- 1Departamento de Ciências Fisiológicas, Universidade Federal do Estado do Rio de Janeiro, UNIRIO, Rio de Janeiro, Brazil
- 2Departamento de Genética, Universidade do Estado do Rio de Janeiro, UERJ, IBRAG, Rio de Janeiro, Brazil
- 3Departamento de Biofísica, Universidade do Estado do Rio de Janeiro, UERJ, IBRAG, Rio de Janeiro, Brazil
Currently, light-emitting diodes (LEDs) are considered a substitute for low-power lasers in phototherapy protocols. LEDs enable photobiomodulation on biological tissues and are considered safe and economical. However, the molecular and metabolic mechanisms involved in LED-induced photobiomodulation are not yet fully understood. This review summarizes the metabolic mechanisms involved in LED-induced photobiomodulation in biological tissues under different irradiation parameters and conditions. Studies on LED-induced metabolism photobiomodulation were accessed using scientific article databases, whose findings were summarized in terms of molecular and cellular mechanisms. Data from the accessed studies suggested that the molecular mechanism of LED-induced photobiomodulation involves photoacceptors, such as cytochrome C oxidase, membrane ion channels, mitochondrial modulation, and the production of ROS.
Introduction
A brief history of light-emiting diodes therapy
The photobiomodulation effect was first described in the 1960s by a Hungarian surgeon, Professor Endre Mester, of the Semmelweis University of Medicine. Mester aimed to reproduce an experiment previously reported by Paul McGuff in 1965, which involved analyzing the effect of a ruby laser on a model of malignant tumors in rats (McGuff et al., 1965). Part of the methodology consisted of shaving the fur and making an incision on the back of the rats to transplant tumors. However, the laser used in the experiment was not as powerful as that used by McGuff. Instead of obtaining the expected results, Mester observed an increase in the healing process of incisions and fur growth in irradiated areas. Thus, the first report on the effects of low-level ruby laser (694 nm) on wound healing and hair growth was published (Mester et al., 1968; Mester et al., 1971). After obtaining positive results for the stimulating effects of a low-level laser, Mester initiated research on the mechanisms underlying the therapeutic potential of the laser and introduced phototherapy in the treatment of hard-to-heal wounds and ulcers (Mester et al., 1985).
The first visible light-emitting diode (GaAsP LED) was invented in 1962 by Nick Holonyak and Bevacqua in New York (Holonyak and Bevacqua, 1962) however, its therapeutic applications began to receive more attention from researchers between 1980 and 1990. LEDs emit light from a solid material, where the energy in an electric current is converted into luminous energy (electroluminescence), emitting electromagnetic radiation in the visible, infrared, or ultraviolet spectrum (Schubert, 2006, p. 1). In the 1980s, NASA conducted experiments using LEDs to cultivate plants for space travel (Nasa.gov [homepage on the internet], 2009). Subsequently, researchers investigated whether the radiation emitted from LEDs had the potential to inhibit bone and muscle loss and increase the body’s capacity to heal wounds in astronauts (Spinoff.nasa.gov [homepage on the internet], 2008). From these initial findings on LED-induced photobiomodulation, interest in this area was established, and the response of biological tissues to LED therapy (LEDT) has since been extensively reported in studies aimed at elucidating their mechanisms of action.
Mechanisms of action of light-emiting diodes therapy light-emiting diodes therapy and effector molecules
Optical window and tissue absorption
Phototherapy is based on the photochemical process caused by the absorption of energy from photons that make up the radiation beam by photoacceptors, which can be molecules or parts of biological molecules. Therefore, an important aspect that needs to be considered to determine the luminous spectrum that will be used is the optical property of this tissue.
Radiation penetration in tissues depends on their absorptive characteristics. The less radiation absorbed by the biological tissue, the greater its penetration. For phototherapy, the choice of radiation owing to its penetration potential is related to the depth of the target tissue. Therefore, an essential concept in phototherapy is the “therapeutic window,” or “optical window,” of a tissue. The optical tissue window is the spectral range in which most tissues respond as sufficiently weak absorbers that allow for significant light penetration (Vo-Dinh, 2003). Radiations emitted from low-power LEDs as well as those used in photodynamic therapy (PDT) are included in the optical window of LED therapy, which ranges from the end of the ultraviolet A (UV-A) spectrum to the near-infrared (390–1,200 nm).
Biological tissues have a variety of chromophores, such as melanin, cytochromes, opsins, flavins, deoxyhemoglobin, and water (Salehpour et al., 2018). Because they consist predominantly of water, it is crucial that the absorption potential of this molecule be considered. Radiation absorption by water occurs mainly in the infrared (λ > 1,200 nm) and ultraviolet (λ < 200 nm) spectra. In the spectral range of 650–1,200 nm (red to near-infrared radiation), radiation absorption by water and organic molecules is low, resulting in a high degree of radiation penetration. Thus, red and near-infrared radiation can reach deeper tissues and can be absorbed by photoacceptors (Gomes, 2011). The most significant tissue absorption and scattering occurs for blue light, as the main chromophores found in superficial biological tissues have high radiation absorption for radiation at short wavelengths; consequently, radiation penetration is low (Huang et al., 2009).
Cytochrome c oxidase and mitochondrial modulation
Although the mechanisms that mediate photobiomodulation are not yet well understood, the mitochondrial enzyme cytochrome c oxidase (CoX) is considered by many researchers as the primary photoacceptor, and the events resulting from the absorption of radiation energy by this protein are responsible for the biological effects triggered (Beauvoit et al., 1994; Karu, 1999; Karu, 2010). This enzyme, known as complex IV, is a terminal enzyme of the electron transport chain and a member of the heme/copper oxidase superfamily, which is in the inner membrane of the mitochondria and acts on the transfer of electrons from mitochondrial cytochrome to molecular oxygen (Rich, 2017). The hypothesis that CoX is the primary chromophore responsible for the effects of phototherapy involves stimulating oxidative metabolism through the photosensitization of the metal sites in the CoX structure. It has been suggested that such a process promotes an increase in available electron levels for the reduction of dioxygen in the catalytic center of cytochrome c oxidase, which leads to changes in the redox reactions carried out by the respiratory chain, increased proton pumping, and the activity of the internal mitochondrial membrane (Albuquerque-Pontes et al., 2015). This alteration in the mitochondrial membrane potential promotes an increase in cyclic adenosine monophosphate and the synthesis of adenosine triphosphate (ATP) (Wu et al., 2014). Consequently, the synthesis of DNA and RNA is increased, which alters cellular activity and metabolism (Karu, 1987).
Dissociation of nitric oxide from CoX
It is well established that nitric oxide (NO) acts as a quick and reversible inhibitor of mitochondrial respiration when present at physiological levels below 100 nM (Antunes et al., 2007). This reversible inhibition occurs because of the production of nitrosyl complexes in the heme group of cytochrome a3 in CoX (Brown, 2001). NO acts as a regulator of CoX activity by competing with O2 for enzymes of the respiratory chain. Thus, another reaction identified as being responsible for the metabolic response of CoX energization is the dissociation of NO from the CoX catalytic center. Dungel and co-workers (Dungel et al., 2008) reported that, depending on the wavelength, visible light restored the rate of normal mitochondrial respiration after promoting the recovery of NO-inhibited mitochondria.
ATP
Cell metabolism is positively stimulated by an increase in ATP levels, which promotes photoacceptor sensitization. In addition, it can elicit responses much broader than those described by its essential energy function within cells because the ATP molecule also has signaling potential, acting on intercellular communication and producing different results by activating P2 family receptors (P2X and P2Y) in purinergic signaling. The activation of P2Y receptors triggers a cascade of reactions that liberates intracellular calcium stores (Khakh and Burnstock, 2009; Burnstock, 2018).
Modulation of reactive oxygen species
Stimulation of ATP production generates the production of reactive oxygen species (ROS). High levels of ROS have deleterious effects on molecules, including membrane proteins, nucleic acids, and lipids. However, at low levels, ROS has positive effects (Di Meo et al., 2018). Studies have suggested a relationship between the proliferative effect of red-to-near-infrared radiation and a moderate increase in the intracellular level of ROS in normal cells (Wang et al., 2017; Amaroli et al., 2019). It is widely accepted that the beneficial effects of ROS involve transcription factors (Schreck et al., 1991). Nuclear factor kappa B (NF-κB) induces gene transcription, promoting positive photobiomodulation (Huang et al., 2009). However, in injured cells or under stress conditions where oxidative stress is increased, exposure to low-power red and near-infrared laser irradiation tends to decrease the intracellular concentration of ROS (Silveira et al., 2016).
Low-power irradiation at higher doses can promote high ROS production and oxidative effects on nucleic acids (Wu et al., 2007; Cadet and Wagner, 2013). Excess ROS induces the formation of non-selective channels through mitochondrial permeability transition pores (mPTP), enhancing inner mitochondrial membrane permeability (Zorov et al., 2000; Bernardi et al., 2099). Jacobson and Duchen (Jacobson and Duchen, 2002) reported an interaction between ROS and Ca+2 and subsequent transient mitochondrial depolarization with the opening of the mPTP. mPTP has protective functions when transiently stimulated, such as preventing mitochondrial damage by releasing calcium located in the matrix through pores. When prolonged, its induction is usually related to damage to the mitochondria and cell death pathways (Korge et al., 2011). The unregulated increase in the permeability of the mitochondrial membrane causes its dysfunctionalization through the entry of solutes, the dilation of the matrix, and the disruption of the outer membrane (Kim et al., 2003; Kinnally and Antonsson, 2007). This disruption causes the release of proapoptotic proteins into the cytosol. Injury to these organelles is crucial for cell apoptosis (Desagher and Martinou, 2000; Forte and Bernardi, 2006). In addition, the opening of long-lasting mPTP, which increases ROS levels, further stimulates ROS production, which threatens the functioning of the mitochondrial population (Zorov et al., 2014).
Nuclear factor kappa B
NF-KB is regulated by changes in the redox state, which modulate several cellular functions, including the expression of multiple protective and stimulating genes. NF-kB acts on enzymes, growth and transcription factors, stress response genes, apoptosis, and cell cycle regulators (D’Angio and Finkelstein, 2000). In addition, the activation of this nuclear factor promotes the positive regulation of inflammatory cytokines (IL-6, IL-8, and TNF-α), cyclooxygenase 2 (COX-2), nitric oxide synthase (NOS), and chemokines (CCL2 and CXCL8) (Pires et al., 2018). NF-kB dimers exist in the cytoplasm but are inhibited by their inhibitory proteins (IkB), which regulate the nuclear translocation of NF-kB. ROS play a key role in the phosphorylation of the IkB protein. Under the action of ROS and the removal of IkB, the NF-kB protein is released and activated, leading to the transcription of genes. In response to this activation, by stimulating ROS production, it is possible to observe some photobiomodulatory effects in normal cells, such as collagen synthesis, the expression of anti-apoptotic proteins, the synthesis of proteins involved in gene expression, cell migration, and proliferation (Tergaonkar, 2006; Chen et al., 2011).
Opsins
Photosensitive proteins, known as opsins (OPN), are expressed in non-visual and visual tissues. Most animal opsins are coupled to the G protein (Gt, Gq, Go, Gs, Gi and Gi, and Gi/Go), and when energized by light, act as photoacceptors, triggering a series of signaling cascades mediated by G proteins (Koyanagi and Terakita, 2014). Thus, they can modulate cellular physiological responses via intentional light irradiation. Humans have nine opsin genes, all of which have different absorption maxima, four visual opsins, peropsin, RGR, OPN3, OPN4, and OPN5 (Terakita and Nagata, 2014). OPN3, also known as panopsin, is expressed in various tissues, such as the eye, liver, and brain. These opsins are more sensitive to green and blue light and activate cascades related to Go and Gi proteins (Koyanagi et al., 2013). The human retina expresses OPN4, also known as melanopsin, which has maximum absorption in the range of blue light and activates cascades mediated by the G-type proteins Gq and Gi/Go (Bailes and Lucas, 2013). OPN5, known as neuropsin, is highly sensitized by the UV region and activates signaling cascades through the Gi protein (Kojima et al., 2011).
One of the episodes that follows opsin energization and activation is the opening of photosensitive ion channels, such as transient receptor potential (TRP) channels (Poletini et al., 2015), which allows for the non-selective permeabilization of sodium, calcium, and magnesium in the cell (Montell, 2011). Lan et al. (2020) demonstrated that OPN3 expression increases in human dermal fibroblasts after exposure to ultraviolet A radiation. This regulation of OPN3 triggers phototransduction and increases the expression of matrix metalloproteinases (MMPs) through the calcium-dependent G protein-coupled signaling pathway related to skin photoaging. Castellano-Pellicena et al. (2019) demonstrated that blue light irradiation at 447 nm increased the expression of OPN3 in the tongue epithelium and accelerated tissue healing. Through differentiation, proliferation, and migration assays, they found that blue light promotes keratinocyte differentiation. Wang et al. (2020a) observed that in human epidermal melanocytes in vitro, the silencing of OPN3 by RNAi-OPN3 decreased intracellular calcium levels, leading to the activation of the mitochondrial apoptosis pathway.
Phototoxicity
The biological effects triggered by phototherapy depend on the standard device, which varies according to the irradiation target (Zein et al., 2018). The cytotoxicity promoted by photobiomodulation has also been found to improve the therapeutic efficiency, mainly for the treatment of tumors (Ohara et al., 2003; Oh et al., 2015; Kim et al., 2021; Tian et al., 2021). The known cell apoptosis pathways are observed in intrinsic (mitochondrial) and extrinsic (activation of cell death receptors) processes (Cavalcante et al., 2019).
Masub et al. (2021) demonstrated that LED irradiation at 633 nm, high fluence at 640 J/cm2, and irradiance at 87 mW/cm2 in human dermal fibroblasts negatively influenced cell cycle progression and induced cell cycle arrest in G0/G1. This finding was followed by an increased expression of the p53 checkpoint regulator.
Regarding the wavelength of light for phototherapy procedures, Oh et al. (2015) demonstrated that blue LED (450 nm) has the potential to inhibit cell growth and trigger apoptosis in melanoma cells. The first proposed mechanism was similar to that reported for red light at high fluences. Elevated ROS levels were found to lead to caspase activation and mitochondrial damage. In addition, their experimental results indicated an increase and activation of the transcription factor p53 due to DNA damage. Increased P53 levels initiate cell cycle arrest and trigger apoptosis via the transcriptional activation of pro-apoptotic genes (Fridman and Lowe, 2003). Wang et al. (2017) compared the effects of higher (660 and 810 nm) and lower (415 and 540 nm) wavelengths on stem cells. In this study, green and blue light decreased cellular ATP, inhibited cell proliferation, increased intracellular ROS and calcium, and decreased intracellular pH and mitochondrial membrane potential. The results indicated an increase in the activity of TRPV1 calcium channels caused by the absorption of blue and green light by OPNs, which have a signaling relationship with these cationic channels. Thus, the authors concluded that due to the energization of opsins, the increase in calcium and ROS levels was the main factor responsible for inhibiting proliferation. Kim et al. (2021) demonstrated the apoptotic potential of a blue LED (460 nm) in a pancreatic tumor, promoted by the regulation of the AKT/mTOR pathway (an important pathway related to the progression of tumors) and its suppressive potential in a tumor model in xenografts through the inhibition of the AKT2 protein. Protein kinase B (AKT) isoforms are overexpressed in cancers, mediate signs of survival, promote tumor cell proliferation, and cause resistance to chemotherapy.
Radiation emitted from light-emiting diodes
Table 1 presents the findings of some recent studies on LED-induced photobiomodulation in different models with varying irradiation parameters. Studies were found on the databases of scientific articles SciElo - Scientific Electronic Library Online (http://www.scielo.br), PubMed (https://pubmed.ncbi.nlm.nih.gov), CAPES - Coordenação de Aperfeiçoamento de Pessoal de Nível Superior (http://www.periodicos.capes.gov.br), Google Scholar (https://scholar.google.com), and ERIC - Educational Resources Information Center (https://eric.ed.gov). To this end, the following keywords were used in the advanced search fields of the aforementioned databases: “light-emitting diodes,” “photobiomodulation,”” phototherapy,” “LED therapy,” “blue LED,” “green LED,” “red LED,” and “NIR-LED.” The search was limited to articles published between 2009 and 2021, from which a rigorous analysis of the resulting articles was conducted. Articles that addressed the mechanisms involved in the response to LED therapy and photobiomodulation itself were selected, the main findings of which are discussed below.
Based on the studies presented in Table 1, some characteristics of photobiomodulation induced by LED irradiation, which have already been clinically explored, were observed, such as increased proliferation and viability in different cell lines and improved repair of different tissues, increased angiogenesis, altered metabolism, and cellular morphology, for wavelengths between red and NIR, in previously established parameters.
Yang and co-workers demonstrated that red LED photobiomodulation positively affects the osteogenic proliferation and differentiation of mesenchymal stem cells. They cited the MAPK/ERK pathway and target cellular pathways of ROS, ATP, NADH, and cAMP as pathways potentially responsible for the observed effects but did not explore the pathways themselves in their study (Yang et al., 2016). Choi and co-workers observed changes in the viability and morphology of human fibroblasts after red LED irradiation, mediated by increased levels of heat shock proteins (HSPs), providing a potential explanation for the improvement in the healing and repair of wounds after irradiation with LED (Choi et al., 2019). In 2020, another study demonstrated the tissue repair potential of red LED in an experimental rat model of Achilles tendonitis. The authors observed an increase in the number of fibroblasts, as well as in the amount of collagen and the expression of HSP70 (Evangelista et al., 2020).
In addition, within the same spectral range, the modulation of the inflammatory response, analgesia, the reduction of neuropathic symptoms, and the modulation of neural oscillations were observed. Tatmatsu-Rocha and co-workers analyzed the effects of photobiomodulation mediated by laser and near-infrared LED on collagen fibers in an animal model. In their study, although no increase in collagen fibers was observed in groups treated with LED, irradiation was found to positively modulate angiogenesis and increase vascular endothelial growth factor (VEGF). They also observed a reduction in COX2 expression, which exerts an anti-inflammatory effect (Tatmatsu-Rocha et al., 2018). In 2016, another study explored the modulation of the inflammatory response by NIR-LED in rats, and reported reduced levels of TNF-α and IL-6, a reduced number of pro-inflammatory cells, and increased IL-10 expression (Helrigle et al., 2016). Two other studies analyzed the analgesic potential of red LED and NIR during childbirth and in a plantar incision model in rats, the latter by activating peripheral opioid receptors via L-arginine/NO (Cidral-Filho et al., 2014; Traverzim et al., 2021). Furthermore, in a 2019, a study demonstrated that NIR-LED-mediated transcranial photobiomodulation showed potential in modulating neural oscillations (Zomorrodi et al., 2019).
These responses are sometimes related to the activation of different proteins and molecular pathways that are already known. However, other times, researchers have been unable to accurately clarify the events behind the responses. Furthermore, it was observed that inhibitory effects could also be obtained by modulating the irradiation parameters in the same spectral range, such as the use of a high fluence. This was demonstrated by Meng and co-workers, who analyzed the response of human synoviocyte MH7A cells to red LED irradiation. Repeated radiation at 26 and 39 J/cm2 exerted an inhibitory effect on these cells, reducing proliferation and migration, potentially through the regulation of the TRPV4/PI3K/AKT/mTOR signaling pathway (Meng et al., 2020). However, inhibitory/cytotoxic effects are more commonly observed at wavelengths belonging to the irradiation range of blue and green light. The effects of inhibition of proliferation, differentiation, and cell migration, the reduction of metabolism and viability, the induction of cell damage and cell death, and the reduction of bacterial biofilm are presented in Table 1.
Shakibaie and co-workers demonstrated that blue LED irradiation at 17.5 J/cm2 decreased the viability of breast cancer cells. LDHA (Lactate dehydrogenase A) and GLS (Glutaminase) expression was found to be downregulated, and the consumption of glutamine, glucose, and lactate decreased. The opposite effects were observed in cells treated with red LED (Shakibaie et al., 2019). In 2020, another study demonstrated that exposing murine photoreceptor-derived cells to blue LED light caused increased levels of damaged lysosomes via the TFEB pathway and the formation of perinuclear clumps, promoting lysosomal cell death (Otsu et al., 2020). Yan and co-workers analyzed the effects of blue LED radiation on colorectal cancer cells and observed a decrease in live cells, lower cell proliferation, and increased apoptosis. Cell migration was inhibited, similar to epithelial-mesenchymal transition (EMT), and an accumulation of ROS and DNA damage were also observed. However, in addition to the results mentioned above, responses contrary to those commonly observed were also observed, such as increased migration, cell proliferation, differentiation, the induction of bone regeneration, increased angiogenesis, and reduced cell necrosis (Yan et al., 2018). Rohringer and co-workers investigated the effects of LED radiation on endothelial cells in vitro. As a result, the authors found that pulsed green light significantly increased cell proliferation and 2D and 3D migration, even more than those observed with red light treatment; however, they did not specify the pathways by which these effects were achieved (Rohringer et al., 2017). In addition, in 2016, a study reported that blue LED could significantly increase bone regeneration in rats (Dereci et al., 2016).
Few studies have addressed photobiomodulation at molecular and metabolic levels. Generally, researchers have investigated responses to LED radiation in a superficial way, only hypothesizing the mechanisms behind the responses obtained, as these are not yet well understood. As such, there is a need for future studies to approach the metabolic and molecular alterations in a qualitative and quantitative manner, using newly available technologies, such as omics technologies, highlighting NMR-based metabolomics. In this way, it may be possible to identify the changes caused by LED therapy, such as the inhibition, stimulation, and/or metabolic shifts, and to understand more clearly how the effects of LED therapy are achieved to enable its safe application, as well as for the development of new therapies.
Metabolic effects of light-emiting diodes therapy
Studying the metabolic shift associated with low-power irradiation is a promising way to clarify LED-induced photobiomodulation. Although metabolomics, the systematic study of metabolites on a large scale, plays a fundamental role in this investigation, studies on LED-induced photobiomodulation from this perspective are scarce. The metabolic and molecular effects of each wavelength are summarized in Figure 1.
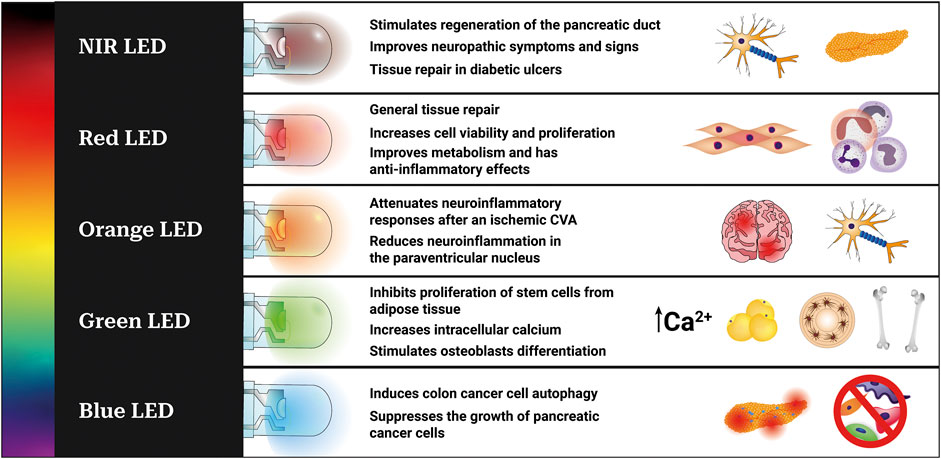
FIGURE 1. Metabolic and molecular effects observed after irradiation with LED in different experimental models and wavelengths. Blue LED (450–495 nm) (Yoshimoto et al., 2018; Kim et al., 2021), green LED (495–570 nm) (Wang et al., 2016; Rohringer et al., 2017; Wang et al., 2017), yellow LED (570–590 nm), orange LED (590–620 nm) (Lee et al., 2017; Wang et al., 2020b), red LED (620–700 nm) (Yang et al., 2016; Brochetti et al., 2017; de Almeida et al., 2017; Shakibaie et al., 2019; Martignago et al., 2020; Traverzim et al., 2021), and NIR-LED (700–2,500 nm) (de Mattos et al., 2014; Tatmatsu-Rocha et al., 2017; Vitoriano et al., 2019).
Shakibaie et al., (2019) investigated the effects of distinct types of radiation on the metabolism of breast cancer cells. As a result, blue LED radiation (435 nm) was found to decrease the viability and expression of glutaminase (GLS) and lactate dehydrogenase A (LDHA) in MCF7 cells compared to those in the group irradiated with red light and the control group. Thus, a decline in glutamine, glucose, and lactate consumption was observed. Blue light irradiation was also found to inhibit cell proliferation and metabolic activation. In contrast to the effect of irradiation with blue LED, red LED was found to positively regulate the expression of LDHA and GLS, followed by a decline in glucose consumption (112%), glutamine, and lactate production (107%). These results indicate increased metabolic activity in tumor cells irradiated with red LED. Zhang et al., (2019) demonstrated the influence of light irradiation on non-visual physiological processes. To this end, rats were exposed to light emitted from LEDs at different wavelengths. The group irradiated with green light showed higher serum and liver lipid levels and greater glucose intolerance than the group exposed to white light. In addition, the expression of melatonin 1b and thyroid hormone β receptors was found to be reduced in the livers of obese rats exposed to green light.
In this review, data on the cellular metabolic pathways altered by the absorption of light emitted from LEDs was gathered for the first time. Despite advances, research that provides insights into cellular responses to irradiation at different wavelengths remains scarce. The gaps in our understanding of LED-induced photobiomodulation and the lack of studies on metabolomic processes underlie the urgent need for more studies. In this context, the analytical techniques of nuclear magnetic resonance (NMR), as well as mass spectrometry (MS), are being increasingly considered as promising tools for the metabolomic analysis of different experimental models (Costa dos Santos et al., 2021), thereby improving our understanding the biological effects of LEDs and their potential therapeutic applications in photobiomodulation.
Future perspectives
The emergence of the omics era has paved the way for medicine to focus on individual genetic and/or metabolic backgrounds and precision medicine. Currently, it is possible to combine omics data with parameters of LED therapy in different cell lines and tissue types with molecular and metabolic profiles, leading to precision LED therapy. Treatment could be personalized based on the target tissue type and patient metabolic profile to enable a more effective and safer treatment, as well as to address the specific needs of each patient individually.
Conclusion
The studies reviewed herein suggest that radiation emitted from low-power LEDs can induce photobiomodulation depending on the irradiation parameters and biological tissue, as shown in Figure 1 and Table 1. The mechanisms involved in this effect are mainly related to the energization of endogenous photoacceptors, such as cytochrome c oxidase. These results include metabolism modulation, viability, the proliferation of normal and tumoral cells, tissue regeneration, an alteration in the differentiation and migration of different cell types, and the induction of cellular damage. This review is important as it highlights the current lack of metabolomics studies with photobiomodulation, as well as providing an organized and comprehensive overview of the mechanisms responsible for the performance of LEDT for use by the scientific community, in addition to future perspectives of precision LED therapy.
Author contributions
GS conceived the study, organized the topics, and revised the manuscript. AF organized the topics and revised the manuscript. TV wrote the manuscript and contributed to discussions. All the authors have read this article before submission.
Funding
We received funding from Fundação de Amparo à Pesquisa do Estado do Rio de Janeiro (FAPERJ) processes E-26/200.597/2020, and E-26/010.001958/2019, Edital No. 2019/2—scholarship, JCNE process E-26/201.259/2021, and CNE process E-26/201.089/2022.- IC scholarship—E/26/200.597/2020, and JCNE SEI-26003/004746/2021
Acknowledgments
Special thanks to the Fundação de Amparo à Pesquisa do Estado do Rio de Janeiro (FAPERJ) (processes E-26/200.597/2020 and E-26/010.001958/2019), Edital No. 2019/2 (scholarship, JCNE process E-26/201.259/2021, and CNE process E-26/201.089/2022 for their support.
Conflict of interest
The authors declare that the research was conducted in the absence of any commercial or financial relationships that could be construed as a potential conflict of interest.
Publisher’s note
All claims expressed in this article are solely those of the authors and do not necessarily represent those of their affiliated organizations, or those of the publisher, the editors and the reviewers. Any product that may be evaluated in this article, or claim that may be made by its manufacturer, is not guaranteed or endorsed by the publisher.
References
Albuquerque-Pontes, G. M., Vieira, R. de P., Tomazoni, S. S., Caires, C. O., Nemeth, V., Vanin, A. A., et al. (2015). Effect of pre-irradiation with different doses, wavelengths, and application intervals of low-level laser therapy on cytochrome c oxidase activity in intact skeletal muscle of rats. Lasers Med. Sci. 30 (1), 59–66. doi:10.1007/s10103-014-1616-2
Amaroli, A., Ravera, S., Baldini, F., Benedicenti, S., Panfoli, I., and Vergani, L. (2019). Photobiomodulation with 808-nm diode laser light promotes wound healing of human endothelial cells through increased reactive oxygen species production stimulating mitochondrial oxidative phosphorylation. Lasers Med. Sci. 34 (3), 495–504. doi:10.1007/s10103-018-2623-5
Antunes, F., Boveris, A., and Cadenas, E. (2007). On the biologic role of the reaction of NO with oxidized cytochrome c oxidase. Antioxid. Redox Signal. 9 (10), 1569–1580. doi:10.1089/ars.2007.1677
Bailes, H. J., and Lucas, R. J. (2013). Human melanopsin forms a pigment maximally sensitive to blue light (λmax ≈ 479 nm) supporting activation of G(q/11) and G(i/o) signalling cascades. Proc. R. Soc. B 280 (1759), 20122987. doi:10.1098/rspb.2012.2987
Beauvoit, B., Kitai, T., and Chance, B. (1994). Contribution of the mitochondrial compartment to the optical properties of the rat liver: A theoretical and practical approach. Biophys. J. 67 (6), 2501–2510. doi:10.1016/S0006-3495(94)80740-4
Bernardi, P., Krauskopf, A., Basso, E., Petronilli, V., Blalchy-Dyson, E., Lisa, F. D., et al. (2099). The mitochondrial permeability transition from in vitro artifact to disease target. FEBS J. 273 (10), 2077–2099. doi:10.1111/j.1742-4658.2006.05213.x
Brochetti, R. A., Leal, M. P., Rodrigues, R., da Palma, R. K., de Oliveira, L. V. F., Horliana, A. C. R. T., et al. (2017). Photobiomodulation therapy improves both inflammatory and fibrotic parameters in experimental model of lung fibrosis in mice. Lasers Med. Sci. 32 (8), 1825–1834. doi:10.1007/s10103-017-2281-z
Brown, G. C. (2001). Regulation of mitochondrial respiration by nitric oxide inhibition of cytochrome c oxidase. Biochimica Biophysica Acta - Bioenergetics 1504 (1), 46–57. doi:10.1016/S0005-2728(00)00238-3
Burnstock, G. (2018). Purine and purinergic receptors. Brain Neurosci. Adv. 2, 239821281881749–10. doi:10.1177/2398212818817494
Cadet, J., and Wagner, J. R. (2013). DNA base damage by reactive oxygen species, oxidizing agents, and UV radiation. Cold Spring Harb. Perspect. Biol. 5 (2), a012559. doi:10.1101/cshperspect.a012559
Castellano‐Pellicena, I., Uzunbajakava, N. E., Mignon, C., Raafs, B., Botchkarev, V. A., and Thornton, M. J. (2019). Does blue light restore human epidermal barrier function via activation of Opsin during cutaneous wound healing? Lasers Surg. Med. 51 (4), 370–382. doi:10.1002/lsm.23015
Cavalcante, G. C., Schaan, A. P., Cabral, G. F., Santana-da-Silva, M. N., Pinto, P., Vidal, A. F., et al. (2019). A cell’s fate: An overview of the molecular biology and genetics of apoptosis. Int. J. Mol. Sci. 20 (17), 4133. doi:10.3390/ijms20174133
Chen, A. C. H., Arany, P. R., Huang, Y-Y., Tomkinson, E. M., Sharmal, S. K., Kharkwal, G. B., et al. (2011). Low-Level laser therapy activates NF-kB via generation of reactive oxygen species in mouse embryonic fibroblasts. PLoS One 6 (7), e22453–8. doi:10.1371/journal.pone.0022453
Choi, S., Chang, S.-Y., Biswas, R., Chung, P.-S., Mo, S., Lee, M. Y., et al. (2019). Light-emitting diode irradiation using 660 nm promotes human fibroblast HSP90 expression and changes cellular activity and morphology. J. Biophot. 12 (9), e201900063. doi:10.1002/jbio.201900063
Cidral-Filho, F. J., Mazzardo-Martins, L., Martins, D. F., and Santos, A. R. S. (2014). Light-emitting diode therapy induces analgesia in a mouse model of postoperative pain through activation of peripheral opioid receptors and the L-arginine/nitric oxide pathway. Lasers Med. Sci. 29 (2), 695–702. doi:10.1007/s10103-013-1385-3
Costa dos Santos, G., Renovato-Martins, M., and de Brito, N. M. (2021). The remodel of the “central dogma”: A metabolomics interaction perspective. Metabolomics 17 (5), 48. doi:10.1007/s11306-021-01800-8
Dall Agnol, M. A., Nicolau, R. A., de Lima, C. J., and Munin, E. (2009). Comparative analysis of coherent light action (laser) versus non-coherent light (light-emitting diode) for tissue repair in diabetic rats. Lasers Med. Sci. 24 (6), 909–16. doi:10.1007/s10103-009-0648-5
D’Angio, C. T., and Finkelstein, J. N. (2000). Oxygen regulation of gene expression: A study in opposites. Mol. Genet. Metab. 71 (1–2), 371–380. doi:10.1006/mgme.2000.3074
de Almeida, L. de F. D., Basso, F. G., Turrioni, A. P. S., De-Souza-Costa, C. A., and Hebling, J., (2017), Metabolism of Odontoblast-like cells submitted to transdentinal irradiation with blue and red LED, Arch. Oral Biol., vol. 83, 258–264. doi:10.1016/j.archoralbio.2017.08.004
de Mattos, L. H. L., Álvarez, L. E. C., Yamada, A. L. M., Hussni, C. A., Rodrigues, C. A., Watanabe, M. J., et al. (2014). Effect of phototherapy with light-emitting diodes (890 nm) on tendon repair: An experimental model in sheep. Lasers Med. Sci. 30 (1), 193–201. doi:10.1007/s10103-014-1641-1
de Paula-Silva, M., Broering, M. F., Scharf, P., da Rocha, G. H. O., Farsky, S., and Lino-Dos-Santos-Franco, A. (2019). Red light-emitting diode treatment improves tissue recovery in DSS-induced colitis in mice. J. Photochem. Photobiol. B Biol. 212, 112018. doi:10.1016/j.jphotobiol.2020.112018
Dereci, Ö., Sindel, A., Serap Toru, H., Yüce, E., Ay, S., and Tozoğlu, S. (2016). The comparison of the efficacy of blue light-emitting diode light and 980-nm low-level laser light on bone regeneration. J. Craniofac. Surg. 27 (8), 2185–2189, Nov. doi:10.1097/SCS.0000000000003068
Desagher, S., and Martinou, J. C. (2000). Mitochondria as the central control point of apoptosis. Trends Cell Biol. 10 (9), 369–77. doi:10.1016/s0962-8924(00)01803-1
Di Meo, S., Reed, T. T., Venditti, P., and Victor, V. M. (2018). Harmful and beneficial role of ROS 2017. Oxid. Med. Cell. Longev. 2018, 1–2. doi:10.1155/2018/5943635
Diamantino, A. G., Nicolau, R. A., Costa, D. R., Almeida, A. P. de B., Mato, D. X. de M., de Oliveira, M. A., et al. (2017). Effect of non-coherent infrared light (LED, λ945 ± 20 nm) on bone repair in diabetic rats—Morphometric and spectral analyses. Lasers Med. Sci. 32 (5), 1041–1049. doi:10.1007/s10103-017-2205-y
Dungel, P., Hartinger, J., Chaudary, S., Slezak, P., Hofmann, A., Hausner, T., et al. (2014). Low level light therapy by LED of different wavelength induces angiogenesis and improves ischemic wound healing. Lasers Surg. Med. 46 (10), 773–780. doi:10.1002/lsm.22299
Dungel, P., Mittermavr, R., Haindl, S., Osipov, A., Wagner, C., Redl, H., et al. (2008). Illumination with blue light reactivates respiratory activity of mitochondria inhibited by nitric oxide, but not by glycerol trinitrate. Arch. Biochem. Biophys. 471 (2), 109–115. doi:10.1016/j.abb.2008.01.009
Evangelista, A. N., dos Santos, F. F., Martins, L. P. de O., Gaiad, T. P., Machado, A. S. D., Rocha-Vieira, E., et al. (2020). Photobiomodulation therapy on expression of HSP70 protein and tissue repair in experimental acute Achilles tendinitis. Lasers Med. Sci. 36, 1201–1208. doi:10.1007/s10103-020-03155-3
Forte, M., and Bernardi, P. (2006). The permeability transition and BCL-2 family proteins in apoptosis: Co-conspirators or independent agents? Cell Death Differ. 13 (8), 1287–1290. doi:10.1038/sj.cdd.4401957
Fridman, J. S., and Lowe, S. W. (2003). Control of apoptosis by p53. Oncogene 22 (56), 9030–9040. doi:10.1038/sj.onc.1207116
Gomes, R. F. dos S. (2011). Optimização da focagem de feixes de laser em tecidos biológicos: Desenvolvimento de um simulador óptico. Lisbon, Portugal: Universidade Nova de Lisboa.
Hayworth, C. R., Rojas, J. C., Padilla, E., Holmes, G. M., Sheridan, E. C., and Gonzalez-Lima, F. (2010). In vivo low-level light therapy increases cytochrome oxidase in skeletal muscle. Photochem. Photobiol. 86 (3), 673–680. doi:10.1111/j.1751-1097.2010.00732.x
Helrigle, C., de Carvalho, P. de T. C., Casalechi, H. L., Leal-Junior, E. C. P., Fernandes, G. H. C., Helrigel, P. A., et al. (2016). Effects of low-intensity non-coherent light therapy on the inflammatory process in the calcaneal tendon of ovariectomized rats. Lasers Med. Sci. 31 (1), 33–40. doi:10.1007/s10103-015-1821-7
Holonyak, N., and Bevacqua, S. F. (1962). COHERENT (VISIBLE) LIGHT EMISSION FROM Ga(As1−xPx) JUNCTIONS. Appl. Phys. Lett. 1 (4), 82–83. doi:10.1063/1.1753706
Huang, Y.-Y., Chen, A. C. H., Carroll, J. D., and Hamblin, M. R. (2009). Biphasic dose response in low level light therapy. Dose-Response 7 (4), 358–383. doi:10.2203/dose-response.09-027
Jacobson, J., and Duchen, M. R. (2002). Mitochondrial oxidative stress and cell death in astrocytes--requirement for stored Ca2+ and sustained opening of the permeability transition pore. J. Cell Sci. 115 (6), 1175–1188. doi:10.1242/jcs.115.6.1175
Karu, T. (2010). Mitochondrial mechanisms of photobiomodulation in context of new data about multiple roles of ATP. Photomed. Laser Surg. 28 (2), 159–160. doi:10.1089/pho.2010.2789
Karu, T. (1987). Photobiological fundamentals of low-power laser therapy. IEEE J. Quantum Electron. 23 (10), 1703–1717. doi:10.1109/JQE.1987.1073236
Karu, T. (1999). Primary and secondary mechanisms of action of visible to near-IR radiation on cells. J. Photochem. Photobiol. B Biol. 49 (1), 1–17. doi:10.1016/S1011-1344(98)00219-X
Khakh, B. S., and Burnstock, G. (2009). The double life of ATP. Sci. Am. 301 (6), 84–92. doi:10.1038/scientificamerican1209-84
Kim, J. S., He, L., and Lemasters, J. J. (2003). Mitochondrial permeability transition: A common pathway to necrosis and apoptosis. Biochem. Biophys. Res. Commun. 304 (3), 463–470. doi:10.1016/S0006-291X(03)00618-1
Kim, Y. M., Ko, S. H., Shin, Y. I., Kim, Y., Kim, T., Jung, J., et al. (2021). Light‐emitting diode irradiation induces AKT/mTOR-mediated apoptosis in human pancreatic cancer cells and xenograft mouse model. J. Cell. Physiol. 236 (2), 1362–1374. doi:10.1002/jcp.29943
Kinnally, K. W., and Antonsson, B. (2007). A tale of two mitochondrial channels, MAC and PTP, in apoptosis. Apoptosis 12 (5), 857–68. doi:10.1007/s10495-007-0722-z
Kojima, D., Mori, S., Torii, M., Wada, A., Morishita, R., and Fukada, Y. (2011). UV-sensitive photoreceptor protein OPN5 in humans and mice. PLoS One 6 (10), e26388. doi:10.1371/journal.pone.0026388
Korge, P., Yang, L., Yang, J.-H., Wang, Y., Qu, Z., and Weiss, J. N. (2011). Protective role of transient pore openings in calcium handling by cardiac mitochondria. J. Biol. Chem. 286 (40), 34851–34857. doi:10.1074/jbc.M111.239921
Koyanagi, M., Takada, E., Nagata, T., Tsukamoto, H., and Terakita, A. (2013). Homologs of vertebrate Opn3 potentially serve as a light sensor in nonphotoreceptive tissue. Proc. Natl. Acad. Sci. U. S. A. 110 (13), 4998–5003. doi:10.1073/pnas.1219416110
Koyanagi, M., and Terakita, A. (2014). Diversity of animal opsin-based pigments and their optogenetic potential. Biochimica Biophysica Acta - Bioenergetics 1837 (5), 710–716. doi:10.1016/j.bbabio.2013.09.003
Kuse, Y., Ogawa, K., Tsuruma, K., Shimazawa, M., and Hara, H. (2014). Damage of photoreceptor-derived cells in culture induced by light emitting diode-derived blue light. Sci. Rep. 4 (1), 5223. doi:10.1038/srep05223
Lan, Y., Wang, Y., and Lu, H. (2020). Opsin 3 is a key regulator of ultraviolet A-induced photoageing in human dermal fibroblast cells. Br. J. Dermatol. 182 (5), 1228–1244. doi:10.1111/bjd.18410
Lee, H. I., Lee, S.-W., Kim, N. G., Park, K.-J., Choi, B. T., Shin, Y.-I., et al. (2017). Low-level light emitting diode (LED) therapy suppresses inflammasome-mediated brain damage in experimental ischemic stroke. J. Biophot. 10 (11), 1502–1513. doi:10.1002/jbio.201600244
Li, W.-H., Fassih, A., Binner, C., Parsa, R., and Southall, M. D. (2018). Low-level red LED light inhibits hyperkeratinization and inflammation induced by unsaturated fatty acid in an in vitro model mimicking acne. Lasers Surg. Med. 50 (2), 158–165. doi:10.1002/lsm.22747
Martignago, C. C. S., Tim, C. R., Assis, L., Silva1, V. R., Santos, E. C. B., Vieira, F. N., et al. (2020). Effects of red and near-infrared LED light therapy on full-thickness skin graft in rats. Lasers Med. Sci. 35 (1), 157–164. doi:10.1007/s10103-019-02812-6
Masub, N., Austin, E., Huang, A., and Jagdeo, J. (2021). High-fluence light emitting diode-red light inhibits cell cycle progression in human dermal fibroblasts. J. Biophot. 14 (2), e202000359–7. doi:10.1002/jbio.202000359
Matsumoto, N., Yoshikawa, K., Shimada, M., Kurita, N., Sato, H., Iwata, T., et al. (2014). Effect of light irradiation by light emitting diode on colon cancer cells. Anticancer Res. 34 (9), 4709–4716. [Online]. Available: http://www.ncbi.nlm.nih.gov/pubmed/25202048.
McGuff, P. E., Deterling, R. A., and Gottlieb, L. S. (1965). Tumoricidal effect of laser energy on experimental and human malignant tumors. N. Engl. J. Med. 273 (9), 490–2. doi:10.1056/NEJM196508262730906
Meng, C., Xia1, Q., Wu1, H., Huang, H., Liu, H., Li, Y., et al. (2020). Photobiomodulation with 630-nm LED radiation inhibits the proliferation of human synoviocyte MH7A cells possibly via TRPV4/PI3K/AKT/mTOR signaling pathway. Lasers Med. Sci. 35 (9), 1927–1936. doi:10.1007/s10103-020-02977-5
Mester, E., Mester, A. F., and Mester, A. (1985). The biomedical effects of laser application. Lasers Surg. Med. 5 (1), 31–39. doi:10.1002/lsm.1900050105
Mester, E., Spiry, T., Szende, B., and Tota, J. G. (1971). Effect of laser rays on wound healing. Am. J. Surg. 32 (2), 532–535. doi:10.1016/0002-9610(71)90482-X
Mester, E., Szende, B., and Gärtner, P. (1968). The effect of laser beams on the growth of hair in mice. Radiobiol. Radiother. 9 (5), 621–6. [Online]. Available: http://www.ncbi.nlm.nih.gov/pubmed/5732466.
Montell, C. (2011). The history of TRP channels, a commentary and reflection. Pflügers Arch. - Eur. J. Physiol. 461 (5), 499–506. doi:10.1007/s00424-010-0920-3
Nasa.gov [homepage on the internet] (2009). NASA’s john F. Kennedy space center. Available from: https://www.nasa.gov/centers/kennedy/home/plant_growth.html (Last Updated SepFeb 1604, 20122022).
Nishioka, M. A., Pinfildi, C. E., Sheliga, T. R., Arias, V. E., Gomes, H. C., and Ferreira, L. M. (2012). LED (660 nm) and laser (670 nm) use on skin flap viability: Angiogenesis and mast cells on transition line. Lasers Med. Sci. 27 (5), 1045–1050. doi:10.1007/s10103-011-1042-7
Oh, P.-S., Na, K. S., Hwang, H., Jeong, H.-S., Lim, S., Sohn, M.-H., et al. (2015). Effect of blue light emitting diodes on melanoma cells: Involvement of apoptotic signaling. J. Photochem. Photobiol. B Biol. 142, 197–203. doi:10.1016/j.jphotobiol.2014.12.006
Ohara, M., Kawashima, Y., Kitajima, S., Mitsuoka, C., and Watanabe, H. (2003). Blue light inhibits the growth of skin tumors in the v-Ha-ras transgenic mouse. Cancer Sci. 94 (2), 205–209. doi:10.1111/j.1349-7006.2003.tb01420.x
Otsu, W., Ishida, K., Nakamura, S., Shimazawa, M., Tsusaki, H., and Hara, H. (2020). Blue light-emitting diode irradiation promotes transcription factor EB-mediated lysosome biogenesis and lysosomal cell death in murine photoreceptor-derived cells. Biochem. Biophys. Res. Commun. 526 (2), 479–484. doi:10.1016/j.bbrc.2020.03.118
Pires, B., Silva, R., Ferreira, G., and Abdelhay, E. (2018). NF-kappaB: Two sides of the same coin. Genes. (Basel) 9(1), 24. doi:10.3390/genes9010024
Poletini, M. O., Moraes, M. N., Ramos, B. C., Jerônimo, R., and Castrucci, A. M. de L. (2015). TRP channels: A missing bond in the entrainment mechanism of peripheral clocks throughout evolution. Temperature 2 (4), 522–534. doi:10.1080/23328940.2015.1115803
Rich, P. R. (2017). Mitochondrial cytochrome c oxidase: Catalysis, coupling and controversies. Biochem. Soc. Trans. 45 (3), 813–829. doi:10.1042/BST20160139
Rohringer, S., Holnthoner, W., Chaudary, S., Slezak, P., Priglinger, E., Strass, M., et al. (2017). The impact of wavelengths of LED light-therapy on endothelial cells. Sci. Rep. 7 (1), 10700. doi:10.1038/s41598-017-11061-y
Rosa, L. P., da Silva, F. C., Viana, M. S., and Meira, G. A. (2016). In vitro effectiveness of 455-nm blue LED to reduce the load of Staphylococcus aureus and Candida albicans biofilms in compact bone tissue. Lasers Med. Sci. 31 (1), 27–32. doi:10.1007/s10103-015-1826-2
Ruan, Y., Kato, H., Taguchi, Y., Yamauchi, N., and Umeda, M. (2020). Irradiation by high-intensity red light-emitting diode enhances human bone marrow mesenchymal stem cells osteogenic differentiation and mineralization through Wnt/β-catenin signaling pathway. Lasers Med. Sci. 36 (1), 55–65. doi:10.1007/s10103-020-03002-5
Salehpour, F., Mahmoudi, J., Kamari, F., Sadigh-Eteghad, S., Rasta, S. H., and Hamblin, M. R. (2018). Brain photobiomodulation therapy: A narrative review. Mol. Neurobiol. 55 (8), 6601–6636. doi:10.1007/s12035-017-0852-4
Schreck, R., Rieber, P., and Baeuerle, P. A. (1991). Reactive oxygen intermediates as apparently widely used messengers in the activation of the NF-kappa B transcription factor and HIV-1. EMBO J. 10 (8), 2247–2258. doi:10.1002/j.1460-2075.1991.tb07761.x
Shakibaie, M., Vaezjalali, M., Rafii-Tabar, H., and Sasanpour, P. (2019). Phototherapy alters the oncogenic metabolic activity of breast cancer cells. Photodiagnosis Photodyn. Ther. 30, 101695. doi:10.1016/j.pdpdt.2020.101695
Silveira, P. C. L., Ferreira, K. B., da Rocha, F. R., Pieri, B. L. S., Pedroso, G. S., de Souza, C. T., et al. (2016). Effect of low-power laser (LPL) and light-emitting diode (LED) on inflammatory response in burn wound healing. Inflammation 39 (4), 1395–404. doi:10.1007/s10753-016-0371-x
Spinoff.nasa.gov [homepage on the internet] (2008). NASA technology transfer program. Available at: https://spinoff.nasa.gov/Spinoff2008/hm_3.html (Cited Feb 04, 2022).
Tatmatsu-Rocha, J. C., de Castro, C. A., Sene-Fiorese, M., and Parizotto, N. A. (2017). Light-emitting diode modulates carbohydrate metabolism by pancreatic duct regeneration. Lasers Med. Sci. 32 (8), 1747–1755. doi:10.1007/s10103-017-2245-3
Tatmatsu-Rocha, J. C., Tim, C. R., Avo, L., Bernardes-Filho, R., Brassolatti, P., Kido, H. W., et al. (2018). Mitochondrial dynamics (fission and fusion) and collagen production in a rat model of diabetic wound healing treated by photobiomodulation: Comparison of 904 nm laser and 850 nm light-emitting diode (LED). J. Photochem. Photobiol. B Biol. 187, 41–47. doi:10.1016/j.jphotobiol.2018.07.032
Terakita, A., and Nagata, T. (2014). Functional properties of opsins and their contribution to light-sensing physiology. Zool. Sci. 31 (10), 653–9. doi:10.2108/zs140094
Tergaonkar, V. (2006). NFκB pathway: A good signaling paradigm and therapeutic target. Int. J. Biochem. Cell Biol. 38 (10), 1647–1653. doi:10.1016/j.biocel.2006.03.023
Teuschl, A., Balmayor, E. R., Redl, H., van Griensven, M., and Dungel, P. (2015). Phototherapy with LED light modulates healing processes in an in vitro scratch-wound model using 3 different cell types. Dermatol. Surg. 41 (2), 261–268. doi:10.1097/DSS.0000000000000266
Tian, Y., Kim, H., and Kang, H. W. (2021). In vitro anti-tumor effect of high-fluence low-power laser light on apoptosis of human colorectal cancer cells. Lasers Med. Sci. 36 (3), 513–520. doi:10.1007/s10103-020-03050-x
Traverzim, M. A., Sobral, A. P. T., Fernandes, K. P. S., Silva, D. de F. T., Pavani, C., Mesquita-Ferrari, R. A., et al. (2021). The effect of photobiomodulation on analgesia during childbirth: A controlled and randomized clinical trial. Photobiomodul. Photomed. Laser Surg. 39 (4), 265–271. doi:10.1089/photob.2020.4976
Vitoriano, N. A. M., Alverne, D. G. B. M., Martins, M. I. S., Silva, P. S., Martins, C. A., Teixeira, H. D., et al. (2019). Comparative study on laser and LED influence on tissue repair and improvement of neuropathic symptoms during the treatment of diabetic ulcers. Lasers Med. Sci. 34 (7), 1365–1371. doi:10.1007/s10103-019-02724-5
Wang, S., Luo, Q., Chen, H., Huang, J., Li, X., Wu, L., et al. (2020). Light emitting diode therapy protects against myocardial ischemia/reperfusion injury through mitigating neuroinflammation. Oxid. Med. Cell. Longev. 2020, 1–8. doi:10.1155/2020/9343160
Wang, Y., Huang, Y.-Y., Wang, Y., Lyu, P., and Hamblin, M. R. (2016). Photobiomodulation (blue and green light) encourages osteoblastic-differentiation of human adipose-derived stem cells: Role of intracellular calcium and light-gated ion channels. Sci. Rep. 6 (1), 33719. doi:10.1038/srep33719
Wang, Y., Huang, Y.-Y., Wang, Y., Lyu, P., and Hamblin, M. R. (2017). Red (660 nm) or near-infrared (810 nm) photobiomodulation stimulates, while blue (415 nm), green (540 nm) light inhibits proliferation in human adipose-derived stem cells. Sci. Rep. 7 (1), 7781. doi:10.1038/s41598-017-07525-w
Wang, Y., Lan, Y., and Lu, H. (2020). Opsin3 downregulation induces apoptosis of human epidermal melanocytes via mitochondrial pathway. Photochem. Photobiol. 96 (1), 83–93. doi:10.1111/php.13178
Wu, S., Xing, D., Wang, F., Chen, T., and Chen, W. R. (2007). Mechanistic study of apoptosis induced by high-fluence low-power laser irradiation using fluorescence imaging techniques. J. Biomed. Opt. 12 (6), 064015. doi:10.1117/1.2804923
Wu, S., Zhou, F., Wei, Y., Chen, W. R., Chen, Q., and Xing, D. (2014). Cancer phototherapy via selective photoinactivation of respiratory chain oxidase to trigger a fatal superoxide anion burst. Antioxid. Redox Signal. 20 (5), 733–46. doi:10.1089/ars.2013.5229
Yan, G., Zhang, L., Feng, C., Gong, R., Idiiatullina, E., Huang, Q., et al. (2018). Blue light emitting diodes irradiation causes cell death in colorectal cancer by inducing ROS production and DNA damage. Int. J. Biochem. Cell Biol. 103, 81–88. doi:10.1016/j.biocel.2018.08.006
Yang, D., Yi, W., Wang, E., and Wang, M. (2016). Effects of light-emitting diode irradiation on the osteogenesis of human umbilical cord mesenchymal stem cells in vitro. Sci. Rep. 6 (1), 37370. doi:10.1038/srep37370
Yoshimoto, T., Morine, Y., Takasu, C., Feng, R., Ikemoto, T., Yoshikawa, K., et al. (2018). Blue light-emitting diodes induce autophagy in colon cancer cells by Opsin 3. Ann. Gastroenterol. Surg. 2 (2), 154–161. doi:10.1002/ags3.12055
Yuan, Y., Gong, G. Y. R., Zhang, L., Feng, T. L. C., Du, W., Yang, Y. W. F., et al. (2017). Effects of blue light emitting diode irradiation on the proliferation, apoptosis and differentiation of bone marrow-derived mesenchymal stem cells. Cell. Physiol. biochem. 43 (1), 237–246. doi:10.1159/000480344
Zein, R., Selting, W., and Hamblin, M. R. (2018). Review of light parameters and photobiomodulation efficacy: Dive into complexity. J. Biomed. Opt. 23 (12), 1. doi:10.1117/1.JBO.23.12.120901
Zhang, S., Zhang, Y., Zhang, W., Chen, S., and Liu, C., (2019), “Chronic exposure to green light aggravates high-fat diet-induced obesity and metabolic disorders in male mice., ” Ecotoxicol. Environ. Saf., vol. 178, no. , pp. 94–104. doi:10.1016/j.ecoenv.2019.04.013
Zomorrodi, R., Loheswaran, G., Pushparaj, A., and Lim, L. (2019). Pulsed near infrared transcranial and intranasal photobiomodulation significantly modulates neural oscillations: A pilot exploratory study. Sci. Rep. 9 (1), 6309. doi:10.1038/s41598-019-42693-x
Zorov, D. B., Filburn, C. R., Klotz, L. O., Zweier, J. L., and Sollott, S. J. (2000). Reactive oxygen species (ROS)-induced ROS release: A new phenomenon accompanying induction of the mitochondrial permeability transition in cardiac myocytes. J. Exp. Med. 192 (7), 1001–1014. doi:10.1084/jem.192.7.1001
Keywords: light-emiting diodes (LEDs), metabolism, photobiomodulation, phototherapy, photoacceptors, metabolomics
Citation: Veloso TM, de Souza da Fonseca A and Costa dos Santos G (2022) Effects of light-emitting diodes on cell biology. Front. Photonics 3:1018773. doi: 10.3389/fphot.2022.1018773
Received: 13 August 2022; Accepted: 24 October 2022;
Published: 10 November 2022.
Edited by:
Beop-Min Kim, Korea University, South KoreaReviewed by:
Alba Alfonso Garcia, University of California, Davis, United StatesCopyright © 2022 Veloso, de Souza da Fonseca and Costa dos Santos. This is an open-access article distributed under the terms of the Creative Commons Attribution License (CC BY). The use, distribution or reproduction in other forums is permitted, provided the original author(s) and the copyright owner(s) are credited and that the original publication in this journal is cited, in accordance with accepted academic practice. No use, distribution or reproduction is permitted which does not comply with these terms.
*Correspondence: Gilson Costa dos Santos Jr, gilson.junior@uerj.br