glycans, and opsins (de Assis et al., 2021).
The direct consequence of light absorption by the EP is the formation of excited states, which are usually much more reactive than their respective ground state counterparts (Bastos et al., 2023). We can categorize excited-state reactions as direct photochemistry, where the excited states react directly with surrounding molecules, and photosensitized oxidation, where the excited states activate other molecules that become reactive and oxidize surrounding molecules. Meanwhile, the original photosensitizer typically returns to the ground state and becomes available to absorb another photon. Among several classifications and definitions of the photosensitized oxidations, there are two competing mechanisms called Type I and Type II. The Type I mechanism involves contact-dependent reactions, i.e., triplet excited states abstracting or donating electrons or hydrogen atoms to and from surrounding molecules. In Type II mechanisms, there is always an energy transfer reaction with molecular oxygen forming singlet oxygen (1O2), a contact-independent pathway with high reactivity towards electron-rich compounds (Figure 1). In the contact-dependent pathway, damage occurs specifically at the site where the photosensitizer (PS) is located. In Type II photosensitized oxidation, the sites of reaction depend on the process of reaction and diffusion of 1O2. Type I and Type II mechanisms can occur simultaneously, with the predominant mechanism being defined according to the substrate, the distance between the PS and the targets, and the oxygen concentration (Baptista et al., 2021).
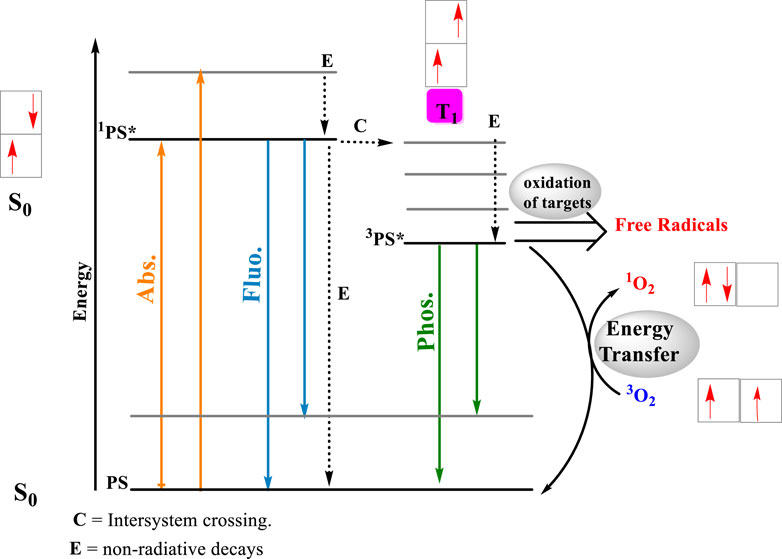
Figure 1. Photosensitized oxidation reactions. After the light absorption by the photosensitizer, a singlet excited state is formed almost instantaneously. A ground and state of the photosensitizer (PS), forms an electronically excited single state, 1PS * (Abs.). The energy absorbed by the PS will return to the surrounding media either by the emission of fluorescence (Fluo.) or through the release of heat (processed labeled as E). In the singlet excited state, the photosensitizing molecule can be converted to a triplet excited state (3PS*) by intersystem crossing (ISC), inducing a multiple change in the reactivity and lifetime of the PS (process C). The most impacting change is substantial increase in the lifetime of the triplet excited state, whose decay to the ground state involves a forbidden reaction. Longer lifetimes, means better chances to react by either type I (electron transfer) or type II (power transfer) mechanisms. In the electron transfer route, 3PS * interaction with biological substrate, triggering radical formations and radical ions, which later interact with molecular oxygen, producing reactive species, such as hydroxyl radical (OH⋅), superoxide ions (O2−⋅) and hydrogen peroxide (H2O2). On the energy transfer route, the 3PS * energy is transferred to molecular oxygen, producing single oxygen (1O2). Arrows in orange identify photon absorption, in blue, photon emission by fluorescence and in green, photon emission by phosphorescence.
Both Type I and Type II mechanisms cause biomolecular damage and ultimately induce the loss of biological function. In living systems, Type I and Type II mechanisms usually work synergistically. For example, it has been shown that membrane damage depends on the formation of alkoxyl groups. Membrane permeabilization is triggered by aldehyde production, which occurs through the direct reaction between the excited triplet state of PS and targets in the lipid membrane, such as lipid unsaturation and -OOH groups formed after the initial “ene” reaction. -OOH groups are the main result of the reaction between 1O2 and the lipid double bond.
These oxidation processes invariably lead to molecular damage. Therefore, an important question we aim to answer in this review is why red-light photons cause stimulatory effects? It is very likely that we know only a small part of the mechanisms that are activated during the interaction of red-light photons with the skin. However, we know enough to propose explanations that are detailed below. Nevertheless, the answer to this question starts with a simple observation. Red light photons penetrate a lot more in the skin, compared with blue and green light photons (De Assis et al., 2021). At a certain limit, the explanation to this observation is also simple, red-light photons are less scattered and are also less absorbed by the EP. Less light absorption means formation of fewer excited states with smaller amounts of reactive oxidants and smaller damage to the biological architecture. The total amount that is induced is seven times less than the amount of these species generated in other regions of the visible light (Zastrow et al., 2009). At the same time, there is activation of several signaling pathways, such as the activation of the Nrf2 pathway, which shifts the metabolic processes within the cells, leading to advantages conditions to overpasses external challenges (de Assis et al., 2021). Therefore, smaller absorption of red-light photons by the EP allows the skin to experience more of the stimulatory effects induced by the light-skin interactions. In this review, we do not aim to discuss the effects of UV, blue, and green light photons, instead we will focus on the effects of red-light photons.
The skin has a window of optical wavelength (600–1,300 nm) in which the absorption coefficients of melanin, hemoglobin, and water are the smallest. The reactions favoring the formation of reactive oxidants decrease with the increase in wavelength, since fewer EP are present in human skin in this optical window. Photobiomodulation affects the skin according to the hormetic paradigms. Hormesis is the principle that an agent, substance, stimulus, or condition can induce a biphasic dose-response (Felician et al., 2023). At lower light fluencies, there are stimulating effects, while at higher fluencies, there are inhibitory effects.
Human skin has a vigorous network of antioxidant molecules and enzymes, whose concentrations are tightly regulated. The generation of reactive oxidants by light absorption affects redox homeostasis in complex ways (Schalka et al., 2022). In general terms, high concentrations of radicals will overwhelm the antioxidant responses, leading to oxidative failure of the redox networks and causing a widespread oxidative damage, a condition that has been called oxidative distress (Sies and Jones, 2020). Depending on where the radicals and oxidants accumulate, specific damage may occur, for example, in important organelles such as mitochondria and lysosomes of the skin cells. This can affect all the biochemical processes triggered by these organelles, including cell metabolism, cell differentiation, autophagy, and immunity (Sena and Chandel, 2012).
The molecular mechanisms of the stimulatory effects are still not completely understood. Activation of mitochondria surely plays a preeminent role, being consistently correlated with the effects of red and infrared light exposure. Because of its key position in the electron transport chain of mitochondria, it has been proposed that the stimulatory effects of red light are due to the light absorption by an enzyme called cytochrome C oxidase. However, recent literature has shown that this hypothesis is not valid, and surely cytochrome c oxidase is not the only molecule involved (Sommer, 2019). Nevertheless, there are many possibilities to explain the stimulatory effects of red light, including several other heme-based proteins, opsins, nitric oxide.
Perhaps the best-known intermediate of the photobiomodulation effect involves nitric oxide (NO). There are consistent reports of increase in NO levels in irradiated tissues (Gebremendhin et al., 2021). NO can induce vasodilation (Ahmad et al., 2018), inhibit lipid peroxidation reactions (Hogg and Kalyanaraman, 1999; Suschek et al., 2001), stimulate insulin secretion by releasing calcium from mitochondria (Laffranchi et al., 1995), activating ion channels such as the large conductance potassium channel (Gebremendhin et al., 2021) and TRP channels through cysteine S-nitrosylation (Yoshida et al., 2006; Gambino et al., 2022), explaining several responses of the skin to sun and red-light exposure (McGarr, et al., 2023). Calcium levels, for example, can regulate mitochondrial respiration (Vilas-Boas et al., 2023), which could be a hallmark of red-light exposure in different cell types (Figure 2).
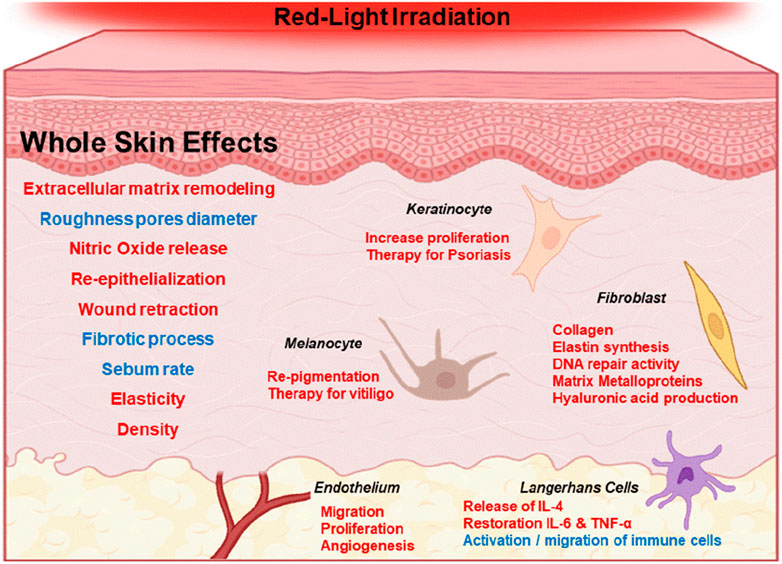
Figure 2. Generalized effects of red-light irradiation of the skin (600–700 nm). Red and blue fonts represent increase and decrease of specific effects, respectively. For example, red light stimulus is correlated with the increase of NO release and with the decrease in the fibrotic process.
Metabolic responses of skin cells induced by red light exposure
The skin is a very complex tissue composed of different cell types (mainly fibroblasts, keratinocytes, and melanocytes, but also Merkel and Langerhans cells) that contribute to the homeostasis of the organism and is responsible to start the biochemical responses during and after red light exposure. In the context of sun exposure, our skin interacts with photons of different energies at various depths, activating different responses to cope with the challenges caused by light absorption. Indeed, the skin has a robust network of redox reactions and redox-active species, known as the skin redoxome, which determines the redox environment of cells and tissues, coordinating signaling cascades and the expression of many genes and transcription factors (Schalka et al., 2022).
In terms of specific irradiation protocols of photobiomodulation, different studies have focused on the esthetic applications of red-light irradiation (600–700 nm), showing that doses up to 15–20 J/cm2 typically increases various skin rejuvenation parameters. These include enhancing elasticity and density, reducing roughness and pore diameter, and normalizing sebum production rates (Couturaud et al., 2023). Red light exposure also induces higher re-epithelialization and wound retraction, particularly in advanced repair phases (Simões et al., 2020), and is beneficial for the recovery of the skin barrier function (Abe et al., 2019). Activation of cell proliferation, independent of cell type and light source (ranging from 600 to 900 nm), is undoubtedly a significant hallmark of photobiomodulation (Glass, 2021; Brondon et al., 2009; Tang et al., 2023).
These effects on the skin have been mainly associated with the stimulatory effect of red light on fibroblast cell lines, leading to metabolic activation (George et al., 2018) and increasing the synthesis of collagen, elastin, and hyaluronic acid (Abergel et al., 1987; Kim et al., 2016; Kim B. et al., 2019). Red light exposure also has several other modulatory effects on fibroblasts, such as enhancing DNA repair (Kim et al., 2017). Umino and Denda established that keratinocytes also respond to red light exposure by increasing respiration rate associated to the increase in the mitochondrial activity. There was an evident increase in the rate of cellular proliferation (Umino and Denda, 2023). Interestingly, even cells lacking citochromo c oxidase show the typical photobiomodulation effect of enhancing cell proliferation after red-light irradiation. (Lima et al., 2019).
The responses observed in melanocytes are not as well understood as those in fibroblasts and keratinocytes. Depending on the experimental setting, different authors have observed inhibition or activation after red light exposure. Red light irradiation has been used for re-pigmentation therapy in vitiligo, based on the stimulation of melanocytes (Macedo et al., 2012; Yu et al., 2003). On the other hand, some authors correlate red light exposure (∼20 J/cm2) with reductions in melanin content, tyrosinase activity, and cell viability (Oh et al., 2017; Chen et al., 2018). Nevertheless, further studies are clearly necessary to provide a more complete understanding of melanocyte responses to red light (Wu et al., 2020). It is evident that melanin itself acts as a photosensitizer, generating 1O2 and other reactive oxidants after red light exposure. The presence of oxidation reactions induced by melanin photosensitization complicates the scenarios of cellular responses after red light exposure, justifying further investigation (Chiarelli-Neto et al., 2011; Chiarelli-Neto et al., 2014).
Red light irradiation also induces strong anti-inflammatory responses in human tissues, suggesting that Langerhans cells also respond to red light. As will be further discussed below, exposure to 650 nm can ameliorate the symptoms of several skin diseases, including psoriasis and atopic dermatitis, by restoring cytokine levels through the reduction of IL-6 and TNF-α (Demirel et al., 2012; Kim et al., 2021). Langerhans cells are integral to innate immune responses, participating in the regulation of T cells and serving as immune sentinels at the skin barrier surface. They sense the environment and regulate immune responses through interactions with microorganisms and viruses (West and Bennett, 2017). According to Salman et al. (2023), red light exposure causes the release of specific cytokines and reduces immune cell migration through the dermis, likely through the activation of Nrf2 (Salman et al., 2023). Indeed, physiological doses of 627 nm have shown to induce the release of IL-4 in co-cultures of keratinocytes and immune cells (macrophages/dendritic cells) (Leong et al., 2018).
Rheumatoid arthritis and osteoarthritis, which involves inflammatory processes that affects the joints, have also been successfully treated by photobiomodulation (Zhang and Qu, 2023). In 2D cell cultures, Sungsoo Na and co-authors have shown enhanced osteoblast proliferation, osteoclast differentiation, and osteoclastic bone resorption activity (Na et al., 2018). By combating the articular degeneration, photobiomodulation has been a key technology to reduce the pain suffered by these patients (Mazulo-Neto et al., 2021).
The sensory mechanisms that allow humans to interact with the environment include the recognition of touch and physical/mechanical stimuli in the skin and in the central nervous system, through neuronal synapses (Talagas et al., 2018). However, there is another non-neuronal cell called the Merkel cell that functions in mechanical and light-touch sensing (Maricich et al., 2009; Bataille et al., 2022). Merkel cells participate in various skin functions such as sensitive indentation, pressure, tactile stimuli, hair movement, and mechanically activated ion channels (Zimmerman et al., 2014; García-Mesa et al., 2017). This type of cell has a significant interaction and is influenced by sun exposure (Horny et al., 2021), making it susceptible to mutations and carcinomas. We did not find any information in the scientific literature that evaluates the responses of Merkel cells to red light irradiation. This could potentially be an interesting topic for future research.
As mentioned above, metabolic activation is also one of the most important hallmarks of the photobiomodulation (Amaroli et al., 2024). Mitochondria activation, including the increase in the rate of oxygen breathing and of ATP production has been observed in many cell types, however, we did not find any report of measurements being done in skin cells. Among others, liver, mesenchymal, neuroblastoma and retina cells showed similar mitochondria activation when irradiated with different light sources (670, 808, 980 nm), (Chu-Tan et al., 2016). Even though skin cells were never directly evaluated, it is very likely they also would respond to the stimulus of red light by increasing the rate of ATP production and the respiratory capacity, since this response has been reported to be similar in different cell types (Mansano, 2020; Amaroli et al., 2021; Tang et al., 2023).
As described above, each type of skin cell has a profile of response to the red light stimuli, which will depend on the particular phenotype and biological function it exerts on the skin. All together, these responses contribute to the systemic tissue changes occurring during and after the red-light exposure, which is currently known as the photobiomodulation effect (Figure 3).
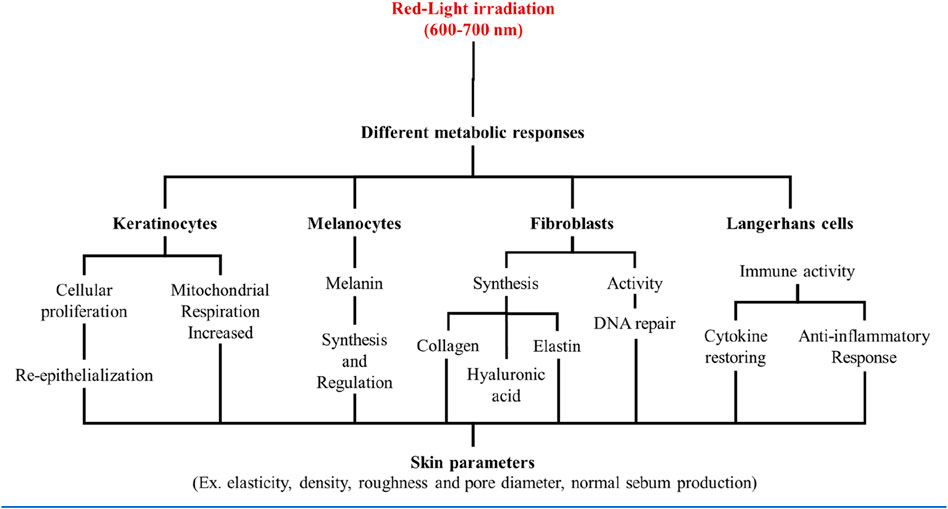
Figure 3. Skin cells were shown to have specific responses to red light stimuli, according to its respective phenotypes. All together those responses contribute to a systemic skin response that is ultimately responsible for the photobiomodulation effect.
Modulation of gene expression mediated by red light exposure
Besides the metabolic changes, exposure to red light can alter expression of several genes and transcription factors on the skin (Da Silva et al., 2023). We will review studies performed mainly with fibroblasts and keratinocytes, aiming to understand the responses of the dermis and epidermis, respectively, during and after the exposure to red light (Figure 4).
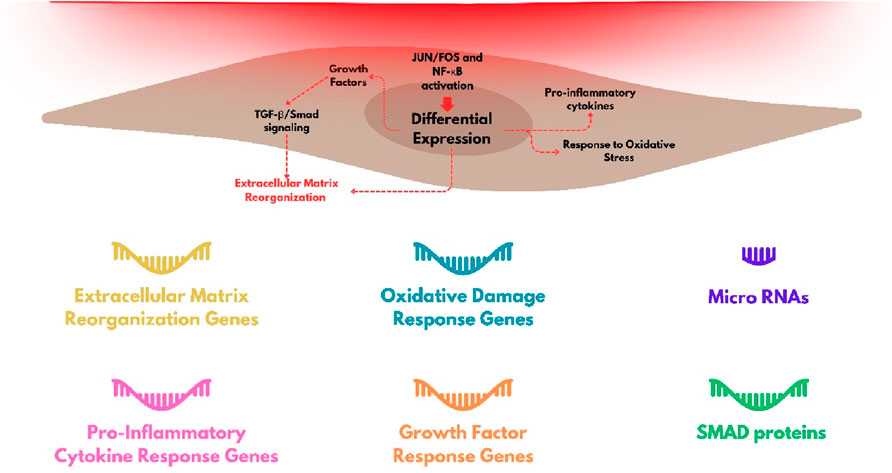
Figure 4. Group of genes with stronger level of modulation by red-light irradiation in fibroblasts and the main cellular processes that are triggered.
Fibroblasts
Fibroblasts are involved in the deposition of proteins in the extracellular space of the dermis, which is fundamental for maintaining the tissue homeostasis. The synthesis of several proteins is activated during wound skin healing, and the dysregulation of this process is critical for the physiopathology of several skin diseases, as for example, in cutaneous fibrosis (Andrews et al., 2016; Darby and Hewitson, 2007). Interestingly, stimulation of wound healing is another hallmark of red light exposure (Felician et al., 2023). The expression of several genes, including of extracellular matrix proteins, such as metalloproteinases (MMP), metalloproteinase inhibitors (TIMP), and collagen isoforms (COL), is regulated by red light exposure (Li et al., 2020). Genes like MMP1, MMP2, MMP3, MMP9, TIMP1, and COL1A1 have shown significantly modulation following irradiation with red light. At a typical dose used in photobiomodulation protocols, i.e. 5 J/cm2 at 660 nm, there is a reduction in the expression of matrix metalloproteinase genes (MMP3 and MMP9), accompanied by an increase in the expression of collagen and proteinase inhibitors, both in normal and challenged fibroblasts (such as those in diabetes or hypoxia) (Ayuk et al., 2018). Kim et al. (2015) observed negative modulation of MMP1 and MMP2 expression and positive modulation of COL1A1 gene expression (although without alteration in the levels of type 1 collagen protein), indicating interference in translation or post-translational mechanisms, following irradiation with 633 nm light. However, the literature shows a lack of consensus on these changes. For example, Lee et al. (2024) observed upregulation of gene expression for both metalloproteinases (MMP1 and MMP3) and type 1 collagen after irradiation with 6 J/cm2 at 628 nm.
Another important set of genes whose expression is affected by red light are those related to the inflammatory response and some growth factors. FGF2 (Fibroblast Growth Factor 2), FGF7, and VEGFA (Vascular endothelial growth factor A) have increased expression in fibroblasts after red light irradiation with 6 J/cm2 at 633 nm (Lee et al., 2024). Heat shock proteins HSPs also have expression levels increased after irradiation with red light (660-nm LED at 30 J/cm2) (Choi et al., 2019). HSPs can also act in responses to other types of stress in addition to thermal, such as oxidative hypoxia, through the folding, remodeling, or degradation of structurally incorrect proteins (Hu et al., 2022).
Genes encoding extracellular matrix remodeling and constitution proteins, growth factors and cytokines (interleukins, angiopoietins, IGFBP, FGF and TGF family), integrins, kinases, mitochondrial and redoxome proteins, are among those upregulated or negatively regulated, depending on the dose of light (Austin et al., 2021; Li et al., 2020; Kim H. S. et al., 2019; Tripodi et al., 2023; Houreld et al., 2014; Zhang et al., 2003).
The differential expression of regulatory RNAs as the microRNAs (miRNAs) involved in the fibrotic process also occurs after red light exposure. Let-7a, miRNA-29, and miRNA-196 showed increased expression levels after irradiation, while pro-fibrotic miRNAs, miRNA-21, miRNA-23b, and miRNA-31, have suppressed expression levels when dermal fibroblasts are exposed to extremely high doses (320 and 640 J/cm2) of red light at 633 nm (Mamalis et al., 2019). Some of these miRNAs are known to regulate growth factor beta TGF pathway, which is directly involved in the fibrotic processes of scleroderma (Zhu et al., 2013; Li et al., 2012).
More comprehensive genomic analyses, by using microarrays or RNA sequencing (RNA-seq), shows the joint expression of groups of genes after red-light irradiation. Evan Austin and colleagues studied the transcriptome of human dermal fibroblasts irradiated by red light 633 nm at a dose of 320 J/cm2 or 640 J/cm2. One of the relevant findings was the temporal resolution of the relative number of Differentially Expressed Genes (DEGs). At time zero after irradiation, 147 of 191 and 205 of 239 DEGs were downregulated and upregulated, respectively. These numbers changed drastically as a function of time, with inversions of the majority regulation modalities (downregulates or upregulates). 4 h after irradiation with 640 J/cm2, the number of positively expressed genes exceeds the number of negatively expressed ones. For cells irradiated at 320 J/cm2, there was equalization in the up and down regulations, along with a significant decrease in DEGs 24 h after irradiation (Austin et al., 2021). These results suggest a transient regulation dependent on both dose and time, complicating the comparisons among studies that have used different irradiation protocols.
In addition to annotating the genes affected by red light exposure, it is important to mention the molecular processes involved in their regulation. The SMAD family of proteins, which belong to the TGF-beta signaling pathway (Derynck et al., 1996; Andrews et al., 2016), includes genes identified in signaling networks through RNA-seq analyses. For instance, SMAD3 (pro-fibrotic) was found to be downregulated from 4 h to 24 h after irradiation with 633 nm light at a dose of 640 J/cm2, accompanied by upregulation of SMAD4 (also pro-fibrotic) and downregulation of SMAD7 (anti-fibrotic). Certain miRNAs identified as mediators of cutaneous fibrosis, as reported by Mamalis et al. (2019), were also found to have downregulated expression under similar irradiation parameters.
The extent of the genes identified also reveals a breadth of cellular pathways and processes involved in the regulation of fibroblasts responses for red-light irradiation. Activation of several transcription factors have been identified, in addition to the SMAD proteins already mentioned, JUN and FOS proteins are also involved in the gene effected by red light (involved in the regulation of MMP synthesis) and also NF-κB families, which are associated with oxidative stress and inflammatory processes (Austin et al., 2021; Kim B. et al., 2019).
Keratinocytes
Although keratinocyte is the most abundant cell found in the skin epidermis, studies of gene expression after exposure to red light, especially at the transcriptional level, are very limited. There are measurements in stressed cells where red-light irradiation predominantly modulated the expression of pro-inflammatory cytokines. For instance, Sun et al. (2018) demonstrated that keratinocytes pre-treated with the pro-oxidant phorbol-12-myristate-13-acetate (PMA) and subsequently irradiated with 633 nm light at doses of 8.1 J/cm2 or 16.2 J/cm2 showed altered gene expression compared to PMA-treated, non-irradiated keratinocytes. They observed differences in the expression of 309 genes between irradiated and non-irradiated cells, including oxidative stress response genes and NF-κB-mediated pro-inflammatory cytokines such as Ptgs2, which encodes cyclooxygenase-2, an enzyme involved in the synthesis of Prostaglandin E2 associated with inflammatory processes. The SPHK1 gene, encoding sphingosine kinase 1, was the most significantly upregulated gene, implicated in suppressing inflammatory effects via NF-κB (Sun et al., 2018). Additionally, Nrf2 activation was confirmed in the same study. Nrf2 activation was also observed in keratinocytes stressed with 2,4-dinitrochlorobenzene (DNCB). Irradiation with 660 nm light at 3 J/cm2 attenuated the expression of pro-inflammatory cytokines (TNF-α, IL-6, and IL-8), except when Nrf2 was silenced using siRNA, indicating that this pathway is crucial for the anti-inflammatory effects of red light on keratinocytes (Salman et al., 2023).
Angiogenesis
The skin, like other organs in the human body, undergoes processes of microcirculation located deep within the dermis, extending to a region close to the basal layer of the epidermis. Skin microcirculation plays a crucial role in regulating thermoregulation, blood pressure, inflammatory responses, and overall tissue homeostasis by facilitating the transport of nutrients and of oxygen and systemic factors through a network extending from arteries to small capillaries near the epidermis (Gutterman et al., 2016; Sanchez et al., 2019). Microvascular endothelial cells are the primary components of dermal blood vessels and contribute to the synthesis and secretion of chemokines and cytokines, recruiting immune cells in response to various stimuli such as light, heat, and both pathological and physiological processes (Swerlick and Lawley, 1993).
RNA-seq analysis in a human umbilical vein endothelial cell showed that cells irradiated at 632.8 nm with a dose of 1 J/cm2 revealed 103 downregulated genes and 135 upregulated genes. KEGG pathway enrichment analysis showed correlations with the TGF-beta, TNF-beta, HIF-1, and insulin signaling pathways, as well as cytokine-cytokine receptor interactions. The vascular endothelial growth factor (VEGF) pathway, which is crucial for angiogenesis and wound healing, was significantly activated as demonstrated by Zhang et al. (2022), along with increased cellular migration after irradiation.
S-nitrosothiol modulation by red light and the mechanism of photobiomodulation: implications for the treatment of psoriasis
Another hallmark of red light exposure is the modulation of cytokines and of inflammatory processes. In a murine model, red light exposure has been proven to decrease the expression of inflammatory cytokines and to increase the levels of anti-inflammatory cytokines (Shamloo et al., 2023; Costa et al., 2017). From a redox point of view, anti-inflammatory responses can be correlated with the redox homeostasis of the skin and with the upregulation of its antioxidant mechanisms (Sun et al., 2018; Simplicio et al., 2009; Shamloo et al., 2023; Schalka et al., 2022; De Freitas and Hamblin, 2016; García-Criado et al., 2009; Dompe et al., 2020). The ability of nitric oxide (NO) to inhibit the progression of the lipid peroxidation reactions is likely to play an important role in this context (Simplicio et al., 2009; Hogg and Kalyanaraman, 1999; Suschek et al., 2001). There is also evidence that NO donors, i.e. small molecule nitrosothiols, reduce the production of inflammatory factors. (García-Criado et al., 2009; Wang M et al., 2021; Corti et al., 2014).
Thiols, are sulfur-based compounds that play an important role on cell signaling mechanisms and on the redox homeostasis of the skin (Ulrich and Jakob, 2019). They are surely among the most important compounds in the antioxidant defenses of cells and tissues (Georgescu et al., 2022). There is an equilibrium between thiols and their oxidized forms, disulfides, known as dynamic thiol-disulfide homeostasis (TDH). There are well stablished correlations between TDH dysregulation and skin inflammatory diseases such as psoriasis (Georgescu et al., 2022). Cysteine thiols and their oxidized disulfide counterparts are carefully balanced to maintain redox homeostasis in various cellular compartments, protect organisms from oxidative stressors, and actively participate in redox regulation and signaling processes. (Ulrich and Jakob, 2019).
When associated with NO, thiols form s-nitrosothiols (RSNO) (Marozkina and Gaston, 2020). NO is a weak oxidant that rarely reacts spontaneously with thiol residues in the physiological environment. Cysteine residues have a pKa of 8.3, indicating that a small percentage of the cysteine exist in the form of thiolate ion (S-) (Massa et al., 2021). However, NO oxidation products are capable of reacting with free thiols (Wink et al., 1996; Massa et al., 2021). Consequently, the formation of RSNO occurs mainly after oxidation reactions that transform NO in nitrogen oxides, such as nitrogen dioxide (NO2) and nitrogen trioxide (N2O3) (Shi and Qiu, 2020; Jourd’heuil et al., 2003). Also, red light, peaking at 700 nm, can strongly induce the formation of nitrosothiols (Pelegrino et al., 2020). By logic analysis, it is evident that red light photons should be able to favor the oxidation of NO and the formation of NO2 and N2O3, although this was not yet shown experimentally.
Besides forming NO stocks, there is clear experimental evidence that visible light is capable of decomposing RSNO, promoting the release of NO (Weihrauch et al., 2021; Opländer et al., 2013; Barolet et al., 2021). Indeed, exposing RSNOs to sun light causes RSNOs decomposition and NO release (Veleeparampil et al., 2009). It is therefore evident that sun light exposure is an extraordinary effective way to naturally release NO in the skin (Veleeparampil et al., 2009; Weihrauch D et al., 2021; Opländer et al., 2013; Barolet et al., 2021).
Red light radiation carries ∼43 kcal per mol of photons, which is an energy capable of decomposing RSNO (Barolet et al., 2021). In this context, light causes the homolytic cleavage of sulfur-nitrogen bonds and release NO from nitrosothiol derivatives (Singh et al., 1996). It is evident therefore that typical protocols of photobiomodulation can induce the formation of novel NO stocks, as well as the release of bioactive NO, which may explain several of the immunomodulatory and redox homeostatic roles played by red light and irradiation during photobiomodulation protocols (Hamblin, 2017; Shamloo et al., 2023; Couturaud et al., 2023).
Psoriasis is a chronic inflammatory disease highly correlated with an increased expression level of inducible nitric oxide synthase (NOS2), an enzyme that can increase the intracellular NO levels by several orders of magnitude, causing oxidation distress and the release of pro-inflammatory cytokines (Wong et al., 2013; Bruch-Gerharz et al., 1996; Pujari et al., 2014). High levels of nitrite, nitrate and malonyldialdehyde (MDA) in psoriasis patients is also an important biomarker of this disease (Tekin et al., 2006; Cannavò et al., 2019). Both oxidative stress and inflammatory cytokines are correlated with increased intracellular levels of nitric oxide (NO) (Suschek et al., 2001; Lee et al., 2000; Demirel et al., 2012; Raykova et al., 2003). In the condition of oxidative distress present in psoriasis, the increased NOS2 activity is paired with the decrease in the levels of superoxide dismutase, favoring the formation of peroxynitrite (Yildirim et al., 2003; Massa et al., 2021). The use of NO-releasing creams for the treatment of disease is proposed, which suggest that low NO bioavailability (Matoshvili et al., 2014), associated with oxidative stress is an important factor for the development of the pathology.
Several literature reports suggest that sun exposure has positive effects on improving chronic inflammatory skin diseases, such as psoriasis (Berg, 1989; Søyland et al., 2011; Matoshvili et al., 2014). Therefore, it has become evident that psoriasis can be treated by photomedicine (Makuch et al., 2022; Hamblin et al., 2023; Kleinpenning et al., 2012). Two protocols have been used: (i) use of psoralen and skin irradiation with UVA (PUVA), and (ii) UVB phototherapy (Wong et al., 2013; Foerster et al., 2017; Tahir and Mujtaba, 2004). There is also evidence that the mechanisms by which these photomedicine protocols work are somehow correlated with NO homeostasis. UV photons can modulate NO bioavailability, via photodecomposition of intracellular NO stocks (Barolet et al., 2021; Opländer et al., 2013), as well as by inhibition of NOS2 activity (Johnson-Huang et al., 2010), suggesting a possible temporary reestablishment of the NO bioavailability homeostasis. Although effective, both treatments use high-energy photons that induce many side effects (Informed Health, 2021; Chuang et al., 1992; Osmancevic et al., 2014; Thatiparthi et al., 2022). Possible side effects of UV radiation therapy include dry skin, itching, as well as skin reactions similar to sunburn (Informed Health, 2021). PUVA therapy has short- and long-term adverse effects. Such erythema, pruritus, nausea and headache, and in the long term, the development of chronic actinic skin damage and dyskeratotic skin conditions (van Praag et al., 1993) Furthermore, the correlation between the use of UV radiation and the occurrence of skin cancer, although controversial, is also described in the literature (Chuang et al., 1992; Osmancevic et al., 2014; Thatiparthi et al., 2022). In this context, it is necessary to advance to new strategies that boost the bioavailability of skin NO, and to reduce inflammation without causing severe adverse side effects.
Strategies that aim to increase thiol levels in the skin can act as a starting point for the generation of RSNOs, when associated with the presence of red light. The logical photobiomodulation strategy follows the use of topical thiols associated with exposure to red light. The exposure time in order to red light induce RSNO generation in the skin is unclear, but it is suggested that long exposure times are necessary (Pelegrino et al., 2020). On the other hand, after the photobiomodulation process, RSNO can be decomposed via exposure to natural sunlight in the affected areas (Veleeparampil et al., 2009), with the corresponding increase in the amount of free NO (Figure 5). Increase in the bioavailability of NO in psoriatic lesions could work in a similar manner to the NO-releasing creams, which are already being suggest for this treatment (Matoshvili et al., 2014). In addition to being a low-cost treatment, red light irradiation also has no side effects to the skin when compared to UV light.
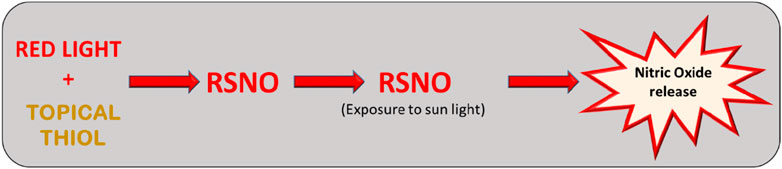
Figure 5. The use of topical thiols associated with exposure to red light could increase the levels of RSNOs in the skin and possibly modulate the inflammatory process. RSNO can be decomposed via exposure to natural sunlight in the psoriasis affected areas with the corresponding increase in the amount of free NO in the tissue.
Conclusion
Understanding the interactions of red-light photons with the human skin is an important step towards the development of novel photonic technologies. In here, we described how red-light photons interact with skin molecules, as well as the subsequent skin responses at the molecular and cellular levels, with direct implications to the mechanisms involved in photobiomodulation. It is becoming more evident that controlled sun exposure is beneficial to the lifespan and to the life quality of individuals and the exposure to the red-light photons may explain part of the benefits. We also highlighted several aspects that need further research to be properly explained. We hope this review will stimulate further basic and applied research in this field to allow the development of novel and optimized photonic techniques applied to photomedicine.
Author contributions
MH: Conceptualization, Validation, Visualization, Writing–original draft, Writing–review and editing. AR: Conceptualization, Formal Analysis, Visualization, Writing–original draft, Writing–review and editing. PC: Conceptualization, Formal Analysis, Supervision, Visualization, Writing–original draft, Writing–review and editing. MB: Conceptualization, Project administration, Resources, Supervision, Writing–original draft, Writing–review and editing.
Funding
The author(s) declare financial support was received for the research, authorship, and/or publication of this article. Grant: # 2022/13066-9, 2021/08521-6 and 2013/07937-8, Fundação de Amparo à Pesquisa do Estado de São Paulo (FAPESP). 88887.951897/2024-00, Capes.
Conflict of interest
The authors declare that the research was conducted in the absence of any commercial or financial relationships that could be construed as a potential conflict of interest.
Publisher’s note
All claims expressed in this article are solely those of the authors and do not necessarily represent those of their affiliated organizations, or those of the publisher, the editors and the reviewers. Any product that may be evaluated in this article, or claim that may be made by its manufacturer, is not guaranteed or endorsed by the publisher.
References
Abe, Y., Konno, H., Yoshida, S., Yamauchi, T., Yamasaki, K., Denda, M., et al. (2019). Red light-promoted skin barrier recovery: spatiotemporal evaluation by transepidermal potential. PLoS One 14 (7), e0219198. doi:10.1371/journal.pone.0219198
Abergel, R. P., Lyons, R. F., Castel, J. C., Dwyer, R. M., and Uitto, J. (1987). Biostimulation of wound healing by lasers: experimental approaches in animal models and in fibroblast cultures. J. Dermatol Surg. Oncol. 13 (2), 127–133. doi:10.1111/j.1524-4725.1987.tb00510.x
Ahmad, A., Dempsey, S. K., Daneva, Z., Azam, M., Li, N., Li, P. L., et al. (2018). Role of nitric oxide in the cardiovascular and renal systems. Int. J. Mol. Sci. 19 (9), 2605. doi:10.3390/ijms19092605
Amaroli, A., Arany, P., Pasquale, C., Benedicenti, S., Bosco, A., and Ravera, S. (2021). Improving consistency of photobiomodulation therapy: a novel flat-top beam hand-piece versus standard gaussian probes on mitochondrial activity. Int. J. Mol. Sci. 22 (15), 7788. doi:10.3390/ijms22157788
Amaroli, A., Clemente Vargas, M. R., Pasquale, C., Raffetto, M., and Ravera, S. (2024). Photobiomodulation on isolated mitochondria at 810 nm: first results on the efficiency of the energy conversion process. Sci. Rep. 14 (1), 11060. doi:10.1038/s41598-024-61740-w
Andrews, J. P., Marttala, J., Macarak, E., Rosenbloom, J., and Uitto, J. (2016). Keloids: the paradigm of skin fibrosis - pathomechanisms and treatment. Matrix Biol. 51, 37–46. doi:10.1016/j.matbio.2016.01.013
Austin, E., Koo, E., Merleev, A., Torre, D., Marusina, A., Luxardi, G., et al. (2021). Transcriptome analysis of human dermal fibroblasts following red light phototherapy. Sci. Rep. 11 (1), 7315. doi:10.1038/s41598-021-86623-2
Ayuk, S. M., Abrahamse, H., and Houreld, N. N. (2018). Photobiomodulation alters matrix protein activity in stressed fibroblast cells in vitro. J. Biophot. 11 (3). doi:10.1002/jbio.201700127
Baptista, M. S., Cadet, J., Greer, A., and Thomas, A. H. (2021). Photosensitization reactions of biomolecules: definition, targets and mechanisms. Photochem Photobiol. 97 (6), 1456–1483. doi:10.1111/php.13470
Barolet, A. C., Litvinov, I. V., and Barolet, D. (2021). Light-induced nitric oxide release in the skin beyond UVA and blue light: red and near-infrared wavelengths. Nitric Oxide 117, 16–25. doi:10.1016/j.niox.2021.09.003
Bastos, E. L., Quina, F. H., and Baptista, M. S. (2023). Endogenous photosensitizers in human skin. Chem. Rev. 123 (16), 9720–9785. doi:10.1021/acs.chemrev.2c00787
Bataille, A., Le Gall, C., Misery, L., and Talagas, M. (2022). Merkel cells are multimodal sensory cells: a review of study methods. Cells 11 (23), 3827. doi:10.3390/cells11233827
Berg, M. (1989). Epidemiological studies of the influence of sunlight on the skin. Photodermatol 6 (2), 80–84.
Brondon, P., Stadler, I., and Lanzafame, R. J. (2009). Pulsing influences photoradiation outcomes in cell culture. Lasers Surg. Med. 41 (3), 222–226. doi:10.1002/lsm.20740
Bruch-Gerharz, D., Fehsel, K., Suschek, C., Michel, G., Ruzicka, T., and Kolb-Bachofen, V. (1996). A proinflammatory activity of interleukin 8 in human skin: expression of the inducible nitric oxide synthase in psoriatic lesions and cultured keratinocytes. J. Exp. Med. 184 (5), 2007–2012. doi:10.1084/jem.184.5.2007
Cannavò, S. P., Riso, G., Casciaro, M., Di Salvo, E., and Gangemi, S. (2019). Oxidative stress involvement in psoriasis: a systematic review. Free Radic. Res. 53 (8), 829–840. doi:10.1080/10715762.2019.1648800
Chen, L., Xu, Z., Jiang, M., Zhang, C., Wang, X., and Xiang, L. (2018). Light-emitting diode 585nm photomodulation inhibiting melanin synthesis and inducing autophagy in human melanocytes. J. Dermatol Sci. 89 (1), 11–18. doi:10.1016/j.jdermsci.2017.10.001
Chiarelli-Neto, O., Ferreira, A. S., Martins, W. K., Pavani, C., Severino, D., Faião-Flores, F., et al. (2014). Melanin photosensitization and the effect of visible light on epithelial cells. PLoS One 9 (11), e113266. doi:10.1371/journal.pone.0113266
Chiarelli-Neto, O., Pavani, C., Ferreira, A. S., Uchoa, A. F., Severino, D., and Baptista, M. S. (2011). Generation and suppression of singlet oxygen in hair by photosensitization of melanin. Free Radic. Biol. Med. 51 (6), 1195–1202. doi:10.1016/j.freeradbiomed.2011.06.013
Choi, S. H., Chang, S. Y., Biswas, R., Chung, P. S., Mo, S., Lee, M. Y., et al. (2019). Light-emitting diode irradiation using 660 nm promotes human fibroblast HSP90 expression and changes cellular activity and morphology. J. Biophot. 12 (9), e201900063. doi:10.1002/jbio.201900063
Chuang, T. Y., Heinrich, L. A., Schultz, M. D., Reizner, G. T., Kumm, R. C., and Cripps, D. J. (1992). PUVA and skin cancer. J. Am. Acad. Dermatol 26 (2 Pt 1), 173–177. doi:10.1016/0190-9622(92)70021-7
Chu-Tan, J. A., Rutar, M., Saxena, K., Wu, Y., Howitt, L., Valter, K., et al. (2016). Efficacy of 670 nm light therapy to protect against photoreceptor cell death is dependent on the severity of damage. Intern. J. of Photoenerg., 1–12. doi:10.1155/2016/2734139
Corti, A., Franzini, M., Scataglini, I., and Pompella, A. (2014). Mechanisms and targets of the modulatory action of S-nitrosoglutathione (GSNO) on inflammatory cytokines expression. Arch. Biochem. Biophys. 562, 80–91. doi:10.1016/j.abb.2014.08.002
Costa, S. G., Barioni É, D., Ignácio, A., Albuquerque, J., Câmara, N. O. S., Pavani, C., et al. (2017). Beneficial effects of Red Light-Emitting Diode treatment in experimental model of acute lung injury induced by sepsis. Sci. Rep. 7 (1), 12670. doi:10.1038/s41598-017-13117-5
Couturaud, V., Le Fur, M., Pelletier, M., and Granotier, F. (2023). Reverse skin aging signs by red light photobiomodulation. Skin. Res. Technol. 29 (7), e13391. doi:10.1111/srt.13391
Darby, I. A., and Hewitson, T. D. (2007). Fibroblast differentiation in wound healing and fibrosis. Int. Rev. Cytol. 257, 143–179. doi:10.1016/s0074-7696(07)57004-x
Da Silva, T. G., Ribeiro, R. S., Mencalha, A. L., and de Souza Fonseca, A. (2023). Photobiomodulation at molecular, cellular, and systemic levels. Lasers Med. Sci. 38 (1), 136. doi:10.1007/s10103-023-03801-6
De Assis, L. V. M., Tonolli, P. N., Moraes, M. N., Baptista, M. S., and de Lauro Castrucci, A. M. (2021). How does the skin sense sun light? An integrative view of light sensing molecules. J. Photochem. Photobiol. C Photochem. Rev. 47, 100403. doi:10.1016/j.jphotochemrev.2021.100403
De Freitas, L. F., and Hamblin, M. R. (2016). Proposed mechanisms of photobiomodulation or low-level light therapy. IEEE J. Sel. Top. Quantum Electron 22 (3), 348–364. doi:10.1109/JSTQE.2016.2561201
Demirel, I., Vumma, R., Mohlin, C., Svensson, L., Säve, S., and Persson, K. (2012). Nitric oxide activates IL-6 production and expression in human renal epithelial cells. Am. J. Nephrol. 36 (6), 524–530. doi:10.1159/000345351
Derynck, R., Gelbart, W. M., Harland, R. M., Heldin, C.-H., Kern, S. E., Massagué, J., et al. (1996). Nomenclature: vertebrate mediators of TGFβ family signals. Cell 87 (2), 173. doi:10.1016/S0092-8674(00)81335-5
Dompe, C., Moncrieff, L., Matys, J., Grzech-Leśniak, K., Kocherova, I., Bryja, A., et al. (2020). Photobiomodulation-Underlying mechanism and clinical applications. J. Clin. Med. 9 (6), 1724. doi:10.3390/jcm9061724
Felician, M. C. P., Belotto, R., Tardivo, J. P., Baptista, M. S., and Martins, W. K. (2023). Photobiomodulation: cellular, molecular, and clinical aspects. J. Photochem. Photobiol. 17, 100197. doi:10.1016/j.jpap.2023.100197
Foerster, J., Boswell, K., West, J., Cameron, H., Fleming, C., Ibbotson, S., et al. (2017). Narrowband UVB treatment is highly effective and causes a strong reduction in the use of steroid and other creams in psoriasis patients in clinical practice. PLoS One 12 (8), e0181813. doi:10.1371/journal.pone.0181813
Gambino, G., Gallo, D., Covelo, A., Ferraro, G., Sardo, P., and Giglia, G. (2022). TRPV1 channels in nitric oxide-mediated signalling: insight on excitatory transmission in rat CA1 pyramidal neurons. Free Radic. Biol. Med. 191, 128–136. doi:10.1016/j.freeradbiomed.2022.08.025
García-Criado, F. J., Rodriguez-Barca, P., García-Cenador, M. B., Rivas-Elena, J. V., Grande, M. T., Lopez-Marcos, J. F., et al. (2009). Protective effect of new nitrosothiols on the early inflammatory response to kidney ischemia/reperfusion and transplantation in rats. J. Interferon Cytokine Res. 29 (8), 441–450. doi:10.1089/jir.2008.0100
García-Mesa, Y., García-Piqueras, J., García, B., Feito, J., Cabo, R., Cobo, J., et al. (2017). Merkel cells and Meissner's corpuscles in human digital skin display Piezo2 immunoreactivity. J. Anat. 231 (6), 978–989. doi:10.1111/joa.12688
Gebremendhin, D., Lindemer, B., Weihrauch, D., Harder, D. R., and Lohr, N. L. (2021). Electromagnetic energy (670 nm) stimulates vasodilation through activation of the large conductance potassium channel (BKCa). PLoS One 16 (10), e0257896. doi:10.1371/journal.pone.0257896
George, S., Hamblin, M., and Abrahamse, H. (2018). Effect of red light and near infrared laser on the generation of reactive oxygen species in primary dermal fibroblasts. J. Photochem. Photobiol. B Biol. 188, 60–68. doi:10.1016/j.jphotobiol.2018.09.004
Georgescu, S. R., Mitran, C. I., Mitran, M. I., Matei, C., Popa, G. L., Erel, O., et al. (2022). Thiol-disulfide homeostasis in skin diseases. J. Clin. Med. 11 (6), 1507. doi:10.3390/jcm11061507
Glass, G. E. (2021). Photobiomodulation: a review of the molecular evidence for low level light therapy. J. Plast. Reconstr. Aesthet. Surg. 74 (5), 1050–1060. doi:10.1016/j.bjps.2020.12.059
Gutterman, D. D., Chabowski, D. S., Kadlec, A. O., Durand, M. J., Freed, J. K., Ait-Aissa, K., et al. (2016). The human microcirculation: regulation of flow and beyond. Circ. Res. 118, 157–172. doi:10.1161/CIRCRESAHA.115.305364
Hamblin, M. R. (2017). Mechanisms and applications of the anti-inflammatory effects of photobiomodulation. AIMS Biophys. 4 (3), 337–361. doi:10.3934/biophy.2017.3.337
Hamblin, M. R., Wen, X., and Zhou, B. (2023). Editorial: photobiomodulation and phototherapy in skin diseases. Front. Med. (Lausanne) 10, 1357286. doi:10.3389/fmed.2023.1357286
Hogg, N., and Kalyanaraman, B. (1999). Nitric oxide and lipid peroxidation. Biochim. Biophys. Acta 1411 (2-3), 378–384. doi:10.1016/s0005-2728(99)00027-4
Horny, K., Gerhardt, P., Hebel-Cherouny, A., Wülbeck, C., Utikal, J., and Becker, J. C. (2021). Mutational landscape of virus- and UV-associated Merkel cell carcinoma cell lines is comparable to tumor tissue. Cancers (Basel) 13 (4), 649. doi:10.3390/cancers13040649
Houreld, N. N., Ayuk, S. M., and Abrahamse, H. (2014). Expression of genes in normal fibroblast cells (WS1) in response to irradiation at 660nm. J. Photochem Photobiol. B 130, 146–152. doi:10.1016/j.jphotobiol.2013.11.018
Hu, C., Yang, J., Qi, Z., Wu, H., Wang, B., Zou, F., et al. (2022). Heat shock proteins: biological functions, pathological roles, and therapeutic opportunities. MedComm (2020) 3 (3), e161. doi:10.1002/mco2.161
Informed Health (2021). Psoriasis: learn More – does light therapy (phototherapy) help reduce psoriasis symptoms? Cologne, Germany: Institute for Quality and Efficiency in Health Care.
Johnson-Huang, L. M., Suárez-Fariñas, M., Sullivan-Whalen, M., Gilleaudeau, P., Krueger, J. G., and Lowes, M. A. (2010). Effective narrow-band UVB radiation therapy suppresses the IL-23/IL-17 axis in normalized psoriasis plaques. J. Invest. Dermatol 130 (11), 2654–2663. doi:10.1038/jid.2010.166
Jourd’heuil, D., Jourd’heuil, F. L., and Feelisch, M. (2003). Oxidation and nitrosation of thiols at low micromolar exposure to nitric oxide. J. Biol. Chem. 278 (18), 15720–15726. doi:10.1074/jbc.M300203200
Kim, B., Mukherjee, A., Seo, S., Ali, F., Southall, M., and Parsa, R. (2019a). Low-level red and infrared light increases expression of collagen, elastin, and hyaluronic acid in skin. J. Am. Acad. Dermatology 81 (4), AB434. doi:10.1016/j.jaad.2019.10.089
Kim, H. S., Kim, Y. J., Kim, S. J., Kang, D. S., Lee, T. R., Shin, D. W., et al. (2019b). Transcriptomic analysis of human dermal fibroblast cells reveals potential mechanisms underlying the protective effects of visible red light against damage from ultraviolet B light. J. Dermatol Sci. 94 (2), 276–283. doi:10.1016/j.jdermsci.2019.03.003
Kim, H. S., Park, W. S., Baek, J. I., Lee, B. S., Yoo, D. S., and Park, S. J. (2015). Continuous irradiation with a 633-nm light-emitting diode exerts an anti-aging effect on human skin cells. Int. J. Mol. Med. 35 (2), 383–390. doi:10.3892/ijmm.2014.2030
Kim, S. K., You, H. R., Kim, S. H., Yun, S. J., Lee, S. C., and Lee, J. B. (2016). Skin photorejuvenation effects of light-emitting diodes (LEDs): a comparative study of yellow and red LEDs in vitro and in vivo. Clin. Exp. Dermatol 41 (7), 798–805. doi:10.1111/ced.12902
Kim, Y. J., Kim, H.-J., Kim, H. L., Kim, H. J., Kim, H. S., Lee, T. R., et al. (2017). A protective mechanism of visible red light in normal human dermal fibroblasts: enhancement of gadd45a-mediated DNA repair activity. J. Investigative Dermatology 137 (2), 466–474. doi:10.1016/j.jid.2016.07.041
Kim, Y. L., Lim, H. S., and Lee, S. M. (2021). Effect of low-level laser intervention on dermatitis symptoms and cytokine changes in DNCB-induced atopy mouse model: a randomized controlled trial. Exp. Ther. Med. 22 (5), 1196. doi:10.3892/etm.2021.10630
Kleinpenning, M. M., Otero, M. E., van Erp, P. E., Gerritsen, M. J., and van de Kerkhof, P. C. (2012). Efficacy of blue light vs. red light in the treatment of psoriasis: a double-blind, randomized comparative study. J. Eur. Acad. Dermatol Venereol. 26 (2), 219–225. doi:10.1111/j.1468-3083.2011.04039.x
Laffranchi, R., Gogvadze, V., Richter, C., and Spinas, G. A. (1995). Nitric oxide (nitrogen monoxide, NO) stimulates insulin secretion by inducing calcium release from mitochondria. Biochem. Biophys. Res. Commun. 217 (2), 584–591. doi:10.1006/bbrc.1995.2815
Lee, S. C., Lee, J. W., Jung, J. E., Lee, H. W., Chun, S. D., Kang, I. K., et al. (2000). Protective role of nitric oxide-mediated inflammatory response against lipid peroxidation in ultraviolet B-irradiated skin. Br. J. Dermatol 142 (4), 653–659. doi:10.1046/j.1365-2133.2000.03409.x
Lee, Y. I., Lee, S. G., Ham, S., Jung, I., Suk, J., and Lee, J. H. (2024). Exploring the safety and efficacy of organic light-emitting diode in skin rejuvenation and wound healing. Yonsei Med. J. 65 (2), 98–107. doi:10.3349/ymj.2023.0125
Leong, C., Bigliardi, P. L., Sriram, G., Au, V. B., Connolly, J., and Bigliardi-Qi, M. (2018). Physiological doses of red light induce IL-4 release in cocultures between human keratinocytes and immune cells. Photochem Photobiol. 94 (1), 150–157. doi:10.1111/php.12817
Li, H., Yang, R., Fan, X., Gu, T., Zhao, Z., Chang, D., et al. (2012). MicroRNA array analysis of microRNAs related to systemic scleroderma. Rheumatol. Int. 32 (2), 307–313. doi:10.1007/s00296-010-1615-y
Li, W., Hu, X., Lu, X., Liu, J., Chen, Z., Zhou, X., et al. (2020). RNA-Seq analysis revealed the molecular mechanisms of photobiomodulation effect on human fibroblasts. Photodermatol. Photoimmunol. Photomed. 36 (4), 299–307. doi:10.1111/phpp.12554
Lima, P. L. V., Pereira, C. V., Nissankan, N., Arguello, T., Gavini, G., Maranduba, C. M. C., et al. (2019). Photobiomodulation enhancement of cell proliferation at 660 nm does not require cytochrome c oxidase. J. Photochem Photobiol. B 194, 71–75. doi:10.1016/j.jphotobiol.2019.03.015
Macedo, A. C. B., Oliveira, C. F. d., Silva, E. C. M., and Andrade, L. A. S. d. (2012). Efeitos da aplicação do L.A.S.E.R. HeNe e do ultravioleta B no vitiligo. Fisioter. Mov. 25, 481–488. doi:10.1590/s0103-51502012000300003
Makuch, S., Dróżdż, M., Makarec, A., Ziółkowski, P., and Woźniak, M. (2022). An update on photodynamic therapy of psoriasis-current strategies and nanotechnology as a future perspective. Int. J. Mol. Sci. 23 (17), 9845. doi:10.3390/ijms23179845
Mamalis, A., Koo, E., Tepper, C., and Jagdeo, J. (2019). MicroRNA expression analysis of human skin fibroblasts treated with high-fluence light-emitting diode-red light. J. Biophot. 12 (5), e201800207. doi:10.1002/jbio.201800207
Mansano, B. S. D. M. (2020). “Red LEDs to improve the efficiency of mesenchymal stem cell therapy,”. PhD (São Paulo, Brazil: Universidade Nove de Julho).
Maricich, S. M., Wellnitz, S. A., Nelson, A. M., Lesniak, D. R., Gerling, G. J., Lumpkin, E. A., et al. (2009). Merkel cells are essential for light-touch responses. Science 324 (5934), 1580–1582. doi:10.1126/science.1172890
Marozkina, N., and Gaston, B. (2020). An update on thiol signaling: S-nitrosothiols, hydrogen sulfide and a putative role for thionitrous acid. Antioxidants (Basel) 9 (3), 225. doi:10.3390/antiox9030225
Massa, C. M., Liu, Z., Taylor, S., Pettit, A. P., Stakheyeva, M. N., Korotkova, E., et al. (2021). Biological mechanisms of S-nitrosothiol formation and degradation: how is specificity of S-nitrosylation achieved? Antioxidants (Basel) 10 (7), 1111. doi:10.3390/antiox10071111
Matoshvili, M., Katsitadze, A., Sanikidze, T., Tophuria, D., Richetta, A., and D'Epiro, S. (2014). The role of nitric oxide in the pathogenesis and severity of psoriasis. Georgian Med. News 234, 61–64.
Mazulo-Neto, J. C. R., Souza, L. G., Mazulo, F. R. S., and Filgueiras, M. d. C. (2021). Effects of photobiomodulation in pain and articular degeneration in mice arthritis model. Braz. J. Pain 4, 104–107. doi:10.5935/2595-0118.20210028
McGarr, G. W., King, K. E., Cassan, C. J. M., Janetos, K. T., Fujii, N., and Kenny, G. P. (2023). Involvement of nitric oxide synthase and reactive oxygen species in TRPA1-mediated cutaneous vasodilation in young and older adults. Microvasc. Res. 145, 104443. doi:10.1016/j.mvr.2022.104443
Na, S., TruongVo, T., Jiang, F., Joll, J. E., Guo, Y., Utreja, A., et al. (2018). Dose analysis of photobiomodulation therapy on osteoblast, osteoclast, and osteocyte. J. Biomed. Opt. 23 (7), 1–8. doi:10.1117/1.jbo.23.7.075008
Oh, C. T., Kwon, T. R., Choi, E. J., Kim, S. R., Seok, J., Mun, S. K., et al. (2017). Inhibitory effect of 660-nm LED on melanin synthesis in in vitro and in vivo. Photodermatol. Photoimmunol. Photomed. 33 (1), 49–57. doi:10.1111/phpp.12276
Opländer, C., Deck, A., Volkmar, C. M., Kirsch, M., Liebmann, J., Born, M., et al. (2013). Mechanism and biological relevance of blue-light (420-453 nm)-induced nonenzymatic nitric oxide generation from photolabile nitric oxide derivates in human skin in vitro and in vivo. Free Radic. Biol. Med. 65, 1363–1377. doi:10.1016/j.freeradbiomed.2013.09.022
Osmancevic, A., Gillstedt, M., Wennberg, A. M., and Larkö, O. (2014). The risk of skin cancer in psoriasis patients treated with UVB therapy. Acta Derm. Venereol. 94 (4), 425–430. doi:10.2340/00015555-1753
Pelegrino, M. T., Paganotti, A., Seabra, A. B., and Weller, R. B. (2020). Photochemistry of nitric oxide and S-nitrosothiols in human skin. Histochem Cell Biol. 153 (6), 431–441. doi:10.1007/s00418-020-01858-w
Pujari, V. M., Ireddy, S., Itagi, I., and Kumar, H. S. (2014). The serum levels of malondialdehyde, vitamin e and erythrocyte catalase activity in psoriasis patients. J. Clin. Diagn Res. 8 (11), CC14–6. doi:10.7860/JCDR/2014/10912.5085
Raykova, V. D., Glibetic, M., Ofenstein, J. P., and Aranda, J. V. (2003). Nitric oxide-dependent regulation of pro-inflammatory cytokines in group B streptococcal inflammation of rat lung. Ann. Clin. Lab. Sci. 33 (1), 62–67.
Salman, S., Guermonprez, C., Peno-Mazzarino, L., Lati, E., Rousseaud, A., Declercq, L., et al. (2023). Photobiomodulation controls keratinocytes inflammatory response through Nrf2 and reduces Langerhans cells activation. Antioxidants (Basel) 12 (3), 766. doi:10.3390/antiox12030766
Sanchez, B., Li, L., Dulong, J., Aimond, G., Lamartine, J., Liu, G., et al. (2019). Impact of human dermal microvascular endothelial cells on primary dermal fibroblasts in response to inflammatory stress. Front. Cell Dev. Biol. 7, 44. doi:10.3389/fcell.2019.00044
Schalka, S., Silva, M. S., Lopes, L. F., de Freitas, L. M., and Baptista, M. S. (2022). The skin redoxome. J. Eur. Acad. Dermatol Venereol. 36 (2), 181–195. doi:10.1111/jdv.17780
Sena, L. A., and Chandel, N. S. (2012). Physiological roles of mitochondrial reactive oxygen species. Mol. Cell 48 (2), 158–167. doi:10.1016/j.molcel.2012.09.025
Shamloo, S., Defensor, E., Ciari, P., Ogawa, G., Vidano, L., Lin, J. S., et al. (2023). The anti-inflammatory effects of photobiomodulation are mediated by cytokines: evidence from a mouse model of inflammation. Front. Neurosci. 17, 1150156. doi:10.3389/fnins.2023.1150156
Shi, X., and Qiu, H. (2020). Post-translational S-nitrosylation of proteins in regulating cardiac oxidative stress. Antioxidants (Basel) 9 (11), 1051. doi:10.3390/antiox9111051
Sies, H., and Jones, D. P. (2020). Reactive oxygen species (ROS) as pleiotropic physiological signalling agents. Nat. Rev. Mol. Cell Biol. 21 (7), 363–383. doi:10.1038/s41580-020-0230-3
Simões, T. M. S., Fernandes Neto, J. A., de Oliveira, T. K. B., Nonaka, C. F. W., and Catão, M. (2020). Photobiomodulation of red and green lights in the repair process of third-degree skin burns. Lasers Med. Sci. 35 (1), 51–61. doi:10.1007/s10103-019-02776-7
Simplicio, F. I., Seabra, A. B., de Souza, G. F. P., and de Oliveira, M. G. (2009). In vitro inhibition of linoleic acid peroxidation by primary S-nitrosothiols. J. Braz. Chem. Soc. 21 (10), 1885–1895. doi:10.1590/S0103-50532010001000013
Singh, R. J., Hogg, N., Joseph, J., and Kalyanaraman, B. (1996). Mechanism of nitric oxide release from S-nitrosothiols. J. Biol. Chem. 271 (31), 18596–18603. doi:10.1074/jbc.271.31.18596
Sommer, A. P. (2019). Mitochondrial cytochrome c oxidase is not the primary acceptor for near infrared light - it is mitochondrial bound water: the principles of low-level light therapy. Ann. Transl. Med. 7, S13. doi:10.21037/atm.2019.01.43
Søyland, E., Heier, I., Rodríguez-Gallego, C., Mollnes, T. E., Johansen, F. E., Holven, K. B., et al. (2011). Sun exposure induces rapid immunological changes in skin and peripheral blood in patients with psoriasis. Br. J. Dermatol 164 (2), 344–355. doi:10.1111/j.1365-2133.2010.10149.x
Sun, Q., Kim, H. E., Cho, H., Shi, S., Kim, B., and Kim, O. (2018). Red light-emitting diode irradiation regulates oxidative stress and inflammation through SPHK1/NF-κB activation in human keratinocytes. J. Photochem Photobiol. B 186, 31–40. doi:10.1016/j.jphotobiol.2018.05.015
Suschek, C. V., Briviba, K., Bruch-Gerharz, D., Sies, H., Kröncke, K. D., and Kolb-Bachofen, V. (2001). Even after UVA-exposure will nitric oxide protect cells from reactive oxygen intermediate-mediated apoptosis and necrosis. Cell Death Differ. 8 (5), 515–527. doi:10.1038/sj.cdd.4400839
Swerlick, R. A., and Lawley, T. J. (1993). Role of microvascular endothelial cells in inflammation. J. Invest. Dermatol. 100, 111S–115S. doi:10.1038/jid.1993.33
Tahir, R., and Mujtaba, G. (2004). Comparative efficacy of psoralen - UVA photochemotherapy versus narrow band UVB phototherapy in the treatment of psoriasis. J. Coll. Physicians Surg. Pak 14 (10), 593–595. doi:10.2004/jcpsp.593595
Talagas, M., Lebonvallet, N., and Misery, L. (2018). Intraepidermal nerve fibres are not the exclusive tranducers of nociception. J. Neurosci. Methods 306, 92–93. doi:10.1016/j.jneumeth.2018.05.013
Tang, L., Qin, H., Lin, S., and Liu, M. (2023). Effects of pulsed red and near-infrared light on neuroblastoma cells—pilot study on frequency and duty cycle. Photonics 10, 315. doi:10.3390/photonics10030315
Tekin, N. S., Ilter, N., Sancak, B., Ozden, M. G., and Gurer, M. A. (2006). Nitric oxide levels in patients with psoriasis treated with methotrexate. Mediat. Inflamm. 2006 (3), 16043. doi:10.1155/mi/2006/16043
Thatiparthi, A., Martin, A., Liu, J., and Wu, J. J. (2022). Risk of skin cancer with phototherapy in moderate-to-severe psoriasis: an updated systematic review. J. Clin. Aesthet. Dermatol 15 (6), 68–75.
Tripodi, N., Sidiroglou, F., Apostolopoulos, V., and Feehan, J. (2023). Transcriptome analysis of the effects of polarized photobiomodulation on human dermal fibroblasts. J. Photochem Photobiol. B 242, 112696. doi:10.1016/j.jphotobiol.2023.112696
Ulrich, K., and Jakob, U. (2019). The role of thiols in antioxidant systems. Free Radic. Biol. Med. 140, 14–27. doi:10.1016/j.freeradbiomed.2019.05.035
Umino, Y., and Denda, M. (2023). Effect of red light on epidermal proliferation and mitochondrial activity. Skin. Res. Technol. 29 (9), e13447. doi:10.1111/srt.13447
Van Praag, M. C., Tseng, L. N., Mommaas, A. M., Boom, B. W., and Vermeer, B. J. (1993). Minimising the risks of PUVA treatment. Drug Saf. 8 (5), 340–349. doi:10.2165/00002018-199308050-00002
Veleeparampil, M. M., Aravind, U. K., and Aravindakumar, C. T. (2009). Decomposition of S-nitrosothiols induced by UV and sunlight. Adv. Phys. Chem. 2009 (1), 890346. doi:10.1155/2009/890346
Vilas-Boas, E. A., Cabral-Costa, J. V., Ramos, V. M., Caldeira da Silva, C. C., and Kowaltowski, A. J. (2023). Goldilocks calcium concentrations and the regulation of oxidative phosphorylation: too much, too little, or just right. J. Biol. Chem. 299 (3), 102904. doi:10.1016/j.jbc.2023.102904
Wang, M., Luo, P., Shi, W., Guo, J., Huo, S., Yan, D., et al. (2021). S-Nitroso-L-Cysteine ameliorated pulmonary hypertension in the MCT-induced rats through anti-ROS and anti-inflammatory pathways. Oxid. Med. Cell Longev. 2021, 6621232. doi:10.1155/2021/6621232
Weihrauch, D., Keszler, A., Lindemer, B., Krolikowski, J., and Lohr, N. L. (2021). Red light stimulates vasodilation through extracellular vesicle trafficking. J. Photochem Photobiol. B 220, 112212. doi:10.1016/j.jphotobiol.2021.112212
West, H. C., and Bennett, C. L. (2017). Redefining the role of Langerhans cells as immune regulators within the skin. Front. Immunol. 8, 1941. doi:10.3389/fimmu.2017.01941
Wink, D. A., Hanbauer, I., Grisham, M. B., Laval, F., Nims, R. W., Laval, J., et al. (1996). Chemical biology of nitric oxide: regulation and protective and toxic mechanisms. Curr. Top. Cell Regul. 34, 159–187. doi:10.1016/s0070-2137(96)80006-9
Wong, T., Hsu, L., and Liao, W. (2013). Phototherapy in psoriasis: a review of mechanisms of action. J. Cutan. Med. Surg. 17 (1), 6–12. doi:10.2310/7750.2012.11124
Wu, Q., Xia, Y., Dai, K., Bai, P., Kwan, K. K. L., Guo, M. S. S., et al. (2020). Solar light induces the release of acetylcholine from skin keratinocytes affecting melanogenesis. Faseb J. 34 (7), 8941–8958. doi:10.1096/fj.202000708R
Yildirim, M., Inaloz, H. S., Baysal, V., and Delibas, N. (2003). The role of oxidants and antioxidants in psoriasis. J. Eur. Acad. Dermatol Venereol. 17 (1), 34–36. doi:10.1046/j.1468-3083.2003.00641.x
Yoshida, T., Inoue, R., Morii, T., Takahashi, N., Yamamoto, S., Hara, Y., et al. (2006). Nitric oxide activates TRP channels by cysteine S-nitrosylation. Nat. Chem. Biol. 2 (11), 596–607. doi:10.1038/nchembio821
Yu, H. S., Wu, C. S., Yu, C. L., Kao, Y. H., and Chiou, M. H. (2003). Helium-neon laser irradiation stimulates migration and proliferation in melanocytes and induces repigmentation in segmental-type vitiligo. J. Invest. Dermatol 120 (1), 56–64. doi:10.1046/j.1523-1747.2003.12011.x
Zastrow, L., Groth, N., Klein, F., Kockott, D., Lademann, J., and Ferrero, L. (2009). Detection and identification of free radicals generated by UV and visible light in ex vivo human skin. Int. J. Cosmet. Sci. 31, 402. doi:10.1111/j.1468-2494.2009.00511_2.x
Zhang, G., Yi, L., Wang, C., Yang, P., Zhang, J., Wang, J., et al. (2022). Photobiomodulation promotes angiogenesis in wound healing through stimulating the nuclear translocation of VEGFR2 and STAT3. J. Photochem Photobiol. B 237, 112573. doi:10.1016/j.jphotobiol.2022.112573
Zhang, R., and Qu, J. (2023). The mechanisms and efficacy of photobiomodulation therapy for arthritis: a comprehensive review. Int. J. Mol. Sci. 24 (18), 14293. doi:10.3390/ijms241814293
Zhang, Y., Song, S., Fong, C. C., Tsang, C. H., Yang, Z., and Yang, M. (2003). cDNA microarray analysis of gene expression profiles in human fibroblast cells irradiated with red light. J. Invest. Dermatol 120 (5), 849–857. doi:10.1046/j.1523-1747.2003.12133.x
Zhu, H., Luo, H., Li, Y., Zhou, Y., Jiang, Y., Chai, J., et al. (2013). MicroRNA-21 in scleroderma fibrosis and its function in TGF-β- regulated fibrosis-related genes expression. J. Clin. Immunol. 33 (6), 1100–1109. doi:10.1007/s10875-013-9896-z
Zimmerman, A., Bai, L., and Ginty, D. D. (2014). The gentle touch receptors of mammalian skin. Science 346 (6212), 950–954. doi:10.1126/science.1254229
Glossary
Keywords: red light, photobiomodulation, skin, metabolism, gene expression, nitric oxide (NO), photosensitized oxidation, sun exposure glossary
Citation: Herrera MA, Ribas AP, da Costa PE and Baptista MS (2024) Red-light photons on skin cells and the mechanism of photobiomodulation. Front. Photonics 5:1460722. doi: 10.3389/fphot.2024.1460722
Received: 06 July 2024; Accepted: 11 September 2024;
Published: 24 September 2024.
Edited by:
Marcelo Victor Pires de Sousa, D’Or Institute for Research and Education (IDOR), BrazilReviewed by:
Shuang Chang, Vanderbilt University, United StatesCopyright © 2024 Herrera, Ribas, da Costa and Baptista. This is an open-access article distributed under the terms of the Creative Commons Attribution License (CC BY). The use, distribution or reproduction in other forums is permitted, provided the original author(s) and the copyright owner(s) are credited and that the original publication in this journal is cited, in accordance with accepted academic practice. No use, distribution or reproduction is permitted which does not comply with these terms.
*Correspondence: Paulo Eduardo da Costa, cGFzdHJvazIwMTVAZ21haWwuY29t; Mauricio S. Baptista, YmFwdGlzdGFAaXEudXNwLmJy
†These authors have contributed equally to this work and share first authorship
‡These authors have contributed equally to this work and share last authorship