- Department of Neuroscience, Rockefeller Neuroscience Institute, West Virginia University, Morgantown, WV, United States
Artificial light at night (ALAN) is a pervasive circadian rhythm disruptor. Exposure to ALAN is associated with detrimental effects on physiology and behavior, including disrupted metabolism, immune function, endocrine function, and pain behavior. Given the detrimental effects of ALAN and other circadian rhythm disruptors on pain, we sought to understand how ALAN may alter the progression and severity of diabetic neuropathy. To do this, we used a previously reported high-fat diet and streptozotocin injection protocol to induce a type II diabetic phenotype in ∼8 week old female and male mice and then exposed the mice to either control or ALAN lighting conditions in 14:10 h light-dark cycles for 4 weeks. Male mice housed in control conditions exhibited reduced responsiveness to cold pain; in contrast, ALAN blunted this effect in male mice. ALAN exposure also elevated blood glucose and altered body mass loss in male mice. These effects were not present in female mice. The results of this study highlight the need to consider and study ALAN exposure and sex as a biological variable as risk factors in the treatment and mitigation of pain.
1 Introduction
Circadian rhythm disruption is a pervasive and nearly ubiquitous aspect of modern human life. Circadian rhythms are intrinsic biological processes that rhythmically fluctuate with a period of about 24 h; they are set to precisely 24 h each day by exposure to light during the circadian day (Vitaterna et al., 2001). These rhythms govern many aspects of behavior and physiology, including pain behavior (Segal et al., 2018; Palada et al., 2020; Bumgarner et al., 2021) and metabolic function. Circadian rhythms are aligned to the external environment primarily via solar light-dark cycle cues, and the synchronization of these rhythms with the external rhythmic environment of the Earth is crucial for health (Vitaterna et al., 2001; Patke et al., 2020).
Artificial light at night (ALAN) is one of the most pervasive disruptors of circadian rhythms (Falchi et al., 2016). ALAN exposure is directly associated with several detrimental health, physiological, and behavioral effects in humans and other animals, including elevated inflammation and obesity (Bumgarner and Nelson, 2021; Walker et al., 2021; Muscogiuri et al., 2022). Importantly, our recent work demonstrated that exposure to ALAN can exacerbate pain behavior in male mice after as few as four nights of exposure (Bumgarner et al., 2020). Other forms of circadian rhythm disruption are also associated with exacerbated pain behavior, including night shift work and sleep deprivation (Mun et al., 2022). Because of the intertwined relationship between circadian rhythms and pain, it is important to understand the effects of ALAN on pain beyond the context of typical states of health.
Type II diabetes (T2DM) is estimated to affect over 500 million adults worldwide, representing 10.5% of the adult human population (Robertson et al., 2022). Moreover, T2DM is estimated to represent ∼98% of global diabetes diagnoses. A large-scale epidemiological study recently reported that ALAN exposure is positively correlated with T2DM risk (Xu et al., 2023). ALAN can impair metabolic function, for example, by reducing insulin and glucose tolerance and elevating circulating free fatty acids and triglycerides (Russart and Nelson, 2018; Fleury et al., 2020; Mason et al., 2020). Work from our lab recently demonstrated that exposure to ALAN can exacerbate the progression of diabetes in a polygenic mouse model of T2DM (TALLYHO mice) (Russart et al., 2019).
Beyond the metabolic consequences of this disease, it is currently estimated that around 50% of individuals with T2DM will develop diabetic neuropathy at some point in their lives (Feldman, 2018; Feldman et al., 2019). This extends the risk potential for the effects of ALAN beyond metabolism.
In this study, we hypothesized that ALAN exposure exacerbates the progression of diabetic neuropathy in female and male mice in a mouse model of T2DM. Diabetes was induced per the protocol previously reported (Nath et al., 2017); then, mice were exposed to dim ALAN (5 lux) for 4 weeks. We predicted that ALAN exposure would exacerbate the progression of diabetes and diabetic neuropathy.
2 Materials and methods
2.1 Animals
Adult female and male Swiss Webster (CFW) mice (Charles River Laboratories) were obtained at 7–8 weeks of age and given 1 week to acclimate to standard vivarium conditions (14:10 h light:dark, i.e., overhead room lights on for 14 h and off for 10 h; lights off at ZT12; 150 ± 25:0 lux, 22°C ± 2°C; 12.0 × 6.5 × 5.5” polycarbonate cages). Following arrival, mice were housed in groups of five per cage. After the acclimation period, mice were singly housed, and the experiment began. Reverse osmosis water and food were provided ad libitum throughout the duration of the experiment. All studies were approved by the West Virginia University Institutional Animal Care and Use Committee, and animals were maintained in accordance with NIH Animal Welfare guidelines.
2.2 T2DM induction
To induce diabetes, we followed a previously published protocol (Nath et al., 2017). For 5 weeks starting at the onset of the experiment, mice were given ad libitum access to a high fat diet (21.0% protein, 24.6% carbohydrate, 54.4% fat per kilocalorie; TD.07011, Envigo). The high fat diet was replaced every 2 days between ZT03-05. At the beginning of the fourth week of the experiment, mice were given a series of five i. p. injections of 40 mg/kg of streptozotocin (STZ; ICN10055701, Fisher). STZ was prepared daily immediately before injections by dissolving STZ in 0.1M citrate buffer pH 4.5 at a concentration of 10 mg/mL. Injections were administered during the light phase between ZT03-05. After the 5 week T2DM induction period, glucose measurements were measured from tail vein blood using a Countour Next 7,377 glucometer and Contour Next 7,278 glucose strips between ZT10-12. Following glucose measurements, animals were pseudorandomly assigned to either LD or ALAN conditions to ensure an even distribution of animals with glucose levels above/below 200 mg/dL between groups. Body mass (g) was recorded weekly throughout the study starting at the beginning of the T2DM induction protocol. At the conclusion of the experiment, all animals were diabetic based on the 200 mg/Dl cutoff.
2.3 ALAN conditions
After the 5 weeks of T2DM induction, animals were separated into either LD or ALAN housing conditions (n = 15 animals per group). Both groups were maintained in 14:10 light-dark cycles with 150 ± 25:0 lux during the day. ALAN animals were exposed to 5 ± 1 lux of ALAN during the 10-h dark phase. The ALAN sources were Luma5 Standard LED light strips (1.5 W/ft, 5000K “cool white”, peak wavelength of 455.0 nm; centroid wavelength of 563.0 nm; RGB Ratio: R = 18.7% G = 76.1% B = 5.2%; Hitlights Inc.). Lux calibrations were conducted by placing a Mavolux 5023C illuminance meter (Gossen) with the light sensor facing toward the ceiling in the center of an empty prepared cage (bedding, nesting, water bottle, and food; no animal). Animals remained in either LD or ALAN conditions for a total of 4 weeks.
2.4 Behavioral testing
Pain behavioral testing consisted of a cold plate and electric von Frey testing. Baseline pain behavioral measurements were taken 1 day before the onset of ALAN/LD experimental housing conditions after the T2DM induction. Pain behavior was then assessed after 2 and 4 weeks of experimental housing conditions. All behavioral testing was conducted during the light phase between ZT03-06. Animals were given a minimum of 30 min to acclimate to the behavioral suite prior to testing. All experimenters conducting behavioral testing were blinded to the experimental condition of the animals. Experimental groups were distributed randomly across the testing period as a result of the pseudorandomization above to avoid batch effects.
Cold plate testing (IITC Life Science Inc.) was performed at 0.5 ± 1.0 C°. Mice were gently placed onto the cold plate, and a stopwatch was started as soon as their hind paws touched the plate. Two observers watched for any sign of nocifensive response, which included hind paw shakes/extended withdrawals/licking, jumping, or squeaking (Deuis et al., 2017). As soon as the first nocifensive response was observed, the latency to response was recorded and the animal was promptly removed from the plate. Animals were removed from the plate after an ethical cutoff threshold of 90 s if no responses were observed.
Electric von Frey testing (Bioseb) was conducted using opaque chambers (80 × 115 × 145 mm; Bioseb) on top of a wire mesh table (Bioseb). Mice were given 15 min to acclimate to the chambers before the onset of testing. Testing was conducted in a series of four rounds, where the wire tip of the electric von Frey was slowly pressed upwards on the plantar surface of a consistent hind paw of each mouse. The maximum force needed to elicit a response was recorded as the mechanical withdrawal threshold. Responses to the von Frey were defined as paw lifts, paw shakes, or jumping. The withdrawal thresholds from the four rounds were averaged to produce a final threshold score for each animal at each time point. Reductions in maximum withdrawal threshold were correlated with mechanical allodynia (Martinov et al., 2013).
2.5 Statistical analyses
All data were analyzed using two-way repeated measures ANOVAs (time, lighting condition, and sex as factors). A priori planned multiple comparisons were conducted using Fisher’s LSD. All data presented in the figures are presented from raw data recordings. All analyses were conducted using Prism 9.5.1. An alpha of 0.05 was used as the cutoff for statistical significance.
3 Results
3.1 ALAN elevates glucose in male, but not female T2DM mice
After 4 weeks of exposure to ALAN, male mice exhibited elevated blood glucose levels (Figure 1A; p < 0.05) with a significant interaction between lighting condition exposure and time (p < 0.05; F2,56 = 4.615). There was no significant effect of ALAN exposure on female blood glucose at any time point. At the final time point, there was no difference in the distribution of animals that were considered diabetic per the defined cutoff level. There was a main effect of time on blood glucose levels in both male (Figure 1A; p < 0.05; F2,56 = 98.03) and female mice (Figure 1C; p < 0.05; F2,56 = 114.1) from the conclusion of the T2DM induction and the onset of the experimental lighting condition housing.
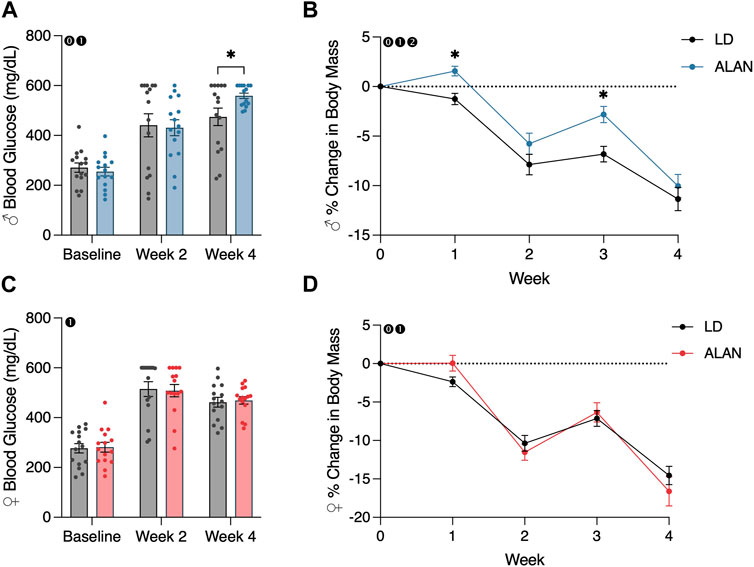
FIGURE 1. ALAN elevates blood glucose and alters body mass loss. Blood glucose levels following the onset of experimental housing in either ALAN or LD conditions (A,C). The percent change in body mass following the onset of experimental housing (B,D). n = 15. All data are represented as mean ± SEM. Enclosed 0–Interaction between Lighting Condition and Time; enclosed 1–Main effect of Time; enclosed 2–Main effect of Lighting Condition. *p < 0.05.
3.2 ALAN alters body mass loss in T2DM
A main effect of lighting condition (p < 0.05; F1,28 = 5.165) and an interaction between lighting condition and time (p < 0.05; F4,112 = 3.325) on body mass were observed in male mice (Figure 1B). When examining individual time point differences, ALAN and LD body masses only differed at weeks one and three in male mice (Figure 1B; p < 0.05). An interaction between lighting condition and time on body mass was observed in female mice (Figure 1D; p < 0.05; F4,112 = 2.741), but no individual time points were significantly different (p < 0.05). A main effect of time was observed in both male (p < 0.05; F2,56 = 124.9) and female mice (p < 0.05; F2,56 = 156.3), indicating a loss in body mass following the conclusion of the T2DM induction protocol. Lastly, there was a significant effect of the T2DM induction protocol on body mass in both male (Supplementary Figure S1A; p < 0.05; F5,140 = 406.8) and female mice (Supplementary Figure S1B; p < 0.05; F5,140 = 229.7).
3.3 ALAN alters cold neuropathy in male, but not female T2DM mice
Male mice exposed to ALAN for 4 weeks exhibited reduced cold plate withdrawal latencies relative to the LD diabetic male mice (Figure 2A; p < 0.05). This difference coincided with a significant interaction between lighting condition and time (p < 0.05; F2,56 = 4.109). When considering within-group changes in cold plate withdrawal latencies, the male ALAN mice had no significant changes in cold plate withdrawal thresholds at week four relative to baseline (Figure 2A; p > 0.05). In contrast, male LD mice exhibited elevated withdrawal thresholds at week four relative to their baseline measurements (Figure 2A; p < 0.05). There was a main effect of time on cold plate withdrawal latencies in male mice (Figure 2A; p < 0.05; F2,56 = 6.358). No effect of ALAN was observed on cold plate withdrawal thresholds in female mice, but both LD and ALAN female mice had elevated cold plate withdrawal latencies at week four relative to their baseline measurements (Figure 2C; p < 0.05) with a main effect of time (p < 0.05; F2,56 = 6.767).
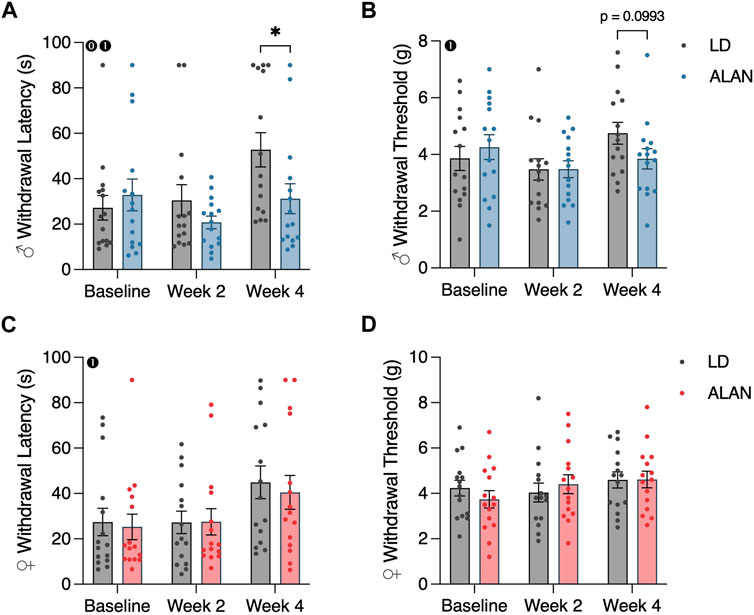
FIGURE 2. ALAN alters cold plate responsiveness in male T2DM mice. Cold plate withdrawal latencies (A,C), and von Frey mechanical withdrawal thresholds (B,D) of female and male mice following the onset of experimental housing. n = 15. All data are represented as mean ± SEM. Enclosed 0–Interaction between Lighting Condition and Time; enclosed 1–Main effect of Time; enclosed 2–Main effect of Lighting Condition. *p < 0.05.
3.4 ALAN did not alter mechanical withdrawal thresholds in T2DM mice
ALAN did not affect mechanical withdrawal latencies in female or male mice (Figures 2B, D; p > 0.05). There was a main effect of time on mechanical withdrawal thresholds in male mice (Figure 2B; p < 0.05; F2,56 = 3.713), indicating a progression of hypoesthesia following the conclusion of the T2DM induction. This effect was not present in females.
4 Discussion
This study sought to understand the effects of ALAN exposure on the progression of diabetic neuropathy in a mouse model of T2DM. Mice first were exposed to a multi-week high-fat diet and low-dose series of streptozotocin to induce a T2DM phenotype based on a previously validated protocol (Nath et al., 2017). Male mice exposed to LD conditions exhibited reduced responsiveness to noxious cold stimuli relative to male ALAN counterparts at the conclusion of the study. Male mice also exhibited elevated blood glucose at the conclusion of the study, and ALAN blunted the loss of body mass in male mice at various time points throughout the study. In contrast, a stark sex difference was observed for each examined metric. ALAN had no observable effects on pain behavior or blood glucose in female mice, but it did alter female body mass loss following T2DM induction.
In humans, diabetic peripheral neuropathy progression can vary, but generally, nociceptive large-diameter thinly myelinated Aδ fibers and small-diameter unmyelinated C fibers are first affected, leading to a period of hyperalgesia and allodynia. As the neuropathy progresses and the nociceptive Aδ and C fibers continue to degenerate, larger diameter myelinated Aβ fibers also begin to degenerate, ultimately leading to numbness, hypoalgesia, and loss of sensation (O'Brien et al., 2014). The progression of diabetic neuropathy in mice is not as robustly characterized. Two studies reported a progression of hypoesthesia (reduced sensitivity to innocuous touch) and hypoalgesia following T2DM induction, but not hypersensitivity (De Gregorio et al., 2018; Sasajima et al., 2022). It is possible that the initial phase of hypersensitivity was not replicated in these studies due to the acute period of T2DM induction. In contrast to the absence of hypoesthesia in female mice in this study, another study reported long-term allodynia using the same T2DM induction protocol as this study, although their study examined female C57BL/6 mice (Castañeda-Corral et al., 2021).
The difference in cold plate withdrawal latencies in male mice exposed to ALAN could be interpreted in two separate ways. One may consider that ALAN led to the development of cold hypersensitivity in male mice relative to the LD counterparts. In contrast, one might consider that ALAN did not induce a state of hypersensitivity, but instead delayed the onset of hypoalgesia that was noted in male LD mice. Distinguishing between these two perspectives would require a follow-up study that tested pain behavior more frequently and for longer periods of time to examine the progression of pain behavior. Further insight into the underlying mechanisms behind this effect would require additional examinations, including the assessment of peripheral inflammation and nerve conduction velocity.
In this experiment, sex differences in the effect of ALAN on changes in metabolic and behavioral phenotypes were observed. These differences may be explained by underlying sex differences in circadian rhythm biology or the effects of circadian rhythm disruption. Indeed, there are numerous sex differences in circadian rhythms, including the function of the immune, metabolic, and endocrine systems (Munyoki et al., 2022; Walton et al., 2022). Moreover, there are sex differences in the metabolic consequences of circadian rhythm disruption, including the effects of ALAN (Masís-Vargas et al., 2019; Rumanova et al., 2020), simulated shift work (Qian et al., 2019), and jet lag (Feliciano et al., 2019), demonstrating precedence for the observed sex differences in this study. However, further experiments will be required to confirm the sex differences found in this study and if verified to identify underlying mechanisms.
The observed sex differences in pain behavior and metabolic phenotypes in this study may be further explained by underlying sex differences in diabetes or diabetic neuropathy (Abraham et al., 2018; Kautzky-Willer et al., 2023). Numerous metabolic risk factors for T2DM present differently between men and women, including glucose and insulin sensitivity, hormonal regulation of metabolism, and hypertension (Kautzky-Willer et al., 2016; Kautzky-Willer et al., 2023). There are few reports on sex differences in diabetic neuropathy, but some sex differences have been reported. Neuropathy appears to present earlier in men than women (Aaberg et al., 2008; Kamenov et al., 2010), whereas one study reported that women with diabetic polyneuropathy report higher pain intensities (Abraham et al., 2018). There is minimal preclinical research on sex differences in diabetic neuropathy. One study using a diet-induced ‘pre-diabetes’ state reported no sex differences in pain behavior (Elzinga et al., 2021). In all, there is minimal documented evidence of the underlying mechanisms that lead to sex differences in diabetic neuropathy. We propose that the sex differences observed in this study likely are a result of underlying differences in either the progression of neuropathy following the diabetes induction protocol or the underlying sex differences in the effects of ALAN exposure.
In conclusion, exposure to ALAN exacerbates cold pain behavior and blood glucose levels in male mice, but not female mice, and ALAN altered the loss of body mass following T2DM induction. Further experimentation will be required to understand the underlying mechanisms behind the pain-related consequences of ALAN and the observed sex differences. In addition, follow-up work might seek to assess these effects at different time points throughout the day, given the above noted circadian variations in pain behavior. These results highlight the continuing need to consider circadian rhythms, the mitigation of circadian rhythm disruption, and sex as a biological variable in the treatment and management of pain.
Data availability statement
The raw data supporting the conclusion of this article will be made available by the authors, without undue reservation.
Ethics statement
The animal study was approved by the West Virginia University Institutional Animal Care and Use Committee. The study was conducted in accordance with the local legislation and institutional requirements.
Author contributions
JB: Conceptualization, Formal Analysis, Investigation, Methodology, Project administration, Supervision, Visualization, Writing–original draft, Writing–review and editing. RW: Investigation, Methodology, Writing–review and editing. JB: Investigation, Methodology, Writing–review and editing. RN: Conceptualization, Funding acquisition, Methodology, Project administration, Resources, Supervision, Validation, Writing–review and editing.
Funding
The author(s) declare financial support was received for the research, authorship, and/or publication of this article. The authors were supported by grants from NINDS (R01NS092388 to RN and ACD), NCCIH (R21AT011238-01 to RN), and NIGMS award number 5U54GM104942. The content is solely the responsibility of the authors and does not necessarily represent the official views of the National Institutes of Health.
Acknowledgments
We would like to acknowledge the animal husbandry staff who provided excellent and attentive care throughout the duration of this experiment.
Conflict of interest
The authors declare that the research was conducted in the absence of any commercial or financial relationships that could be construed as a potential conflict of interest.
Publisher’s note
All claims expressed in this article are solely those of the authors and do not necessarily represent those of their affiliated organizations, or those of the publisher, the editors and the reviewers. Any product that may be evaluated in this article, or claim that may be made by its manufacturer, is not guaranteed or endorsed by the publisher.
Supplementary material
The Supplementary Material for this article can be found online at: https://www.frontiersin.org/articles/10.3389/fphot.2024.1323539/full#supplementary-material
References
Aaberg, M. L., Burch, D. M., Hud, Z. R., and Zacharias, M. P. (2008). Gender differences in the onset of diabetic neuropathy. J. Diabetes Compl 22, 83–87. doi:10.1016/j.jdiacomp.2007.06.009
Abraham, A., Barnett, C., Katzberg, H. D., Lovblom, L. E., Perkins, B. A., and Bril, V. (2018). Sex differences in neuropathic pain intensity in diabetes. J. Neurol. Sci. 388, 103–106. doi:10.1016/j.jns.2018.03.008
Bumgarner, J. R., and Nelson, R. J. (2021). Light at night and disrupted circadian rhythms alter physiology and behavior. Integr. Comp. Biol. 61 (3), 1160–1169. doi:10.1093/icb/icab017
Bumgarner, J. R., Walker, W. H., and Nelson, R. J. (2021). Circadian rhythms and pain. Neurosci. Biobehav. Rev. 129, 296–306. doi:10.1016/j.neubiorev.2021.08.004
Bumgarner, J. R., Walker, W. H., Liu, J. A., Walton, J. C., and Nelson, R. J. (2020). Dim light at night exposure induces cold hyperalgesia and mechanical allodynia in male mice. Neuroscience 434, 111–119. doi:10.1016/j.neuroscience.2020.03.022
Castañeda-Corral, G., Velázquez-Salazar, N. B., Martínez-Martínez, A., Taboada-Serrano, J. N., Núñez-Aragón, P. N., González-Palomares, L., et al. (2021). Characterization of mechanical allodynia and skin innervation in a mouse model of Type-2 diabetes induced by cafeteria-style diet and low-doses of Streptozotocin. Front. Pharmacol. 11, 628438. doi:10.3389/fphar.2020.628438
De Gregorio, C., Contador, D., Campero, M., Ezquer, M., and Ezquer, F. (2018). Characterization of diabetic neuropathy progression in a mouse model of type 2 diabetes mellitus. Biol. Open 7, bio036830. doi:10.1242/bio.036830
Deuis, J. R., Dvorakova, L. S., and Vetter, I. (2017). Methods used to evaluate pain behaviors in rodents. Front. Mol. Neurosci. 10, 284. doi:10.3389/fnmol.2017.00284
Elzinga, S. E., Savelieff, M. G., O’Brien, P. D., Mendelson, F. E., Hayes, J. M., and Feldman, E. L. (2021). Sex differences in insulin resistance, but not peripheral neuropathy, in a diet-induced prediabetes mouse model. Dis Models Mech 14, dmm048909. doi:10.1242/dmm.048909
Falchi, F., Cinzano, P., Duriscoe, D., Kyba, C. C., Elvidge, C. D., Baugh, K., et al. (2016). The new world atlas of artificial night sky brightness. Sci. Adv. 2, e1600377. doi:10.1126/sciadv.1600377
Feldman, E. L. (2018). Epidemiology and classification of diabetic neuropathy. Railway Town, Tehran: UpToDate®. Availble from: https://www.uptodate.com/contents/epidemiology-and-classification-of-diabetic-neuropathy (Accessed February 17, 2016).
Feldman, E. L., Callaghan, B. C., Pop-Busui, R., Zochodne, D. W., Wright, D. E., Bennett, D. L., et al. (2019). Diabetic neuropathy. Nat. Rev. Dis. Prim. 5, 41. doi:10.1038/s41572-019-0092-1
Feliciano, E. M. C., Rifas-Shiman, S. L., Quante, M., Redline, S., Oken, E., and Taveras, E. M. (2019). Chronotype, social jet lag, and cardiometabolic risk factors in early adolescence. JAMA Pediatr. 173, 1049–1057. doi:10.1001/jamapediatrics.2019.3089
Fleury, G., Masís-Vargas, A., and Kalsbeek, A. (2020). Metabolic implications of exposure to light at night: lessons from animal and human studies. Obesity 28, S18–S28. doi:10.1002/oby.22807
Kamenov, Z. A., Parapunova, R. A., and Georgieva, R. T. (2010). Earlier development of diabetic neuropathy in men than in women with type 2 diabetes mellitus. Gend. Med. 7, 600–615. doi:10.1016/j.genm.2010.11.001
Kautzky-Willer, A., Harreiter, J., and Pacini, G. (2016). Sex and gender differences in risk, pathophysiology and complications of type 2 diabetes mellitus. Endocr. Rev. 37, 278–316. doi:10.1210/er.2015-1137
Kautzky-Willer, A., Leutner, M., and Harreiter, J. (2023). Sex differences in type 2 diabetes. Diabetologia 66, 986–1002. doi:10.1007/s00125-023-05891-x
Martinov, T., Mack, M., Sykes, A., and Chatterjea, D. (2013). Measuring changes in tactile sensitivity in the hind paw of mice using an electronic von Frey apparatus. J. Vis. Exper 82, e51212. doi:10.3791/51212
Masís-Vargas, A., Hicks, D., Kalsbeek, A., and Mendoza, J. (2019). Blue light at night acutely impairs glucose tolerance and increases sugar intake in the diurnal rodent Arvicanthis ansorgei in a sex-dependent manner. Physiol. Rep. 7, e14257. doi:10.14814/phy2.14257
Mason, I. C., Qian, J., Adler, G. K., and Scheer, F. A. J. L. (2020). Impact of circadian disruption on glucose metabolism: implications for type 2 diabetes. Diabetologia 63, 462–472. doi:10.1007/s00125-019-05059-6
Mun, C. J., Burgess, H. J., Sears, D. D., Parthasarathy, S., James, D., Altamirano, U., et al. (2022). Circadian rhythm and Pain: a review of current research and future implications. Cur. Sleep. Med. Rep. 8, 114–123. doi:10.1007/s40675-022-00228-3
Munyoki, S. K., Goff, J. P., Kolobaric, A., Long, A., Mullett, S. J., Burns, J. K., et al. (2022). Circadian rhythms in the gut microbiota shape sex differences in host gene expression and metabolism. bioRxiv. doi:10.1101/2022.09.15.508006
Muscogiuri, G., Poggiogalle, E., Barrea, L., Tarsitano, M. G., Garifalos, F., Liccardi, A., et al. (2022). Exposure to artificial light at night: a common link for obesity and cancer. Eur. J. Cancer 173, 263–275. doi:10.1016/j.ejca.2022.06.007
Nath, S., Ghosh, S. K., and Choudhury, Y. (2017). A murine model of type 2 diabetes mellitus developed using a combination of high fat diet and multiple low doses of streptozotocin treatment mimics the metabolic characteristics of type 2 diabetes mellitus in humans. J. Pharmacol. Toxicol. Methods 84, 20–30. doi:10.1016/j.vascn.2016.10.007
O’Brien, P. D., Sakowski, S. A., and Feldman, E. L. (2014). Mouse models of diabetic neuropathy. ILAR J. 54, 259–272. doi:10.1093/ilar/ilt052
Palada, V., Gilron, I., Canlon, B., Svensson, C. I., and Kalso, E. (2020). The circadian clock at the intercept of sleep and pain. Pain 161, 894–900. doi:10.1097/j.pain.0000000000001786
Patke, A., Young, M. W., and Axelrod, S. (2020). Molecular mechanisms and physiological importance of circadian rhythms. Nat. Rev. Mol. Cell. Biol. 21, 67–84. doi:10.1038/s41580-019-0179-2
Qian, J., Morris, C. J., Caputo, R., Wang, W., Garaulet, M., and Scheer, F. A. J. L. (2019). Sex differences in the circadian misalignment effects on energy regulation. Proc. Natl. Acad. Sci. U. S. A. 116, 23806–23812. doi:10.1073/pnas.1914003116
Robertson, R. P., Nathan, D. M., and Mulder, J. E. (2022). Type 2 diabetes mellitus: prevalence and risk factors. Railway Town, Tehran: UpToDate. Jan 25.
Rumanova, V. S., Okuliarova, M., and Zeman, M. (2020). Differential effects of constant light and dim light at night on the circadian control of metabolism and behavior. Int. J. Mol. Sci. 21, 5478. doi:10.3390/ijms21155478
Russart, K. L. G., Chbeir, S. A., Nelson, R. J., and Magalang, U. J. (2019). Light at night exacerbates metabolic dysfunction in a polygenic mouse model of type 2 diabetes mellitus. Life Sci. 231, 116574. doi:10.1016/j.lfs.2019.116574
Russart, K. L. G., and Nelson, R. J. (2018). Light at night as an environmental endocrine disruptor. Physiol. Behav. 190, 82–89. doi:10.1016/j.physbeh.2017.08.029
Sasajima, S., Kondo, M., Ohno, N., Ujisawa, T., Motegi, M., Hayami, T., et al. (2022). Thermal gradient ring reveals thermosensory changes in diabetic peripheral neuropathy in mice. Sci. Rep. 12, 9724–9813. doi:10.1038/s41598-022-14186-x
Segal, J. P., Tresidder, K. A., Bhatt, C., Gilron, I., and Ghasemlou, N. (2018). Circadian control of pain and neuroinflammation. J. Neurosci. Res. 96, 1002–1020. doi:10.1002/jnr.24150
Vitaterna, M. H., Takahashi, J. S., and Turek, F. W. (2001). Overview of circadian rhythms. Alcohol Res. Health 25, 85–93.
Walker, W. H., Bumgarner, J. R., Becker-Krail, D. D., May, L. E., Liu, J. A., and Nelson, R. J. (2021). Light at night disrupts biological clocks, calendars, and immune function. Semin. Immunopathol. 44, 165–173. doi:10.1007/s00281-021-00899-0
Walton, J. C., Bumgarner, J. R., and Nelson, R. J. (2022). Sex differences in circadian rhythms. Cold Spring Harb. Perspect. Biol. 14, a039107. doi:10.1101/cshperspect.a039107
Keywords: artificial light at night, circadian rhythm disruption, type II diabetes mellitus, cold neuropathy, cold hypersensitivity, sex differences, ALAN, T2DM
Citation: Bumgarner JR, White RC, Brown JA and Nelson RJ (2024) Artificial light at night alters progression of cold neuropathy in a sex-dependent manner in a mouse model of type II diabetes mellitus. Front. Photonics 5:1323539. doi: 10.3389/fphot.2024.1323539
Received: 18 October 2023; Accepted: 19 January 2024;
Published: 06 February 2024.
Edited by:
Bahman Anvari, University of California, Riverside, United StatesReviewed by:
Yayao Ma, University of California, Los Angeles, United StatesMichael Smolensky, The University of Texas at Austin, United States
Copyright © 2024 Bumgarner, White, Brown and Nelson. This is an open-access article distributed under the terms of the Creative Commons Attribution License (CC BY). The use, distribution or reproduction in other forums is permitted, provided the original author(s) and the copyright owner(s) are credited and that the original publication in this journal is cited, in accordance with accepted academic practice. No use, distribution or reproduction is permitted which does not comply with these terms.
*Correspondence: Jacob R. Bumgarner, anJidW1nYXJuZXJAbWl4Lnd2dS5lZHU=