- 1Instituto de Biociencias de la Patagonia (INBIOP), Consejo Nacional de Investigaciones científicas y Técnicas (CONICET) and Universidad Nacional de la Patagonia San Juan Bosco (UNPSJB), Comodoro Rivadavia, Argentina
- 2Departamento de Biología y Ambiente, Facultad de Ciencias Naturales y Ciencias de la Salud, Universidad Nacional de la Patagonia San Juan Bosco (UNPSJB), Comodoro Rivadavia, Argentina
- 3Departamento de Medicina, Facultad de Ciencias Naturales y Ciencias de la Salud, Universidad Nacional de la Patagonia San Juan Bosco (UNPSJB), Comodoro Rivadavia, Argentina
- 4Instituto de Ecología, Genética y Evolución de Buenos Aires (IEGEBA), Consejo Nacional de Investigaciones científicas y Técnicas (CONICET) and Universidad de Buenos Aires, Buenos Aires, Argentina
Leaf water uptake (FWU) represents an alternative pathway to plant water acquisition that can have positive effects on water and carbon balance. Leaf surface traits including the phyllosphere microbes can affect the leaf wetness capacity and FWU. These functional and structural leaf traits could change depending on soil resources availability. The aim of this study was to evaluate the responses of FWU and leaf surface traits such as contact angle, water drop adhesion (LWA) and phyllosphere-associated microbiota to soil nitrogen addition. Three dominant plant species, Azorella prolifera, Senecio filaginoides, and Papostippa speciosa, of an arid steppe in Patagonia exposed to nitrogen (+N) and nitrogen plus water (+NW) addition for ten years were selected. Leaf contact angle did not exhibit statistical differences among treatments within species. LWA was higher in all treatments with respect to the control (C) for shrub A. prolifera and grass P. speciosa. Nitrogen addition increased significantly FWU in A. prolifera and in P. speciosa with respect to C. Colony-forming units of culturable microorganisms (CFU) on leaf surface responded to N addition, but the changes were statistically significant in S. filaginoides and P. speciosa in +NW, increasing three and eight times, respectively, in relation to the C. A positive linear relationship was found between FWU and LWA across species and treatments. On the other hand, CFU of phyllosphere was negative and exponentially correlated with LWA and FWU, across species and treatments. The results suggest that soil N enrichment could affect functional leaf traits and phyllosphere microbiota in a way that may confer a higher potential to cope with drought by facilitating the use of alternative water sources. On the other hand, we suggested that species with leaves more colonized have less surface exposed for FWU and could have lower wettability depending on the hydrophobicity degree of microbes. However, a higher cover of epiphyte’s microorganisms could compensate the effects of lower FWU by avoiding the leaf dehydration. This study contributes to a better understanding of plant leaf-microbe interactions under higher N atmospheric deposition and intensive fertilization as global agricultural production is expected to increase.
1 Introduction
Plants resistance to drought relies on several adaptive strategies, which include the use of alternative water sources (e.g., atmospheric water deposited on leaf surface). Foliar water uptake (FWU) has been observed in a wide range of ecosystems (Cavallaro et al., 2020b; Eller et al., 2013; Hayes et al., 2020; Losso et al., 2023; Matos et al., 2024; Roth-Nebelsick et al., 2023; Schreel and Steppe, 2019; Waseem et al., 2021). Some studies have showed than leaf wetting contributes to maintain the water status through FWU (Cavallaro et al., 2020a, b; Eller et al., 2013). Liquid or gaseous atmospheric water can entry to the leaf through cuticle (Fernández et al., 2017), trichomes (Losso et al., 2023; Ohrui et al., 2007; Schreel and Steppe, 2020; Waseem et al., 2021), stomatal pores (Binks et al., 2020; Guzmán-Delgado et al., 2021) or/and hydathodes (Fradera-Soler et al., 2024), when the water potential inside the leaf is lower than that in the air surrounding leaf. In addition to a favorable water potential gradient, it is necessary that the leaf exhibited certain traits that favor the leaf wetness, water adhesion and/or water retention (Carbonell Silletta et al., 2022; Roth-Nebelsick et al., 2023). These traits increase the wettability, and the amount and the time duration that water drops are maintained on leaves with possibility to be up taken and moved inside leaf.Moreover of physic-chemical cuticle traits and of inherent three-dimensional foliar microstructures (e.g., trichomes, epicuticular waxes, stomata) (Almonte et al., 2022; Fernández et al., 2024; Papierowska et al., 2018; Tie et al., 2023), aggregates microbes inhabiting leaf surface can modify the leaf hydrophilicity (Brewer and Nun, 2007; Drummond and Rosado, 2022; Holder, 2007; Rosado and Holder, 2013). At the same time, the leaf hydrophilicity degree can affect the leaf colonization by microorganisms.
Leaf surface, which represents the higher percentage of the plant phyllosphere (Sohrabi et al., 2023; Vorholt, 2012), is colonized by a wide range of microorganisms including bacteria, fungi, yeasts, algae, archaea and viruses (Lindow and Brandl, 2003). While several microorganisms on leaf surface detrimentally affect plant health and vigor (Cappelletti et al., 2016), other members have significant contributions to plant growth-promoting traits and defenses against pathogens (De Mandal and Jeon, 2023). This microbiome may also have beneficial impacts on plant water balance (Rosado et al., 2018; Vorholt, 2012). For example, aggregates of epiphytes microbiome can improve the leaf wettability through the production of hygroscopic exopolysaccharides or of surfactants (Grinberg et al., 2019; Knoll and Schreiber, 2000), restricting the leaf dehydration or increasing the water retention on leaves and thus facilitating FWU. In other cases, fungal endophytes and bacteria growing into stomata pores can active the stomatal aperture by the formation of a continue water film that connect the apoplast with the leaf surface allowing a bidirectional flow of water and solutes (Schreel and Steppe, 2020), Burkhardt et al., 2012; Fernández et al., 2017).
The heterogeneous topography of leaf surface interposed by three-dimensional microstructures generate different microhabitats for the colonization by microorganisms. Because of the exposed nature of leaves, the microbiota of phyllosphere must cope with several stress factors (e.g., high UV radiation exposition, low water and nutrient availability, high temperatures) to maintain hydration and to obtain nutrients for growth and survival. Thus, the degree of site colonization on the leaves depends on a combination of biotic and abiotic factors. Although the cuticle is the primary barrier for passage of water from the leaf interior to leaf surface in contact with the atmosphere, the base of trichomes, stomata and groves along veins are considered aqueous sites of the cuticle (Schönherr, 2006), favoring the microbiota establishment (Chaudhry et al., 2021). These sites are likely also rich in leaf-derived soluble nutrients (Beattie, 2011). It has been found that water and nutrient content in plant tissues and on the leaf surface are positively correlated (Derridj, 1996; Yadav et al., 2005).
Plants and microbes, alone or in interaction, are faced with quick changes in their environment due to climate change (changes in precipitation and temperature) and to anthropogenic activities leading to higher atmospheric N deposition or to changes in soil chemical properties, directly through agronomic fertilization. These factors can modify the leaf functional traits (Binks et al., 2020; Bucci et al., 2006; Cavallaro et al., 2023), micro topology and physicochemical properties (Chin et al., 2023a; Whipps et al., 2008), affecting the microbiome colonization degree and diversity (Karlsson et al., 2017) and thus to the complex interplay of plant-microbiome association (Zhu et al., 2022). Cavallaro et al. (2023) showed that soil water addition decreased FWU, while leaf wettability remained unchanged in several woody species in a semiarid steppe. On the other hand, exposure to climate variation (temperature and humidity) resulted in acclimation of leaf surface traits related with FWU in some species of other ecosystems (Chin et al., 2023b). The effects of environmental changes have been more studied for microbial communities in the soil and associated to roots (e.g., Hartman and Tringe, 2019; Li et al., 2021; Trivedi et al., 2017) but less for epiphytic phyllosphere microbiota (e.g., Darlison et al., 2019; Flexas et al., 2018; Xiong and Lu, 2022). Mainly, there is a paucity of information on the effects of changes in soil water and nutrient inputs on FWU and leaf surface traits. Consequently, in this study we evaluated the effects of long-term soil N addition and N plus water addition on FWU, leaf traits related to wettability and colony-forming units of culturable microorganisms in the phyllosphere (CFU) of three native plant species in a Patagonian steppe in Southern Argentina, characterized by low precipitation and soil nutrients. We also explored the relationships between FWU, wettability traits and CFU across species and treatments.
2 Materials and methods
2.1 Site description and study species
The study was conducted at the Río Mayo Experimental Field Station of INTA (National Institute of Agricultural Technology) in South Central Patagonia (45°24′11″S 70°17′37″W, 500 m a.s.l.), in the growing season of 2022. The study site has a mean annual temperature (1982-2018) of 9.4°C, with a mean monthly temperature in the warmer month (January) of 15°C and in the cooler month (July) of 2°C (Carbonell-Silletta et al., 2024). The historical mean annual precipitation in the last four decades is 130 mm, with an annual range between 50 and 250 mm (Carbonell-Silletta et al., 2024). Most precipitation occurs in the autumn and winter, while in the growing season (spring-middle summer) precipitation is scarce and occurs as small and unpredictable events (55% of them are less than 1 mm) (Carbonell Silletta et al., 2022; Cavallaro et al., 2020b). Soil nitrogen content (Carbonell Silletta et al., 2022) and moisture (Pereyra et al., 2017) are very low. The vegetation is distributed in patches composed of shrubs and grasses on a bare soil matrix (Soriano et al., 1994). The dominant shrub species in the study area are Adesmia volckmannii Phil., Azorella prolifera (Cav.) G.M. Plunkett & A.N. Nicolas and Senecio filaginoides DC. The dominant grass species are Poa ligularis Nees ex Steu, Pappostipa speciosa (Trin. & Rupr.) Romasch and Pappostipa humilis (Cav.) Romasch.
For this study, two species of shrubs (A. prolifera and S. filaginoides) and one of grass (P. speciosa) were used. All determinations were carried out during the growing season (December).The study involved two treatments with soil nitrogen addition (+N) and nitrogen with water addition (+NW) and a control (C). The experiment was initiated in 2013 and continues to the present. It consists of 15 plots of 625 m2 each, separated into 5 plots per treatment. Fertilized plots were treated with 100 kg ha-1 yr-1 in the form of urea and diammonium phosphate (160 kg and 154 kg, respectively) in two applications per year for 9 years. After, the amount of fertilizer was reduced to 25 kg ha-1 yr-1. Plots with water addition are equipped with a semi-automatic system of sprinkler irrigation in which the amount of historical annual precipitation for the site is increased by approximately 25%, distributed in events of 5 mm each during the growing season. After 2021 we added a single water pulse of 20 mm in each plot with +NW. More details on experimental design can be found in Carbonell-Silletta et al. (2024).
2.2 Soil and leaf N content
To determine total nitrogen, three soil cores (5 cm diameter and 5 cm depth) were randomly taken from each plot and mixed well to obtain a composite sample (n = 5). Total nitrogen was determined by the Kjeldahl method (Bremner, 1996).
Leaf nitrogen content was determined in fully expanded and healthy leaves from different individuals of each species and in each plot. A composite sample of different individuals was used per plot and species. Total leaf N content was measured using the Kjeldahl technique.
2.3 Leaf water content
To determine leaf water content (LWC) fully expanded and healthy leaves from different individuals of each species and in each plot were collected. Fresh weight (FW) was determined using a precision electronic balance (0.001 g precision, ACCULAB ALC-210.4). Then, leaves were dried in oven at 70°C per 72 h and the dry weight (DW) was determined. Leaf water content (g H2O g-1 dry weight) was determined as follows:
2.4 Foliar water uptake
To assess foliar water uptake (FWU), the methodology described by Limm et al. (2009) was followed. Thirty fully mature leaves per species and treatment were cut at midday and transported in sealed bags to the measurement site. Petioles were sealed, and the leaves were submerged in distilled water for 3 hours. This duration is based on previous studies on the same species and study site (Cavallaro et al., 2020a). After time, the leaves were removed from the water and dried with paper towels before being weighed again. To evaluate errors associated with drying, the procedure followed to Cavallaro et al. (2020a). We determined the increase in water mass expressed in mmol and then we normalized by leaf area (m2).
FWU was calculated as:
Where Mass1 is the initial mass and Mass2 is the final mass after immersion, Residual Mass1 is the initial mass of the leaf after the air-drying event before being submerged again, and Residual Mass2 is the final mass after being briefly wetted and LA is the leaf area (m2).
2.5 Leaf water drop adhesion
To evaluate the amount of water on leaf surface per unit leaf area at a point which additional water can no longer be retained and starts to drip off (i.e. leaf water drop adhesion), we used the protocol described in Cavallaro et al. (2022). Thirty mature leaves per species and treatment were used. The leaves were weighed using a precision electronic balance (0.001 g precision, ACCULAB ALC-210.4) to obtain their initial mass (Mass1). Leaves were immediately submerged for 10 seconds in distilled water, then removed and allowed to drain for 10 seconds. Posteriorly, final leaf mass was determined (Mass2). The procedure lasted less than 1 minute, avoiding water losses due to transpiration before wetting and later due to evaporation after immersion in water. Then, the leaf area (LA) was determined using the ImageJ (1.52a) software. Leaf water adhesion (LWA, g m-2) was calculated as:
where Mass1 (g) corresponds to the leaf mass before immersion, Mass2 (g) is the leaf mass after being submerged and LA is the leaf area (m2).
2.6 Contact angle
The contact angle formed by a tangent line to the point of contact between a water droplet and the leaf surface in a horizontal state was measured using a 1 μl droplet. We used this size droplet because the species of interest have small leaves (Cavallaro et al., 2022) and even so, it was not possible to perform measurements on P. speciosa. The contact angle was measured by photographs of the droplets on the leaf surfaces taken with a camera attached to a magnifying glass and analyzed with the ISCapture software (V3.6.6).
2.7 Colony forming units
To quantify colony-forming units (CFU) on phyllosphere, the protocol described by Mina et al. (2020) was adapted. One g of leaves from each species and treatment was immersed in test tubes containing 10 mL of peptone water (BRITANIA, 10 g/l peptone and 5 g l-1 sodium chloride) and shaken with an orbital shaker (ARCANO TS-1000) at 100 rpm for one hour at room temperature. Aliquots of 100 μL of the suspension were plated in triplicate on nutrient agar medium (BRITANIA, 5 g l-1 pluripeptone, 3 g l-1 meat extract, 8 g l-1 sodium chloride, and agar 15 g l-1) and incubated in darkness at a constant temperature of 25°C. Daily observations were made at 24 h, 48 h, and 72 h. The number of CFU was recorded at 72 h after seeding.
Cultivable bacteria from superficial soil were determined by the dilution plate count method (Pepper and Gerba, 2015). Six soil samples per treatment were collected. For each sample, 1 g of soil was weighed and placed in dilution bottles containing 20 ml of sterile saline solution. Bottles were shaken at 1000 rpm for 5 min, and then 1 ml of the suspension was serially diluted and 0.1 ml spread-plated onto nutritive agar. Plates were incubated at 25°C for 4 days. Then, colony-forming units (CFU) were determined. Only plates containing between 30 and 300 colonies were considered for counting.
2.8 Observation of leaf surface microbial colonization by epifluorescence
To visualize microorganisms on leaf surface we followed the method used by (Morris et al., 1997). Briefly, 20 to 40 fresh and healthy leaves of A. prolifera and P. speciosa were collected in the field, Then, in the laboratory leaves were immersed in a solution of acridine orange for two minutes, raised two folds with distilled water, set on a microscope slide and observed with an epifluorescence microscope Trino Biotraza XSZ146AT with a camera Leica DFC 450 C attached.
2.9 Statistical analysis
We used one-way ANOVA to assess the effects of N addition on soil N content, soil CFU, leaf N content, leaf water content, foliar water uptake, leaf water drop adhesion, and CFU on phyllosphere. Each species was analyzed separately. The normality and homoscedasticity of data were tested. Treatment means were compared to control with a Tukey post-hoc test when significant differences at p<0.05 were detected in the ANOVAs. Simple regression analyzes were tested separately to evaluate the relationship between LWA and FWU, CFU and LWA, and CFU and FWU. Regression analyzes were performed for all treatments and species together. All statistical analyzes were performed with Statistica 7.0 software (StatSoft, OK, US) and graphs were performed using SigmaPlot 12.6 (Systat Software, Inc., Point Richmond, CA).
3 Results
Soil N content in the +N and +NW plots increased substantially in relation with C plots, although the differences were not statistically significant (Table 1). Culturable colony-forming units in the soil were lower in the soil of the plots with +N and +NW compared to the soil in C plots, with significant differences in +N (p<0.05).
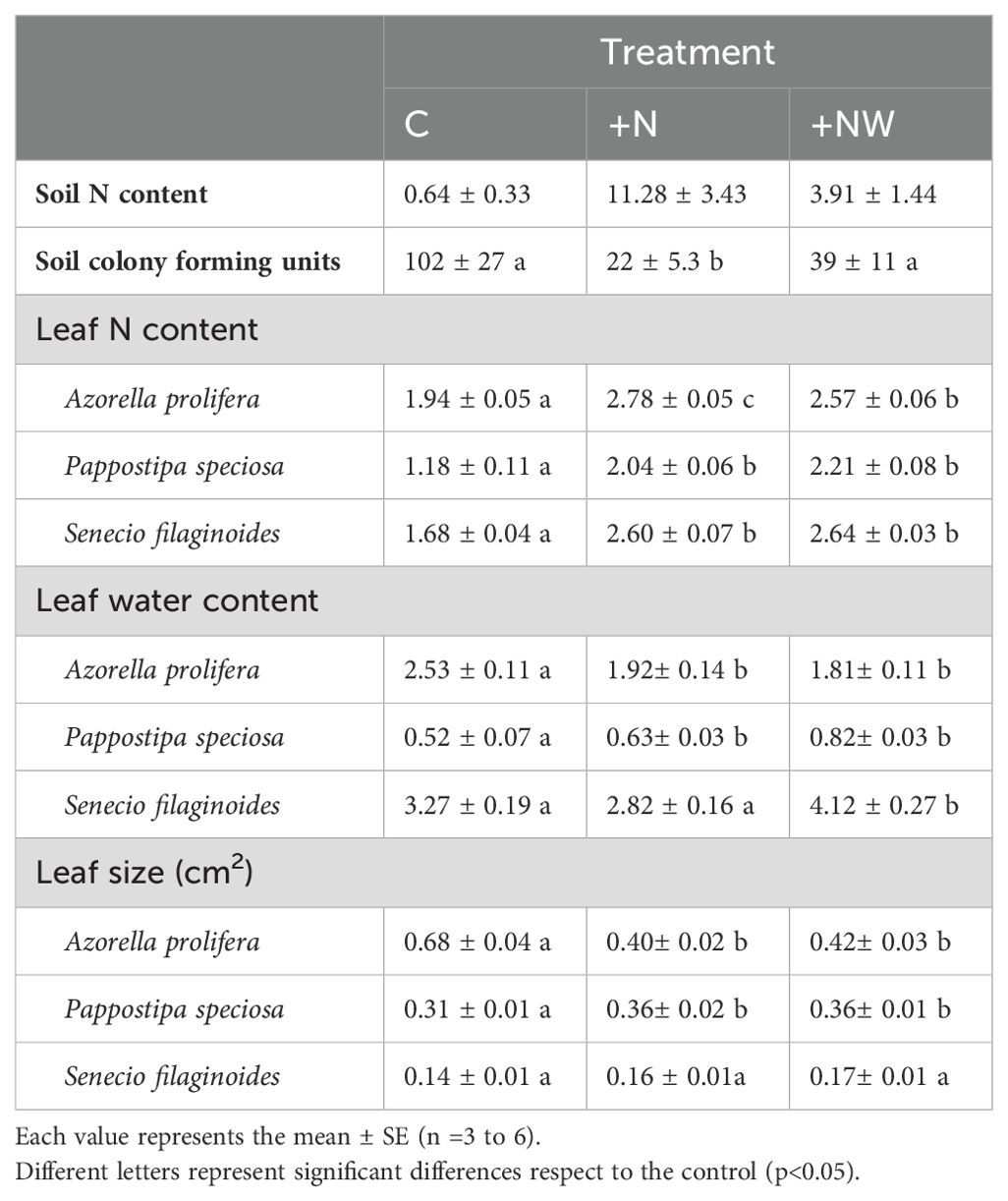
Table 1. Soil inorganic nitrogen content (ppm), colony forming units in the soil (CFU μl-1), leaf nitrogen content (%), leaf water content (g H2O g-1 dry mass) and leaf size (cm2) for control (C), Nitrogen addition (+N) and Nitrogen plus water addition (+NW) treatments.
Leaf nitrogen content was significantly higher in leaves of all study species under N addition (+N and +NW) (Table 1). The maximum increments were observed in +N, being in A. prolifera at 43%, in P. speciosa at 72% and in S. filaginoides at 55% in relation to the control. Leaf water content decreased significantly in N+ and NW+ treatments in A. prolifera (p<0.05) (Table 1). However, in P. speciosa, the leaf water content increased significantly in +N and +NW (p<0.05). In S. filaginoides leaf water content was significantly higher in +NW (p<0.05) and had no statistical differences in N+ plots. Leaf area decreased in A. prolifera and increased in P. speciosa with significant differences (p<0.05) and there were no differences in S. filaginoides under N+ and NW+ treatments (Table 1).
All the studied species exhibited FWU during the growing season, with values ranging from 0.37 ± 0.02 mmol m-2 s-1 in P. speciosa to 1.12 ± 0.05 mmol m-2 s-1 in A. prolifera (Figure 1). Foliar water uptake increased or tended to increase in the +N and +NW treatments compared to the control in all species. In A. prolifera, FWU increased significantly only in +N compared to the control (p <0.01) (Figure 1). Although in S. filaginoides the FWU increased by 30% in the +N treatment compared to the control, this change was not statistically significant. In the grass P. speciosa, FWU increased by 60% in the +N treatment compared to the control (p <0.01).
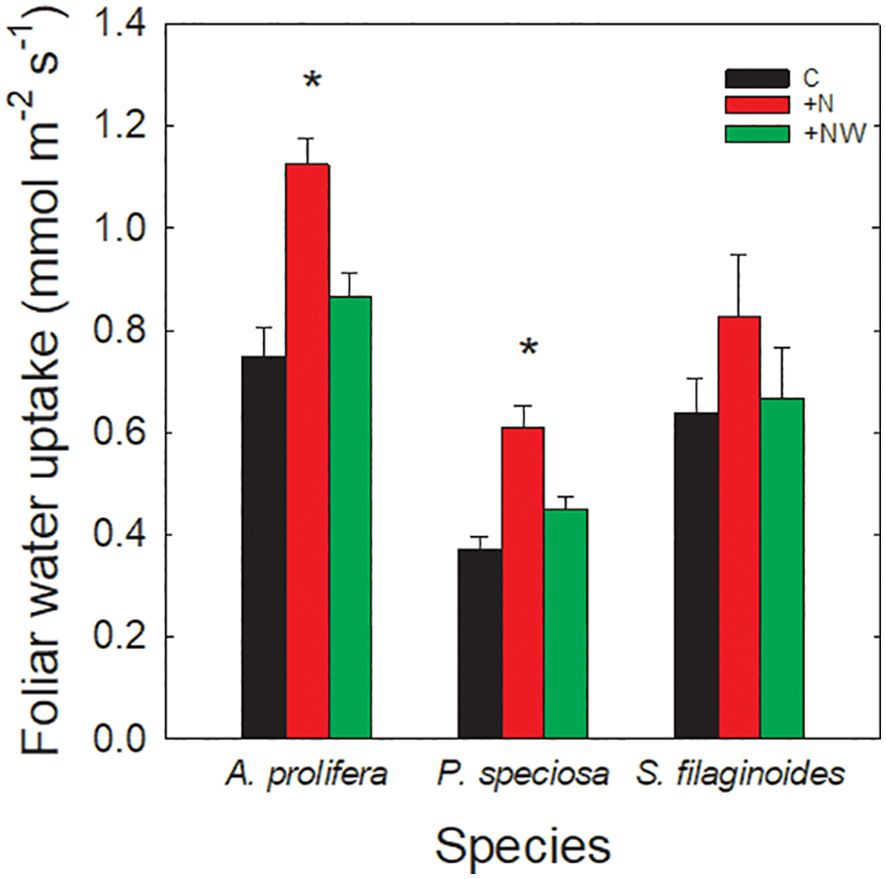
Figure 1. Foliar water uptake in control (black bars), Nitrogen addition (+N; red bars), and combination of Nitrogen and water addition (+NW; green bars) treatments. Each bar represents the mean value ± SE of 30 leaves. The asterisks indicate significant differences compared to the control treatment. (p<0.05). *Statistical difference.
Leaf water drop adhesion (LWA) had a similar behavior in A. prolifera and P. speciosa (Figure 2). In both species, LWA increased significantly with N addition (+N and +NW) (p <0.01). In A. prolifera LWA increased about 70% in +N and +NW compared to the C. In P. speciosa LWA increased more than two-folds in the +N and +NW treatments compared to the control (19.75 ± 1.64 g m-2, 15.77 ± 1.59 g m-2, and 8.00 ± 1.00 g m-2, respectively; p <0.01). In S. filaginoides there were no significant differences in LWA between treatments, varying from 144.19 ± 9.6 g m-2 in +NW to 188.59 ± 13.96 g m-2 in the control.
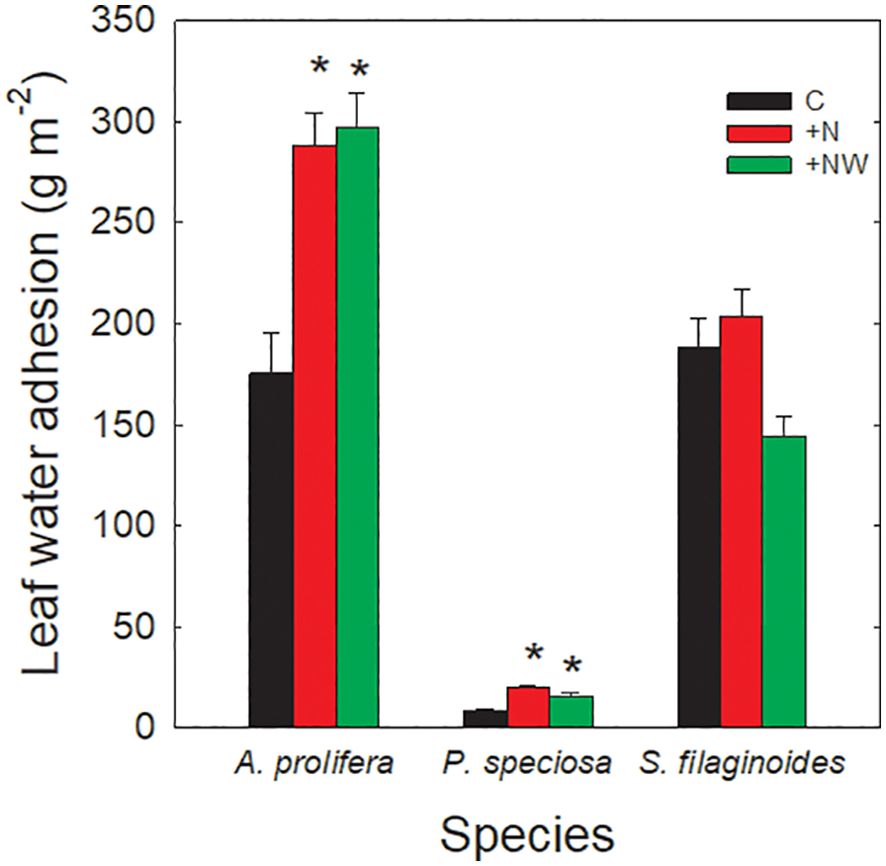
Figure 2. Leaf water adhesion (g m-2) in Azorella prolifera, Pappostipa speciosa and Senecio filaginoides in each treatment: control (C; black bars), Nitrogen addition (+N; red bars) and Nitrogen and water addition (+NW; green bars). Each bar represents the mean value ± SE of 30 leaves. Significant differences with the control treatment are indicated with an asterisk (p<0.05). *Statistical difference.
No significant differences between nitrogen addition treatments and control were found for the contact angle between a water droplet and the leaf surface, however there was a tendency to increase (data not shown). The contact angle in A. prolifera varied between 60.7° ± 2.12° in the control and 68° ± 3.9° in +NW treatment, while in S. filaginoides ranged from 126.18° ± 2.76° in the control to 136° ± 2.93° in the +N treatment.
The three species showed a trend to have higher CFU on phyllosphere per µL in the treatments with N addition (Figure 3). In A. prolifera CFU increased more than two and eight-folds in +N and +NW, respectively, compared to the control, although the differences were not statistically significant (0.01 ± 0.008 CFU µL-1 in the control, 0.04 ± 0.03 CFU µL-1 in +N and 0.08 ± 0.002 CFU µL-1 in +NW). Senecio filaginoides showed significant differences in the combined treatment compared to the control (p<0.05). The highest value of CFU per µL for this species was found in the +NW treatment (0.22 ± 0.03 CFU µL-1), while in the control CFU were 0.06 ± 2.52 CFU µL-1. In P. speciosa, the lowest amount of CFU per µL was found in the control (0.05 ± 0.01 CFU µL-1) and the highest in the +NW treatment (0.41 ± 0.01 CFU µL-1), with a significant difference between these two treatments (p<0.05).
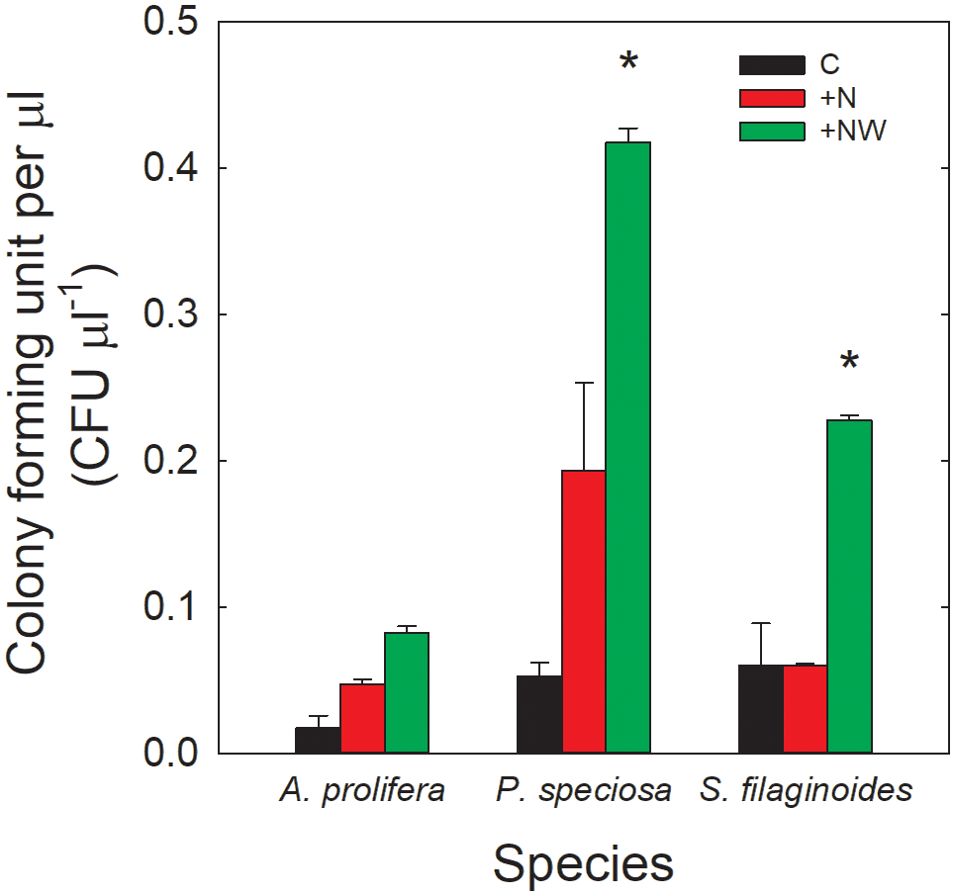
Figure 3. Colony-forming units of culturable microorganisms (CFU µl-1) in control (C; black bars), Nitrogen addition (+N; red bars), and nitrogen and water addition (+NW; green bars) treatments. Each bar represents the mean value ± SE; n=4. The asterisks indicate significant differences compared to the control treatment (p<0.05). *Statistical difference.
Aggregates of microorganisms were observed on leaves of A. prolifera and P. speciosa stained with orange acridine. Higher leaf surface area fluorescence was observed in +NW treatments compared with the C in the two species analyzed (Supplementary Figure S1).
There was a positive and significant relationship between FWU and LWA (Figure 4). A single function was fitted across species and treatments (y = 0.4 + 0.0018x, R2 = 0.79, p <0.001) although each species tended to have a different operating range along the common functional response curve. The species with the lowest LWA (P. speciosa) was also that with the lowest FWU, while A. prolifera, with the highest LWA, also had the highest FWU.
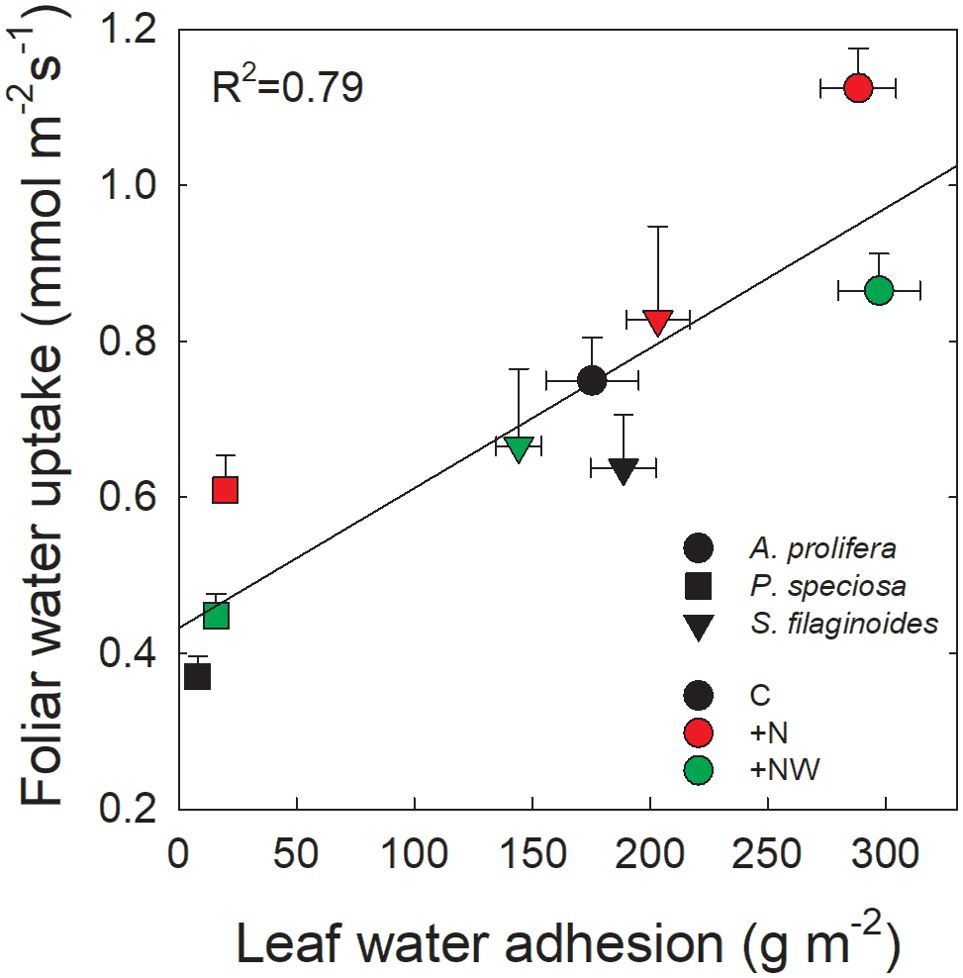
Figure 4. Relationship between leaf water adhesion (LWA; g m-2) and foliar water uptake (FWU; mmol m-2 s-1) across species and treatments. Control (black symbols), nitrogen addition (red symbols) and Nitrogen and water addition (green symbols). Each symbol represents the mean value ± SE (n=30 for LWA and FWU). The function fitted to the data is y= 0.43 + 0.002x; R2 = 0.79; p<0.001.
The CFU per µL were related through a decreasing exponential function with LWA (y = 0.35exp(-0.007x); R2 = 0.66; p < 0.01) (Figure 5A) and with FWU (y = 4.46exp(-5.3x), R2 = 0.8 p < 0.001) (Figure 5B) across species and treatments. Species with higher LWA and FWU showed a lower quantity of CFU per µL on the leaf surface.
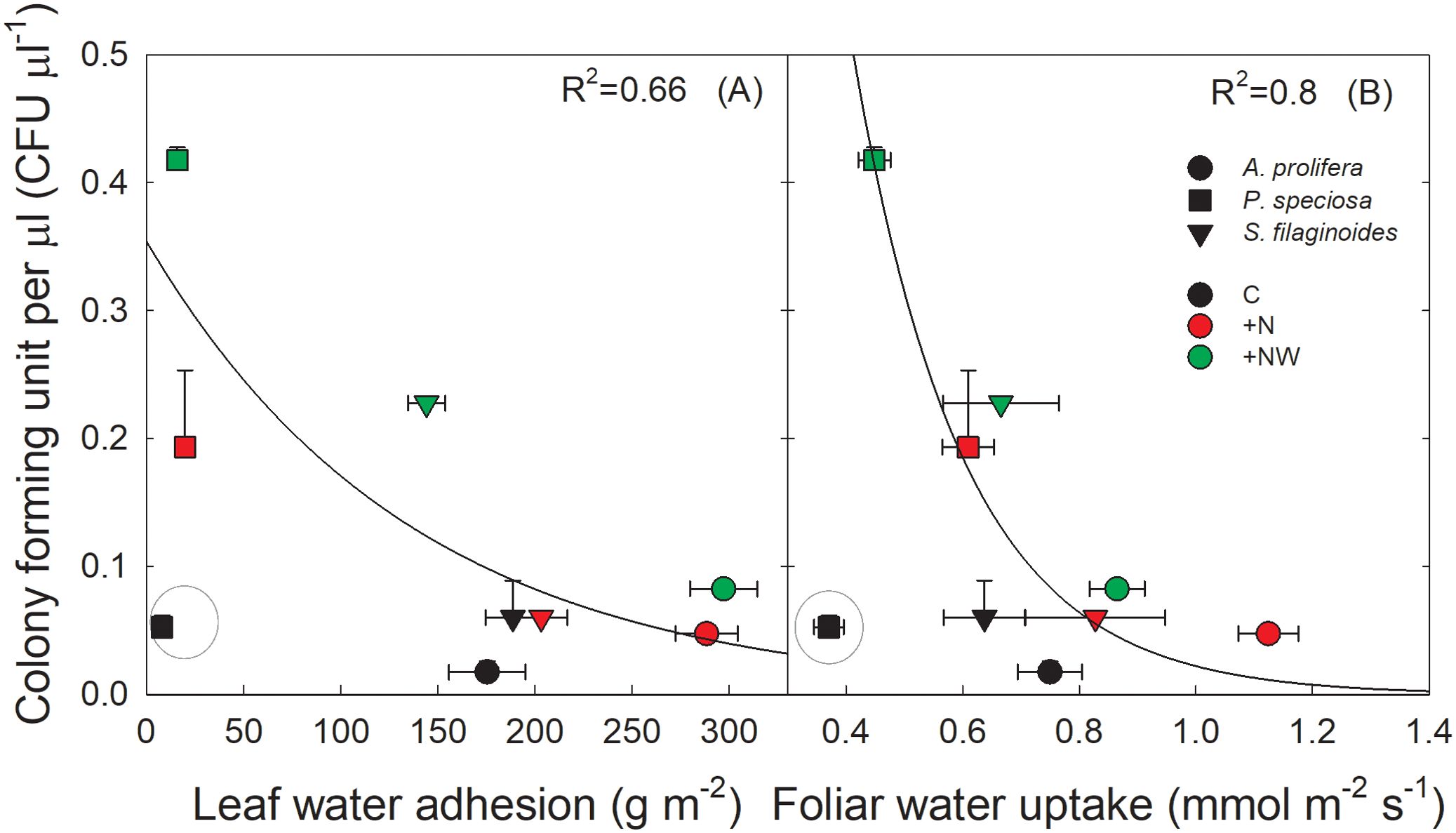
Figure 5. Relationship between colony-forming units of culturable microorganisms (CFU µl-1) and (A) leaf water adhesion (LWA; g m-2) and (B) foliar water uptake (FWU; mmol m-2s-1) across species in control (black symbols), Nitrogen addition (red symbols) and Nitrogen plus water addition (green symbols) treatments. Each symbol represents the mean value ± SE (n=4 for CFU and n=30 for LWA and FWU). The functions fitted to the date are: (A) y = 0.65e(-0.007x); R2 = 0.66; p<0.01 and (B) y = 4.46e(-5.3x); R2 = 0.8; p<0.001. Values of P. speciosa in the Control plots were not included to fit the functions and were considered outliers.
4 Discussion
In this study, we observed a high plasticity in the leaf responses to changes in soil resources availability in species from different functional groups growing in an arid environment characterized by low soil N availability. Soil and leaf nitrogen enrichment impacted on functional leaf traits as FWU and on leaf surface properties that increased the force of water drop adhesion and the colonization of culturable microorganisms on leaf surface.
Consistent with previous studies in the Patagonian steppe (Cavallaro et al., 2022, 2023; Cavallaro et al., 2020a), the three study species absorbed water via FWU. Values of FWU observed for shrubs and grasses in this steppe are relatively higher than those found in other ecosystems worldwide (Berry et al., 2019; Losada et al., 2021; Losso et al., 2023), suggesting that FWU have an important role in the water balance for these species (Cavallaro et al., 2020a, b). This mechanism allows shrubs and grasses to use the leaf wetting events due to dew formation and to small rain pulses occurring during spring and summer (Cavallaro et al., 2020b) when soil water availability decreases due to low precipitation and high air saturation deficit (Pereyra et al., 2017). Thus, FWU contributes to the recovery of the leaf water potential and thus avoiding or reducing the water stress (Cavallaro et al., 2020b; Matos et al., 2024; Tian et al., 2024; Wang et al., 2023; Yokoyama et al., 2021).
Several studies have analyzed the plant plasticity to changes in soil resources (Arias et al., 2015, 2021; Bucci et al., 2006; Cavallaro et al., 2023; Costa et al., 2021; Scholz et al., 2007), however, it is not well understood how FWU and leaf wettability acclimate to new environmental conditions. Under improved soil water availability, Cavallaro et al. (2023) found that in shrub species the FWU decreases, and it was related more to changes in the gradient between leaf water potential and the water potential of the air surrounding the leaf surface more than to leaf surface traits such as contact angle, water drop adhesion and retention. In our study, we observed a relative plasticity not only in FWU but also in leaf wetness properties predicted by LWA under N addition alone or combined with water addition in two of the species analyzed. However, while FWU tended to increase only with +N, the LWA increased similarly in both treatments. The negative effect of soil water addition on FWU observed previously by Cavallaro et al. (2023) in these species was compensated by an increment due to nitrogen addition. Thus, the interactive effect of +NW observed on FWU suggests antagonistic impacts of increased soil N content and increased soil water inputs. On the other hand, the interactive effects of combined treatment on LWA confirmed the low plasticity of the biophysical leaf surface traits to higher precipitation (Cavallaro et al., 2023), resulting in a similar response of LWA to +N and to +NW. Recently, Carbonell-Silletta et al. (2024) showed that Patagonian shrubs and grasses respond more to soil N addition than to soil water addition, suggesting a higher limitation by soil nutrients than by soil water content. Changes in the amount and distribution of waxes, number of trichomes and stomata and in cuticle thickness could explain the improved LWA observed under N addition. At this instance, we have no information on how these leaf traits were modified under N addition. However, studies in other ecosystems indicate that soil N enrichments can change stomatal density (Costa et al., 2021; Zhu et al., 2020), trichomes density (Bilkova et al., 2016), the cuticle properties (Batool et al., 2024) and, consequently, could affect the FWU, leaf wettability and probably increase the leaf water uptake at a plant level. Although some studies have found that higher trichome density is associated with higher water drop adherence on leaf surface and higher FWU (Fernández et al., 2014; Li et al., 2023; Pan et al., 2021), other studies suggest that trichomes increase the hydrophobicity of leaf surface (Brewer et al., 1991; Durante et al., 2011; Fernández et al., 2014). In our study we observed that P.speciosa exhibites lower values of LWA compared with S. filaginoides and A. prolifera. P. speciosa is a grass that has distinctive leaf traits as longitudinal grooves running alongside the leaf that are known to act like channels for guide the drop of water to the base of the plants at the root zone (Roth-Nebelsick et al., 2012). This leaf trait could help grasses to use alternative sources of water and maintain leaf water status under periods of water deficits moving water downward toward the root zone. LWA need to be lower in the grooves of the leaf to enhanced water movement to the base of the plant. However, under N+ and NW+ treatments P. speciosa increased LWA and the amount of CFU, probably because N+ addition increased trichomes or modified density of stomata that could increase the sites of colonization for microorganisms and thus improve leaf wettability.In addition to possible changes in structural traits of the leaf surface, Knoll and Schreiber (2000) suggested that native wetting properties of leaf surfaces given by hydrophobic nature of cutin and waxes, could be significantly masked by the presence of epiphytic microorganisms. Some studies show that the leaf surface colonized by microorganisms is more wettable (Knoll and Schreiber, 2000; Schreiber et al., 2005) and that epiphytic microbes can increase the water permeability of cuticle (Schreiber et al., 2005). Both effects can improve the leaching of inorganic and organic substances from the leaf interior through the cuticle to the leaf surface. Thus, leaf water adhesion increase observed under N treatments may be correlated with the higher colonization by epiphytic microorganisms, as suggested by the higher colony-forming units count of culturable microorganisms. In P. speciosa and A. prolifera there was an increase in LWA and CFU. Higher CFU could also increase the amount of extracellular polymeric substances (EPS) and biosurfactants secreted by some microorganisms as a dispersed soluble slime on the leaf surface as well as the hydrophilicity of the surface (Knoll and Schreiber, 2000).
As the phyllosphere is an open system, microbes composition and abundance can be directly related to the surrounding environment of host plants (i.e., soil, air, neighbor plants) (Brown et al., 2020; Song et al., 2015; Zhu et al., 2022). Thapa et al. (2017) found that the quantity of cultivable microorganisms is modified in accordance with the amount of N in leaves and soil. Thus, an explanation about why soil nitrogen addition increased the CFU in the leaves could be related to changes in the soil biota due to fertilization. However, we may discharge this possibility, because, although we did not determine the biomass and diversity of the soil microbes under N addition, the soil CFU counts in +N and +NW plots were substantially lower than those in the C plots. A plausible explanation for the increase of CFU count in the phyllosphere of plants under N addition is the higher leaf N content. Nitrogen absorbed by roots and then transported to leaves may arrive to leaf surface by moving through aqueous routes in the cuticle (Schönherr, 2006), which in turn results in a better nutrient supply to leaf surface microorganisms. Although in some ecosystems it is not expected that N be a growth limiting factor for organisms living on leaf surface due to high atmospheric nitrogen deposition, it does not occur in the Patagonian steppes where N deposition is relatively low (Yahdjian et al., 2011) and leaf N content is very low (Carbonell-Silletta et al., 2024). Different studies have showed that leaf N is one of the main factors affecting the composition of bacteria and fungi in phyllosphere (Kembel et al., 2014; Liu et al., 2023). In addition, higher relative water content observed in leaves of P. speciosa and S. filaginoides under N treatments could contribute to higher microbiome colonization. For some mediterranean woody and non-woody species, leaf water content was found to be the primary explanatory variable for phyllosphere microbial colonization (Yadav et al., 2005). Because the Patagonian steppe exhibits higher productivity under N addition (Carbonell-Silletta et al., 2024), higher photosynthesis rate could increase the availability of other nutrients, such as soluble carbohydrates, to phyllosphere microorganisms (Kembel et al., 2014; Yadav et al., 2005). The observation of epifluorescence in the furrows of the leaves of A. prolifera and in the uncinate trichomes in P. speciosa supports the higher degree of colonization of microsites with a higher probability of co-localization of higher water, nutrient, and energy-rich molecules availability by culturable microbiota, mainly in the leaf surface under +NW. In addition to the role of epiphytic microorganisms in increasing LWA, higher coverage of the leaf surface by microorganisms could contribute to maintaining the water status by avoiding the leaf dehydration. To survive in the harsh leaf environment, microorganisms can trigger the production of biofilms, which are characterized as “water-filled regions” (Quan et al., 2022) to protect against desiccation. As we have quantified only culturable microbial communities, it is possible that the response of phyllosphere to changes in the surrounding environments may be more complex than that observed in this study.
We found a relationship between FWU and LWA with CFU across species and treatments, but with the species occupying different portions of this gradient in leaf traits. This suggests that species differ in their capacity of foliar water uptake which could depend on leaf structural characteristics, soil water access, and stomatal regulation (Cavallaro et al., 2023, a; Wang et al., 2023), and that host species is a main factor determining the composition and diversity of epiphytes in the phyllosphere (Vogel et al., 2021; Vokou et al., 2012). Although FWU and the microorganisms in the phyllosphere contribute both to avoid the water stress, the inverse relationship observed between these leaf characteristics across species suggest that both strategies could not occur simultaneously.
In this study, we observed that under natural field conditions plants with higher FWU were those with less CFU. Higher CFU could compensate the lower FWU and thus contribute to maintaining plant water status. At one end of this relationship is the grass species while on the opposite side is the shrub A. prolifera; however, these study species reach similar minimum water potentials during the dry season (Bucci et al., 2011). We assumed that species with higher CFU counts probably have higher leaf surface covered with microorganisms and, thus, lower leaf surfaces exposed to FWU. In addition, this is probable that traits favoring the microbial colonization do not contribute to FWU. For example, species such as P. speciosa and S. filaginoides have trichomes, which are considered hydrophobic, depending on the density, structure and chemical composition (Fernández et al., 2014), decreasing leaf water retention (Brewer et al., 1991). However, the trichomes could constitute sites colonized by epiphytes (Monier and Lindow, 2004; Yadav et al., 2005). On the other hand, the effects of the phyllosphere on wettability are dependent on the hydrophobicity of organisms (Rosado and Almeida, 2020). In this study we did not identify the microbes, however, we found many CFU of fungi in P. speciosa and S. filaginoides while in leaves of A. prolifera were not observed (data not shown), which could affect the leaf wettability. Duan et al. (2024) suggest the presence of a “core microbiome” in the leaves that could be modified by differences in leaf traits with an important role at the ecosystem level. In arid ecosystems, plants that favor the colonization and establishment of microorganisms could have advantages in maintaining the leaf water status.
In conclusion, the results indicate that soil N inputs not only enhance plant nutrient availability, but also increase the leaf wettability and the capacity to use alternative water sources by FWU. Moreover, the higher leaf surface covered by microorganisms may reduce the leaf dehydration, contributing to cope with water shortage or higher atmospheric evaporative demand during the dry season. These responses observed under N addition may explain the higher plant growth observed in +N and +NW plots by Carbonell-Silletta et al. (2024) in this Patagonian steppe. Overall, this study helps to a better understanding of plant leaf-microbe interactions and to predict how the phyllosphere could respond to climate change and to higher N atmospheric deposition and intensive fertilization as global agricultural production is expected to increase.
Data availability statement
The raw data supporting the conclusions of this article will be made available by the authors, without undue reservation.
Author contributions
MP: Data curation, Formal Analysis, Investigation, Methodology, Writing – review & editing. NA: Conceptualization, Writing – original draft, Writing – review & editing. GP: Investigation, Writing – review & editing. LC: Formal Analysis, Funding acquisition, Writing – review & editing. RS: Methodology, Writing – review & editing. GG: Conceptualization, Writing – review & editing. FS: Conceptualization, Funding acquisition, Writing – review & editing. SB: Conceptualization, Funding acquisition, Project administration, Writing – original draft, Writing – review & editing.
Funding
The author(s) declare financial support was received for the research, authorship, and/or publication of this article. The authors acknowledge the funding support from CONICET (PUE-INBIOP 00033) and Fondo para la Promoción Científica y Tecnológica (FONCyT; grant PICT 2019-1306 and grant PICT 2021-831). We thank to staff of Instituto Nacional de Tecnología Agropecuaria (INTA) for allowing access and assistance at the Rio Mayo Experimental Field.
Acknowledgments
The authors acknowledge the funding support from CONICET (PUE-INBIOP 00033) and Fondo para la Promoción Científica y Tecnológica (FONCyT; grant PICT 2019-1306 and grant PICT 2021-831). We thank to staff of Instituto Nacional de Tecnología Agropecuaria (INTA) for allowing access and assistance at the Rio Mayo Experimental Field. This work complies with Argentinian law. The authors have no conflict of interest.
Conflict of interest
The authors declare that the research was conducted in the absence of any commercial or financial relationships that could be construed as a potential conflict of interest.
The author(s) declared that they were an editorial board member of Frontiers, at the time of submission. This had no impact on the peer review process and the final decision.
Publisher’s note
All claims expressed in this article are solely those of the authors and do not necessarily represent those of their affiliated organizations, or those of the publisher, the editors and the reviewers. Any product that may be evaluated in this article, or claim that may be made by its manufacturer, is not guaranteed or endorsed by the publisher.
Supplementary material
The Supplementary Material for this article can be found online at: https://www.frontiersin.org/articles/10.3389/fphgy.2024.1457037/full#supplementary-material
References
Almonte L., Pimentel C., Rodríguez-Cañas E., Abad J., Fernández V., Colchero J. (2022). Rose petal effect: A subtle combination of nano-scale roughness and chemical variability. Nano Select 3, 977–989. doi: 10.1002/nano.202100193
Arias N. S., Bucci S. J., Scholz F. G., Goldstein G. (2015). Freezing avoidance by supercooling in Olea europaea cultivars: The role of apoplastic water, solute content and cell wall rigidity. Plant Cell Environ. 38, 2061–2070. doi: 10.1111/pce.12529
Arias N. S., Scholz F. G., Goldstein G., Bucci S. J. (2021). Low-temperature acclimation and legacy effects of summer water deficits in olive freezing resistance. Tree Physiol. 41, 1836–1847. doi: 10.1093/treephys/tpab040
Batool F., Bahadur S., Long W. (2024). Soil nutrients determine leaf traits and above-ground biomass in the tropical cloud forest of Hainan Island. Front. Forests Global Change 7. doi: 10.3389/ffgc.2024.1342135
Beattie G. A. (2011). Water relations in the interaction of foliar bacterial pathogens with plants. Annu. Rev. Phytopathol. 49, 533–555. doi: 10.1146/annurev-phyto-073009-114436
Berry Z. C., Emery N. C., Gotsch S. G., Goldsmith G. R. (2019). Foliar water uptake: Processes, pathways, and integration into plant water budgets. Plant Cell Environ. 42, 410–423. doi: 10.1111/pce.13439
Bilkova I., Kjaer A., van der Kooy F., Lommen W. J. M. (2016). Effects of N fertilization on trichome density, leaf size and artemisinin production in Artemisia annua leaves. Acta Hortic. 1125, 369–376. doi: 10.17660/ActaHortic.2016.1125.48
Binks O., Coughlin I., Mencuccini M., Meir P. (2020). Equivalence of foliar water uptake and stomatal conductance? Plant Cell \& Environ. 43, 524–528. doi: 10.1111/pce.13663
Bremner J. M. (1996). “Nitrogen-total,” in Methods of Soil Analysis (Madison, Wisconsin: John Wiley & Sons, Ltd), 1085–1121. doi: 10.2136/sssabookser5.3.c37
Brewer C. A., Nun C. I. (2007). Patterns of leaf wettability along an extreme moisture gradient in Western Patagonia, Argentina. Int. J. Plant Sci. 168, 555–562. doi: 10.1086/513468
Brewer C. A., Smith W. K., Vogelmann T. C. (1991). Functional interaction between leaf trichomes, leaf wettability and the optical properties of water droplets. Plant Cell \& Environ. 14, 955–962. doi: 10.1111/j.1365-3040.1991.tb00965.x
Brown S. P., Grillo M. A., Podowski J. C., Heath K. D. (2020). Soil origin and plant genotype structure distinct microbiome compartments in the model legume Medicago truncatula. Microbiome 8, 139. doi: 10.1186/s40168-020-00915-9
Bucci S. J., Scholz F. G., Goldstein G., Meinzer F. C., Franco A. C., Campanello P. I., et al. (2006). Nutrient availability constrains the hydraulic architecture and water relations of savannah trees. Plant Cell Environ. 29. doi: 10.1111/j.1365-3040.2006.01591.x
Bucci S. J., Scholz F. G., Iogna P. A., Goldstein G. (2011). Water economy of woody species from the Patagonian steppes. Ecologia Austral 21:43–60.
Burkhardt J., Basi S., Pariyar S., Hunsche M. (2012). Stomatal penetration by aqueous solutions – an update involving leaf surface particles. New Phytol. 196, 774–787. doi: 10.1111/j.1469-8137.2012.04307.x
Cappelletti M., Perazzolli M., Antonielli L., Nesler A., Torboli E., Bianchedi P. L., et al. (2016). Leaf treatments with a protein-based resistance inducer partially modify phyllosphere microbial communities of grapevine. Front. Plant Sci. 7. doi: 10.3389/fpls.2016.01053
Carbonell Silletta L., Cavallaro A., Pereyra D. A., Askenazi J. O., Goldstein G., Scholz F. G., et al. (2022). Soil respiration and N-mineralization processes in the Patagonian steppe are more responsive to fertilization than to experimental precipitation increase. Plant Soil 479, 405–422. doi: 10.1007/s11104-022-05531-0
Carbonell-Silletta L., Scholz F. G., Burek A., Villa V. D., Cavallaro A., Askenazi J. O., et al. (2024). Nitrogen rather than water availability limits aboveground primary productivity in an arid ecosystem: Substantial differences between grasses and shrubs. Ecohydrology 17, e2636. doi: 10.1002/eco.2636
Cavallaro A., Bucci S. J., Carbonell Silletta L. M., Pereyra D. A., Arias N. S., Goldstein G., et al. (2020a). Contribution of leaf water uptake to transpiration and water status in arid ecosystems. Acta Hortic. 1300. doi: 10.17660/ActaHortic.2020.1300.18
Cavallaro A., Carbonell Silleta L., Pereyra D. A., Goldstein G., Scholz F. G., Bucci S. J. (2020b). Foliar water uptake in arid ecosystems: seasonal variability and ecophysiological consequences. Oecologia 193. doi: 10.1007/s00442-020-04673-1
Cavallaro A., Carbonell-Silletta L., Askenazi J. O., Goldstein G., Bucci S. J., Scholz F. G. (2023). Phenotypic plasticity in leaf traits in response to experimental precipitation increase: Wettability, foliar water uptake and gas exchange. Ecohydrology 16, e2573. doi: 10.1002/eco.2573
Cavallaro A., Carbonell-Silletta L., Burek A., Goldstein G., Scholz F. G., Bucci S. J. (2022). Leaf surface traits contributing to wettability, water interception and uptake of above-ground water sources in shrubs of Patagonian arid ecosystems. Ann. Bot. 130, 409–418. doi: 10.1093/aob/mcac042
Chaudhry V., Runge P., Sengupta P., Doehlemann G., Parker J. E., Kemen E. (2021). Shaping the leaf microbiota: plant-microbe-microbe interactions. J. Exp. Bot. 72, 36–56. doi: 10.1093/jxb/eraa417
Chin A. R. O., Guzmán-Delgado P., Görlich A., HilleRisLambers J. (2023a). Towards multivariate functional trait syndromes: Predicting foliar water uptake in trees. Ecology 104, e4112. doi: 10.1002/ecy.4112
Chin A. R. O., Guzmán-Delgado P., Kerhoulas L. P., Zwieniecki M. A. (2023b). Acclimation of interacting leaf surface traits affects foliar water uptake. Tree Physiol. 43, 418–429. doi: 10.1093/treephys/tpac120
Costa L. S., De Moura C. O., Bucci S. J., Sonsin-Oliveira J., Gomes S. M., Da Cunha Bustamante M. M. (2021). Nutrient enrichment changes water transport structures of savanna woody plants. Environ. Res. Lett. 16. doi: 10.1088/1748-9326/abe6c5
Darlison J., Mogren L., Rosberg A. K., Grudén M., Minet A., Liné C., et al. (2019). Leaf mineral content govern microbial community structure in the phyllosphere of spinach (Spinacia oleracea) and rocket (Diplotaxis tenuifolia). Sci. Total Environ. 675, 501–512. doi: 10.1016/j.scitotenv.2019.04.254
De Mandal S., Jeon J. (2023). Phyllosphere microbiome in plant health and disease. Plants (Basel Switzerland) 12. doi: 10.3390/plants12193481
Derridj S. (1996). “Nutrients on the leaf surface,” in Aerial Plant Surface Microbiology. Eds. Morris C. E., Nicot P. C., Nguyen-The C. (New York: Springer US), 25–42. doi: 10.1007/978-0-585-34164-4_2
Drummond J. S., Rosado B. H. P. (2022). On the role of the phyllosphere community in leaf wettability and water shedding. J. Exp. Bot. 73, 7204–7207. doi: 10.1093/jxb/erac350
Duan Y., Siegenthaler A., Skidmore A. K., Chariton A. A., Laros I., Rousseau M., et al. (2024). Forest top canopy bacterial communities are influenced by elevation and host tree traits. Environ. Microbiome 19, 21. doi: 10.1186/s40793-024-00565-6
Durante M., Maseda P. H., Fernández R. J. (2011). Xylem efficiency vs. safety: Acclimation to drought of seedling root anatomy for six Patagonian shrub species. J. Arid Environments 75, 397–402. doi: 10.1016/j.jaridenv.2010.12.001
Eller C. B., Lima A. L., Oliveira R. S. (2013). Foliar uptake of fog water and transport belowground alleviates drought effects in the cloud forest tree species, Drimys brasiliensis (Winteraceae). New Phytol. 199, 151–162. doi: 10.1111/nph.2013.199.issue-1
Fernández V., Almonte L., Bahamonde H. A., Galindo-Bernabeu A., Sáenz-Arce G., Colchero J. (2024). Chemical and structural heterogeneity of olive leaves and their trichomes. Commun. Biol. 7, 352. doi: 10.1038/s42003-024-06053-4
Fernández V., Bahamonde H. A., Javier Peguero-Pina J., Gil-Pelegrín E., Sancho-Knapik D., Gil L., et al. (2017). Physico-chemical properties of plant cuticles and their functional and ecological significance. J. Exp. Bot. 68, 5293–5306. doi: 10.1093/jxb/erx302
Fernández V., Sancho-knapik D., Guzmán P., Peguero-pina J. J., Gil L., Karabourniotis G., et al. (2014). Wettability, polarity, and water absorption of holm oak leaves : effect of leaf side and age. Plant Physiology 166, 168–180. doi: 10.1104/pp.114.242040
Flexas J., Carriquí M., Nadal M. (2018). Gas exchange and hydraulics during drought in crops: who drives whom? J. Exp. Bot. 69, 3791–3795. doi: 10.1093/jxb/ery235
Fradera-Soler M., Mravec J., Schulz A., Taboryski R., Jørgensen B., Grace O. M. (2024). Revisiting an ecophysiological oddity: Hydathode-mediated foliar water uptake in Crassula species from southern Africa. Plant Cell Environ. 47, 460–481. doi: 10.1111/pce.14743
Grinberg M., Orevi T., Steinberg S., Kashtan N. (2019). Bacterial survival in microscopic surface wetness. ELife 8. doi: 10.7554/eLife.48508
Guzmán-Delgado P., Laca E., Zwieniecki M. A. (2021). Unravelling foliar water uptake pathways: The contribution of stomata and the cuticle. Plant Cell \& Environ. 44, 1728–1740. doi: 10.1111/pce.14041
Hartman K., Tringe S. G. (2019). Interactions between plants and soil shaping the root microbiome under abiotic stress. Biochem. J. 476, 2705–2724. doi: 10.1042/BCJ20180615
Hayes M. A., Chapman S., Jesse A., O’Brien E., Langley J. A., Bardou R., et al. (2020). Foliar water uptake by coastal wetland plants: A novel water acquisition mechanism in arid and humid subtropical mangroves. J. Ecol. 108, 2625–2637. doi: 10.1111/1365-2745.13398
Holder C. D. (2007). Leaf water repellency of species in Guatemala and Colorado ( USA ) and its significance to forest hydrology studies. J. Hydrology 336, 147–154. doi: 10.1016/j.jhydrol.2006.12.018
Karlsson I., Friberg H., Kolseth A.-K., Steinberg C., Persson P. (2017). Organic farming increases richness of fungal taxa in the wheat phyllosphere. Mol. Ecol. 26, 3424–3436. doi: 10.1111/mec.14132
Kembel S. W., O’Connor T. K., Arnold H. K., Hubbell S. P., Wright S. J., Green J. L. (2014). Relationships between phyllosphere bacterial communities and plant functional traits in a neotropical forest. Proc. Natl. Acad. Sci. United States America 111, 13715–13720. doi: 10.1073/pnas.1216057111
Knoll D., Schreiber L. (2000). Plant-microbe interactions: wetting of ivy (Hedera helix L.) leaf surfaces in relation to colonization by epiphytic microorganisms. Microbial Ecol. 40, 33–42. doi: 10.1007/s002480000012
Li W., Jiang L., Zhang Y., Teng D., Wang H., Wang J., et al. (2021). Structure and driving factors of the soil microbial community associated with Alhagi sparsifolia in an arid desert. PloS One 16, e0254065. doi: 10.1371/journal.pone.0254065
Li C., Mo Y., Wang N., Xing L., Qu Y., Chen Y., et al. (2023). The overlooked functions of trichomes: Water absorption and metal detoxication. Plant Cell \& Environ. 46, 669–687. doi: 10.1111/pce.14530
Limm E. B., Simonin K. A., Bothman A. G., Dawson T. E. (2009). Foliar water uptake: A common water acquisition strategy for plants of the redwood forest. Oecologia 161, 449–459. doi: 10.1007/s00442-009-1400-3
Lindow S. E., Brandl M. T. (2003). Microbiology of the phyllosphere. Appl. Environ. Microbiol. 69, 1875–1883. doi: 10.1128/AEM.69.4.1875-1883.2003
Liu J., Zhang W., Liu Y., Zhu W., Yuan Z., Su X., et al. (2023). Differences in phyllosphere microbiomes among different Populus spp. in the same habitat. Front. Plant Sci. 14. doi: 10.3389/fpls.2023.1143878
Losada J. M., Díaz M., Holbrook N. M. (2021). Idioblasts and peltate hairs as distribution networks for water absorbed by xerophilous leaves. Plant Cell Environ. 44, 1346–1360. doi: 10.1111/pce.13985
Losso A., Dämon B., Hacke U., Mayr S. (2023). High potential for foliar water uptake in early stages of leaf development of three woody angiosperms. Physiologia Plantarum 175, e13961. doi: 10.1111/ppl.13961
Matos I. S., Rifai S. W., Gouveia W. F., Oliveras I., Mantuano D., Rosado B. H. P. (2024). A causal trait model for explaining foliar water uptake capacity. J. Vegetation Sci. 35, e13258. doi: 10.1111/jvs.13258
Mina D., Pereira J. A., Lino-Neto T., Baptista P. (2020). Epiphytic and endophytic bacteria on olive tree phyllosphere: exploring tissue and cultivar effect. Microbial Ecol. 80, 145–157. doi: 10.1007/s00248-020-01488-8
Monier J. M., Lindow S. E. (2004). Frequency, size, and localization of bacterial aggregates on bean leaf surfaces. Appl. Environ. Microbiol. 70, 346–355.
Morris C. E., Monier J., Jacques M. (1997). Methods for observing microbial biofilms directly on leaf surfaces and recovering them for isolation of culturable microorganisms. Appl. Environ. Microbiol. 63, 1570–1576. doi: 10.1128/aem.63.4.1570-1576.1997
Ohrui T., Nobira H., Sakata Y., Taji T., Yamamoto C., Nishida K., et al. (2007). Foliar trichome- and aquaporin-aided water uptake in a drought-resistant epiphyte Tillandsia ionantha Planchon. Planta 227, 47–56. doi: 10.1007/s00425-007-0593-0
Pan Z.-L., Guo W., Zhang Y.-J., Schreel J. D. M., Gao J.-Y., Li Y.-P., et al. (2021). Leaf trichomes of Dendrobium species (epiphytic orchids) in relation to foliar water uptake, leaf surface wettability, and water balance. Environ. Exp. Bot. 190, 104568. doi: 10.1016/j.envexpbot.2021.104568
Papierowska E., Szporak-Wasilewska S., Szewińska J., Szatyłowicz J., Debaene G., Utratna M. (2018). Contact angle measurements and water drop behavior on leaf surface for several deciduous shrub and tree species from a temperate zone. Trees 32, 1253–1266. doi: 10.1007/s00468-018-1707-y
Pepper I. L., Gerba C. P. (2015). “Chapter 8 - environmental sample collection and processing,” in Eds. Pepper I. L., Gerba C. P., Gentry T. E. (Oxford: Elsevier, Academic Press), 157–175. doi: 10.1016/B978-0-12-394626-3.00008-9
Pereyra D. A., Bucci S. J., Arias N. S., Ciano N., Cristiano P. M., Goldstein G., et al. (2017). Grazing increases evapotranspiration without the cost of lowering soil water storages in arid ecosystems. Ecohydrology 10. doi: 10.1002/eco.1850
Quan K., Hou J., Zhang Z., Ren Y., Peterson B. W., Flemming H.-C., et al. (2022). Water in bacterial biofilms: pores and channels, storage and transport functions. Crit. Rev. Microbiol. 48, 283–302. doi: 10.1080/1040841X.2021.1962802
Rosado B. H. P., Almeida L. C. (2020). The importance of phyllosphere on foliar water uptake. Trends Plant Sci. 25, 1058–1060. doi: 10.1016/j.tplants.2020.09.002
Rosado B. H. P., Almeida L. C., Alves L. F., Lambais M. R., Oliveira R. S. (2018). The importance of phyllosphere on plant functional ecology: a phyllo trait manifesto. New Phytol. 219, 1145–1149. doi: 10.1111/nph.15235
Rosado B. H. P., Holder C. D. (2013). The signi fi cance of leaf water repellency in ecohydrological research : a review. Ecohydology 161, November 2012, 150–161. doi: 10.1002/eco.1340
Roth-Nebelsick A., Ebner M., Miranda T., Gottschalk V., Voigt D., Gorb S., et al. (2012). Leaf surface structures enable the endemic Namib desert grass Stipagrostis sabulicola to irrigate itself with fog water. J. R. Soc. Interface 9, 1965–1974. doi: 10.1098/rsif.2011.0847
Roth-Nebelsick A., Hacke U. G., Voigt D., Schreiber S. G., Krause M. (2023). Foliar water uptake in Pinus species depends on needle age and stomatal wax structures. Ann. Bot. 131, 287–300. doi: 10.1093/aob/mcac141
Scholz F. G., Bucci S. J., Goldstein G., Meinzer F. C., Franco A. C., Miralles-Wilhelm F. (2007). Removal of nutrient limitations by long-term fertilization decreases nocturnal water loss in savanna trees. Tree Physiol. 27. doi: 10.1093/treephys/27.4.551
Schönherr J. (2006). Characterization of aqueous pores in plant cuticles and permeation of ionic solutes. J. Exp. Bot. 57, 2471–2491. doi: 10.1093/jxb/erj217
Schreel J. D. M., Steppe K. (2019). Foliar water uptake changes the world of tree hydraulics. NPJ Climate Atmospheric Sci. 2, 1. doi: 10.1038/s41612-018-0060-6
Schreel J. D. M., Steppe K. (2020). Foliar water uptake in trees: negligible or necessary? Trends Plant Sci. 25, 590–603. doi: 10.1016/j.tplants.2020.01.003
Schreiber L., Franke R., Hartmann K. D., Ranathunge K., Steudle E. (2005). The chemical composition of suberin in apoplastic barriers affects radial hydraulic conductivity differently in the roots of rice (Oryza sativa L. cv. IR64) and corn (Zea mays L. cv. Helix). J. Exp. Bot. 56, 1427–1436. doi: 10.1093/jxb/eri144
Sohrabi R., Paasch B. C., Liber J. A., He S. Y. (2023). Phyllosphere microbiome. Annu. Rev. Plant Biol. 74, 539–568. doi: 10.1146/annurev-arplant-102820-032704
Song W., Chen S., Zhou Y., Wu B., Zhu Y., Lu Q., et al. (2015). Contrasting diel hysteresis between soil autotrophic and heterotrophic respiration in a desert ecosystem under different rainfall scenarios. Sci. Rep. 5, 16779. doi: 10.1038/srep16779
Soriano A., Sala O. E., Perelman S. B. (1994). Patch structure and dynamics in a Patagonian arid steppe. Vegetatio 111, 127–135. doi: 10.1007/BF00040332
Thapa S., Prasanna R., Ranjan K., Velmourougane K., Ramakrishnan B. (2017). Nutrients and host attributes modulate the abundance and functional traits of phyllosphere microbiome in rice. Microbiological Res. 204, 55–64. doi: 10.1016/j.micres.2017.07.007
Tian J., Li Z., Wang H., Lv G., Li W., Wang H., et al. (2024). Physiological responses and ecological benefits of water uptake by populus euphratica leaves in arid areas. Forests 15. doi: 10.3390/f15030430
Tie J., Gao M., Huang Y., Li K., Wang H. (2023). Factors influencing wettability and surface/interface mechanics of plant surfaces: a review. Front. Materials 10. doi: 10.3389/fmats.2023.1311735
Trivedi P., Delgado-Baquerizo M., Jeffries T. C., Trivedi C., Anderson I. C., Lai K., et al. (2017). Soil aggregation and associated microbial communities modify the impact of agricultural management on carbon content. Environ. Microbiol. 19, 3070–3086. doi: 10.1111/1462-2920.13779
Vogel M. A., Mason O. U., Miller T. E. (2021). Composition of seagrass phyllosphere microbial communities suggests rapid environmental regulation of community structure. FEMS Microbiol. Ecol. 97. doi: 10.1093/femsec/fiab013
Vokou D., Vareli K., Zarali E., Karamanoli K., Constantinidou H.-I. A., Monokrousos N., et al. (2012). Exploring biodiversity in the bacterial community of the Mediterranean phyllosphere and its relationship with airborne bacteria. Microbial Ecol. 64, 714–724. doi: 10.1007/s00248-012-0053-7
Vorholt J. A. (2012). Microbial life in the phyllosphere. Nat. Rev. Microbiol. 10, 828–840. doi: 10.1038/nrmicro2910
Wang H., Li Z., Yang J. (2023). Effects of leaf hydrophilicity and stomatal regulation on foliar water uptake capacity of desert plants. Forests 14. doi: 10.3390/f14030551
Waseem M., Nie Z.-F., Yao G.-Q., Hasan M., Xiang Y., Fang X.-W. (2021). Dew absorption by leaf trichomes in Caragana korshinskii: An alternative water acquisition strategy for withstanding drought in arid environments. Physiologia Plantarum 172, 528–539. doi: 10.1111/ppl.13334
Whipps J. M., Hand P., Pink D., Bending G. D. (2008). Phyllosphere microbiology with special reference to diversity and plant genotype. J. Appl. Microbiol. 105, 1744–1755. doi: 10.1111/j.1365-2672.2008.03906.x
Xiong C., Lu Y. (2022). Microbiomes in agroecosystem: Diversity, function and assembly mechanisms. Environ. Microbiol. Rep. 14, 833–849. doi: 10.1111/1758-2229.13126
Yadav R. K. P., Karamanoli K., Vokou D. (2005). Bacterial colonization of the phyllosphere of mediterranean perennial species as influenced by leaf structural and chemical features. Microbial Ecol. 50, 185–196. doi: 10.1007/s00248-004-0171-y
Yahdjian L., Gherardi L., Sala O. E. (2011). Nitrogen limitation in arid-subhumid ecosystems : A meta-analysis of fertilization studies. J. Arid Environments 75, 675–680. doi: 10.1016/j.jaridenv.2011.03.003
Yokoyama G., Yasutake D., Minami K., Kimura K., Marui A., Yueru W., et al. (2021). Evaluation of the physiological significance of leaf wetting by dew as a supplemental water resource in semi-arid crop production. Agric. Water Manage. 255, 106964. doi: 10.1016/j.agwat.2021.106964
Zhu K., Wang A., Wu J., Yuan F., Guan D., Jin C., et al. (2020). Effects of nitrogen additions on mesophyll and stomatal conductance in Manchurian ash and Mongolian oak. Sci. Rep. 10, 10038. doi: 10.1038/s41598-020-66886-x
Keywords: foliar water uptake, phyllosphere microbiota, patagonian steppe, water drop adhesion, wettability, nitrogen, plant-microbe interactions
Citation: Palmeri MA, Arias NS, Parra G, Carbonell-Silletta L, Silva RA, Goldstein G, Scholz FG and Bucci SJ (2024) Foliar water uptake and phyllosphere microbe colonization increase under higher soil nitrogen availability. Front. Plant Physiol. 2:1457037. doi: 10.3389/fphgy.2024.1457037
Received: 30 June 2024; Accepted: 13 November 2024;
Published: 02 December 2024.
Edited by:
Yasuyuki Kawaharada, Iwate University, JapanReviewed by:
Anish Malladi, University of Georgia, United StatesRamya Enganti, Indiana University, United States
Copyright © 2024 Palmeri, Arias, Parra, Carbonell-Silletta, Silva, Goldstein, Scholz and Bucci. This is an open-access article distributed under the terms of the Creative Commons Attribution License (CC BY). The use, distribution or reproduction in other forums is permitted, provided the original author(s) and the copyright owner(s) are credited and that the original publication in this journal is cited, in accordance with accepted academic practice. No use, distribution or reproduction is permitted which does not comply with these terms.
*Correspondence: N. S. Arias, bnNfYXJpYXNAeWFob28uY29tLmFy