- 1UMRT INRAE 1158 BioEcoAgro – BIOPI Biologie des Plantes et Innovation, Université de Picardie Jules Verne, Amiens, France
- 2INRAE, AgroParisTech, Institut Jean-Pierre Bourgin(IJPB), Université Paris-Saclay, Versailles, France
- 3Centre de Ressources Régionales en Biologie Moléculaire(CRRBM), Université de Picardie Jules Verne, Amiens, France
Plant cell adhesion is essential for development and stress response and is mediated by the deposition of pectin-rich middle lamella between cell walls. However, the precise control mechanism of cell adhesion remains unclear. The qua2-1 and esmd1-1 mutants provide a better understanding of this process and suggest a signaling pathway triggering the loss and restoration of adhesion via cell wall modifications. This study aims at a better characterization of the production of endogenous oligogalacturonides(OGs) and cell wall structural modifications in the control of cell adhesion in Arabidopsis. From dark-grown hypocotyls of wild type, qua2-1, esmd1-1, and qua2-1/esmd1-1 mutants, we identified seven distinct endogenous OGs, that varied in their degree of polymerization and extent of substitution and in their abundance. The structure of homogalacturonans were further analyzed by enzymatic fingerprint, indicating a change in esterification patterns. Expression analysis of genes encoding pectin-modifying enzymes, including PME, PMEI, and PAE showed significant variations depending on genotypes. Gene expression levels correlated with changes in the structure of homogalacturonans and cell adhesion phenotypes. This study suggests the involvement of a feedback loop between endogenous OGs, the fine-tuning of homogalacturonans structure, and the gene expression of pectin remodeling enzymes in controlling cell adhesion.
Introduction
Cell adhesion plays a crucial role in plant development and in response to stress (Somerville et al., 2004). The adhesion between adjacent plant cells is facilitated by the deposition of a pectin-rich middle lamella (Daher and Braybrook, 2015). The middle lamella primarily consists of homogalacturonans (HGs), whose degree of methyl and O-acetyl esterification is regulated by cell wall-localized proteins such as pectin methylesterases (PMEs), pectin methylesterase inhibitors (PMEIs), and pectin acetylesterases (PAEs), all encoded by large multigenic families in Arabidopsis (Pelloux et al., 2007; Philippe et al., 2017). While some molecular regulators involved in cell adhesion have been identified (Atakhani et al., 2022), the mechanism by which plants control and maintain cell adhesion in response to changes in cell wall chemistry remains poorly understood. Studies on the Arabidopsis quasimodo2 (qua2) mutant, which is affected in a pectin methyltransferase gene and exhibits cellular adhesion defects and reduced amount of HGs but not in their degree of methyl-esterification (Mouille et al., 2007; Du et al., 2020b). Furthermore, this mutant exhibits impaired cellulose and microtubule alignments, likely associated with mechanical defects in tissue tension due to stress-strain during cell growth (Zang et al., 2021; Kelly-Bellow et al., 2023). The gradual loss of cell adhesion suggests that a biological process is necessary, at least for the separation of the middle lamella. Other mutants, such as friable1, a rhamnosyl transferase defective mutant, demonstrate altered cell adhesion without any changes in cellulose or HGs contents (Neumetzler et al., 2012; Wachananawat et al., 2020). Interestingly, the esmeralda1 mutant (esmd1-1), carrying a point mutation in a putative O-fucosyltransferase, rescues quasimodo1-1 (Bouton et al., 2002), friable1 & quasimodo2-1 (qua2-1) phenotypes, without restoring HG content but rather by altering the expression of the pectin response gene FAD-LINKED OXIDOREDUCTASE, FADLOX (Denoux et al., 2008; Verger et al., 2016). These findings suggest that ESMERALDA plays a central role in modulating a signal related to pectin biosynthesis and/or a pectin-mediated signal, influencing the state of cell adhesion. It has been hypothesized that qua2-1 initiates a signal leading to the loss of cell adhesion, and esmd1 may impact this pathway and restore cell adhesion through modifications in the cell wall (Verger et al., 2016). Oligogalacturonides (OGs) are considered as endogenous elicitors that activate plant immunity and control developmental processes (Ferrari et al., 2013; Lin et al., 2022) and the prime candidate that could control cell adhesion in Arabidopsis (Verger et al., 2016). OGs are oligomers released from plant cell walls upon partial degradation of homogalacturonans by polygalacturonases (PGs) and or polysaccharide lyases (PLLs) that can activate various signaling pathways when perceived by cell wall receptors (Wolf, 2022). However, this signal does not seem to involve the wall-associated kinase receptor (WAK), as the loss of function of five WAK did not affect esmd1’s ability to suppress the cell adhesion defect in qua2-1 (Kohorn et al., 2021). Therefore, identifying a potential OG signal modified by the esmd1 mutation in qua2-1, together with analyzing the fine structure of pectins by oligoprofiling will advance our understanding of the regulation of cell adhesion in plants.
Results
Are endogenous oligogalacturonides involved in cell adhesion?
Since endogenous OGs could play a role in controlling cell adhesion, we extracted endogenous OGs from dark-grown hypocotyls and analyzed them by high-performance size exclusion chromatography (HP-SEC) coupled with mass spectrometry (Voxeur et al., 2019). We identified seven different OGs with degrees of polymerization (DP) ranging from 2 to 5, decorated with various methylation and oxidation statuses, whose amount differed in Col-0, qua2-1, esmd1-1, and qua2-1/esmd1-1 mutants (Figure 1). Notably, all the extracted OGs were less abundant in qua2-1, with a 50% to 30% decrease compared to the wild type, depending on the OG considered. However, the average quantity of most OGs was partially or fully restored by the esmd1-1 mutation, excepted GalA2Ox, and in some cases, the double mutant exhibited even higher levels than the wild type. Statistically, only GalA4Me and GalA4Me2 were significantly more abundant in esmd1-1 and in the double mutant qua2-1/esmd1-1 compared to qua2-1 (Figure 1). As there was no difference in the identity of endogenous OGs, our results suggest that their overall amount is of importance to mediate the recovery of cell adhesion in qua2-1/esmd1-1.
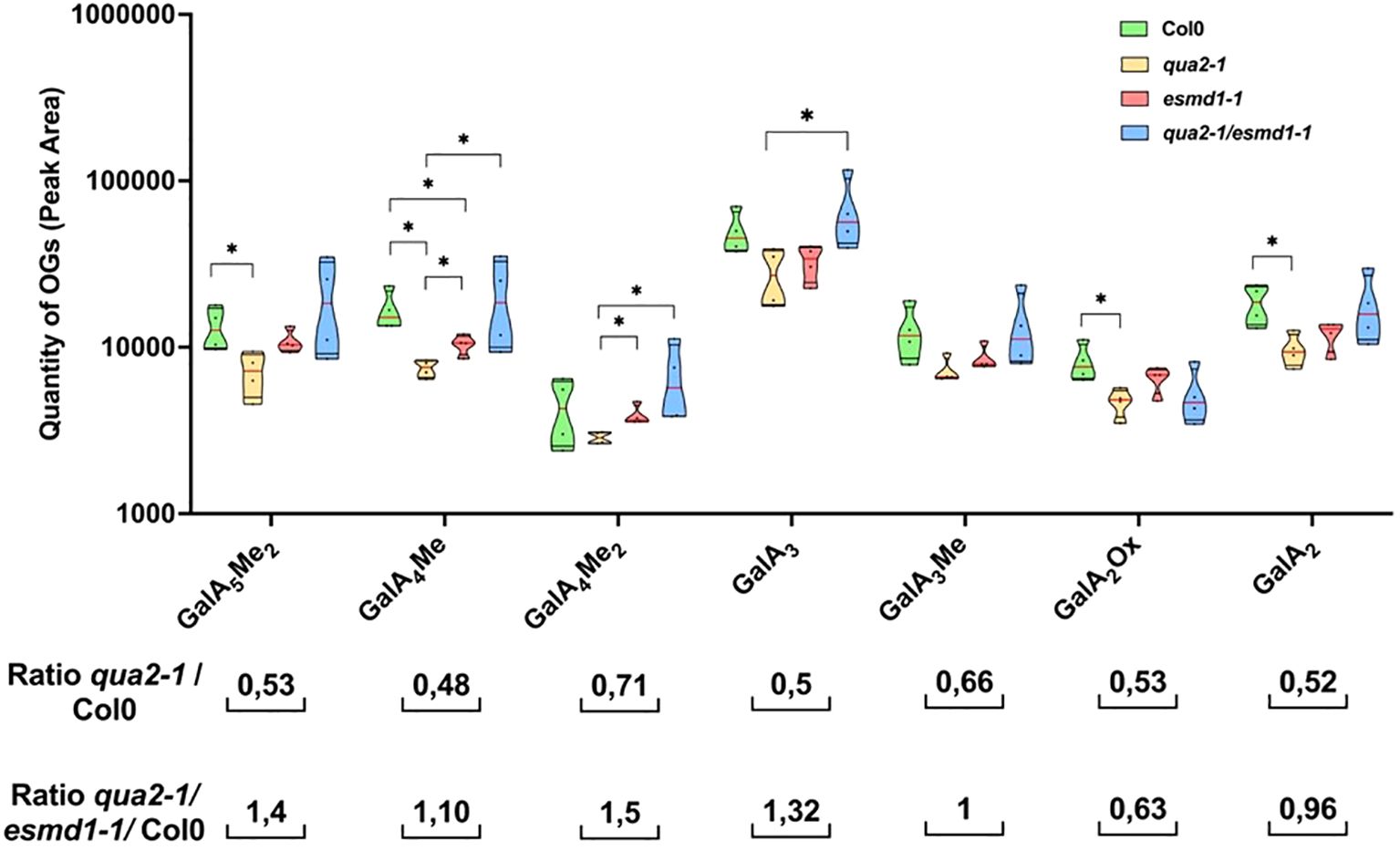
Figure 1. Endogenous OGs. Truncated violin plot of peak area of endogenous OGs of 150 4-day-old dark grown dissected hypocotyls of Col-0, qua2-1, qua2-1/esmd1-1 and esmd1-1. Oligogalacturonides (OGs) are named as follow: GalAxMey where the numbers x and y in subscripts indicates the degree of polymerization and the number of methylations respectively. GalA, galacturonic acid; Me, methyl ester group; Ox, oxidation. Red line represents the median, black line the quartiles, black dot represents biological replicate (n = 4 biological replicates per genotype). *, P < 0.05, Mann & Whitney test.
As endogenous OGs production is related to the digestibility of HG, which is directly influenced by the degrees/patterns of methylesterification as well as the action of various polygalacturonases, pectin and pectate lyases, we further investigated the fine structure of homogalacturonans in the different genetic backgrounds.
Does the esmeralda1 mutation alters homogalacturonans pattern?
To explore the structure of digestible HGs in different genotypes, we enzymatically digested dried cell walls of dark-grown seedlings of the various genotypes with a commercial endo-polygalacturonase from Aspergillus aculeatus. The released fragments were then analyzed by high-performance size exclusion chromatography (HP-SEC) coupled with mass spectrometry. We identified 92 HG fragments, including monomers, with distinct degrees of methyl and O-acetyl esterification, some of which were oxidized. Our results revealed that, independently of the total amount of HGs hydrolyzed by trifluoroacetic acid (TFA)(Supplementary Figure S1B), less HG fragments were released from the total dried cell wall fractions in qua2-1 and the double mutant compared to the wild type (Supplementary Figure S1C). Conversely, this digestible fraction of HG, relative to the total amount of HGs hydrolyzed by TFA (Figure 2A), remains unchanged in the mutants compared to the wild type, despite a slight decrease being observed. Since the qua2-1/esmd1-1 double mutant shows a similar pectin defect to qua2-1 (Verger et al., 2016), this suggests that the fraction of HGs sensitive to PGs digestion, relative to the total amount of HGs, is not the main factor responsible of the restoration of adhesion, as it is maintained across the different genotypes (Figure 2A).
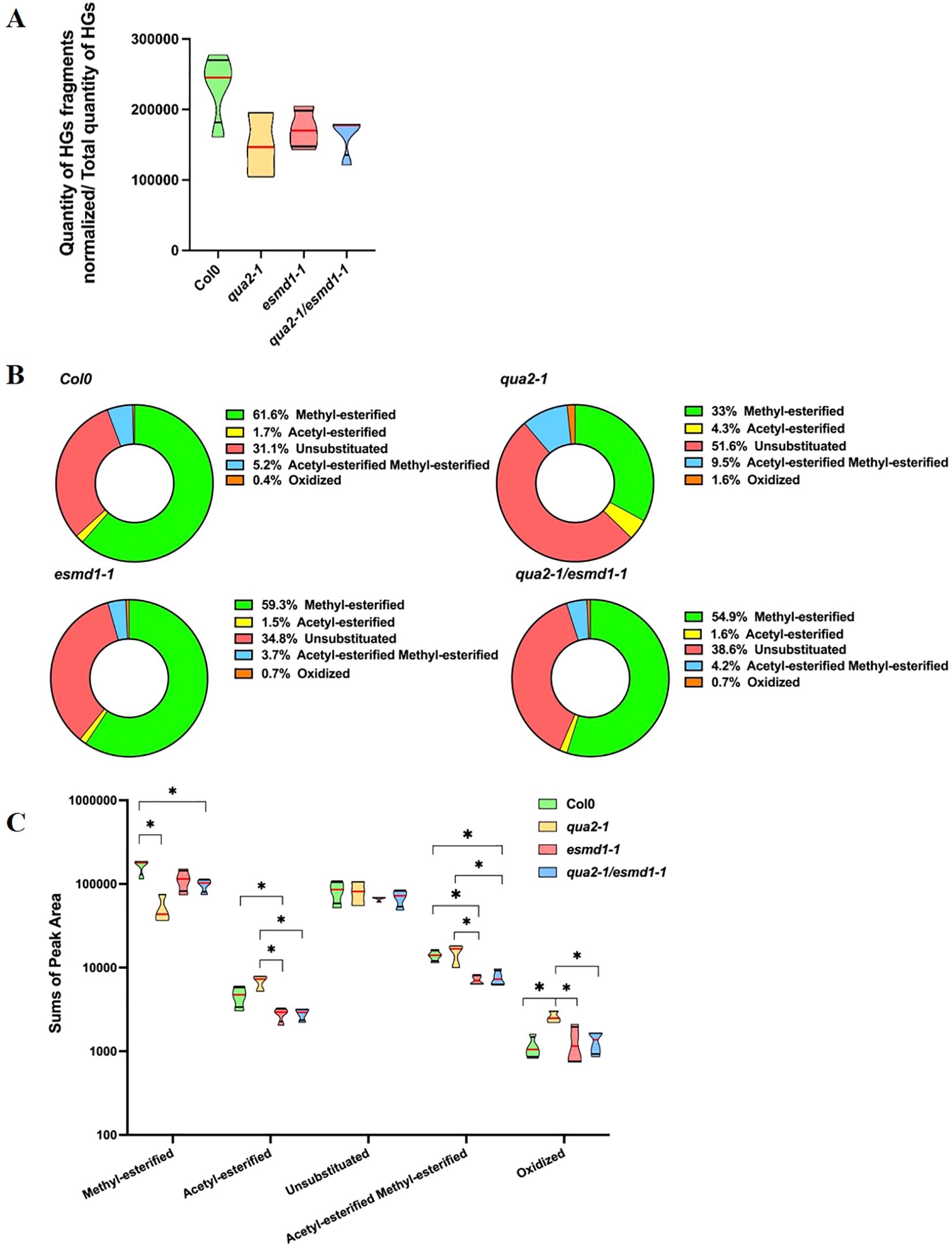
Figure 2. Fine structure of homogalacturonans. (A) Truncated violin plot of the quantity of HGs fragments & monomers released by PG Aspergillus aculeatus digestion of dried cell wall seedlings 4 days dark grown of Col-0, qua2-1, qua2-1/esmd1-1 and esmd1-1. The quantity was calculated for 1 mg of dried cell wall and normalized by the GalA released by TFA hydrolysis. The quantity of GalA hydrolyzed for 1 mg of dried cell wall of same samples was analyzed by GC/MS (Supplementary Figure S1A) and subtracted to the equivalent quantity of rhamnose to get the quantity of HGs. HGs fragments were quantified by LC/MS-MS according to Voxeur et al., 2019. Red line represents the median, black line the quartiles (n ≤ 4 biological replicates per genotype). Mann-Whitney test. (B) Relative content of HGs fragments family released by Aspergillus aculeatus PG digestion of dried cell wall of 4-days-old dark grown of seedlings Col-0, qua2-1, qua2-1/esmd1-1 and esmd1-1 (n ≤ 4 biological replicates per genotype). (C) Truncated violin plot of sums of the quantity of the classes of HGs fragments released by Aspergillus aculeatus PG digestion of dried cell wall of 4-days-old dark grown seedlings of Col-0, qua2-1, qua2-1/esmd1-1 and esmd1-1. HGs fragments were quantified by LC/MS according to Voxeur et al., 2019. Red line represents the median, black line the quartiles, (n ≤ 4 biological replicates per genotype). *, P < 0.058, Mann-Whitney test.
The identified OGs were grouped into five different categories based on their substitution and oxidation state, reflecting the homogalacturonans pattern. In qua2-1, there was a relative decrease (50%) in the amount of methylesterified fragments released compared to the wild type, and to esmd1-1 (Figure 2B). In contrast, all other fragment categories were 1.5- to 4-fold more abundant in qua2-1. This fingerprint analysis revealed a previously unidentified pectin modification in qua2-1, indicating a decrease in methyl esterified HGs sensitive to polygalacturonase digestion. The proportions of HGs pattern in the qua2-1/esmd1-1 double mutant suggest that esmd1-1 restores the relative content of the five fragment groups, resulting in a pattern almost indistinguishable from that of the wild type (Figure 2B), without recovering HGs quantity (Verger et al., 2016).
Further examination of the quantity of each of the five groups of released HG fragments revealed that the oxidized fragments were more abundant in qua2-1 compared to the wild type (Figure 2C). In contrast, methyl-esterified fragments were less abundant in qua2-1. Except for the oxidized and unsubstituted fragments, the quantities of the other three groups were altered in the qua2-1/esmd1-1 double mutant but different from qua2-1. It is worth mentioning that the pattern of HG substitution was also altered in the single esmd1-1 mutant, where the digestion of its cell wall released fewer acetyl and acetyl-methyl-esterified fragments compared to the wild type (Figure 2C).
Among the 48 HG fragments significantly different between the wild type and qua2-1, 30 were more abundant in the wild type, while 18 were more abundant in qua2-1 (Supplementary Figure S2A). In the wild type, the accumulated fragments exhibited moderate degree of methyl-esterification (2 to 5) with or without mono-acetyl-esterification, whereas in qua2-1, they were highly methyl-esterified (5 to 10), with or without acetyl-esterification (1-3). Thus, the observed modifications in the methyl-esterified HG pattern in qua2-1 suggest a defect in PMEs expression or activity.
The qua2 versus double mutant comparison reveals 46 HGs fragment significantly different (Supplementary Figure S2C). Among them, 12 methyl-esterified (*), 2 acetyl-esterified (°) and 2 methyl-acetyl-esterified (•) fragments were specific to esmd1-1 (Supplementary Figure S2C). The 34 remaining fragments display the same profile in the qua2 versus wild-type comparison (Supplementary Figure S2A).
Both esmd1-1 HGs pattern features contribute to the restoration of the proportion of digestible methyl-esterified pattern in qua2-1 background (Figure 2B). These results also indicate that the esmd1-1 mutation reduces the acetyl-esterified pattern in the qua2-1 background, suggesting a higher activity of PAEs that restore the proportion of acetyl-esterified and methyl- acetyl-esterified patterns respectively (Figure 2B). The indirect regulation of HG methyl and O-acetyl esterification patterns by the O-fucosyltransferase ESMERALDA1 is further supported by the comparison between esmd1-1 and the wild type (Supplementary Figure S2B).
Overall, giving that the fraction of HGs sensitive to PGs digestion relative to the total amount of HGs remains unchanged, it seems that esmd1-1 mutation restores the proportion of methyl and/or acetylesterified pattern as well as the oxidized categories of HGs in the double mutant. This specific modulation of HG pattern including some similarities and divergencies with wild-type.
Additionally, we examined other cell wall polymers. Significant variations were observed in qua2 compared to wild type for galacturonate and arabinose monomers (Supplementary Figure S1A) as previously mentioned (Du et al., 2020a) while minor differences are noted for glucose and xylose (Siupplementary Fgure S1A). Concurrently, we conducted an analysis of both the quantity and structure of xyloglucans and assessed cellulose content in parallel. While a notable enhancement in digestible xyloglucan is noted in qua2 compared to the wild type, as well as in the double mutant. All xyloglucan fragments exhibit a similar proportion/distribution across all genotypes except for a reduction in XXG and XXXG and an increase in XLXG in qua2 (Supplementary Figures S3A, S3B). Concerning cellulose, no alterations in content could be detected in mutants compared to the wild type (Supplementary Figure S3C).
Which factors are responsible for changing the pectin pattern of qua2, qua2/esmd1 and esmd1?
To gain a better understanding of how these modifications of HG pattern occur in the various genotypes, we analyzed pectin modifying enzymes expression. We performed RT-qPCR analysis of the PME, PMEI and PAE multigenic families in four days-old dark-grown seedlings. Among these genes(respectively 66, 76 and 12), only six exhibited significant expression variations across the genotypes (Figure 3). In qua2-1, the expression of PME53 and PME41 were increased compared to the wild type. These changes in PME gene expression may contribute to the loss of cell adhesion in quasimodo2. On the other hand, the expression of PMEI4 and PME35 was repressed in the double mutant and esmd1-1 compared to qua2-1 (Figure 3). These underexpressions may play a role in the control of cell adhesion by the esmeralda1 mutation, restoring the relative content of HG methyl-esterified pattern (Figure 2B, C). Regarding PAEs, two genes exhibited similar expression variations. The expressions of PAE7 and PAE12 seem to be repress in qua2-1 whereas in the double mutant, expressions are similar to the wild type (Figure 3). The restoration of PAE expression supports the rebalanced acetyl-esterified and/or methyl-acetyl-esterified patterns in the double mutant (Figures 2B, C).
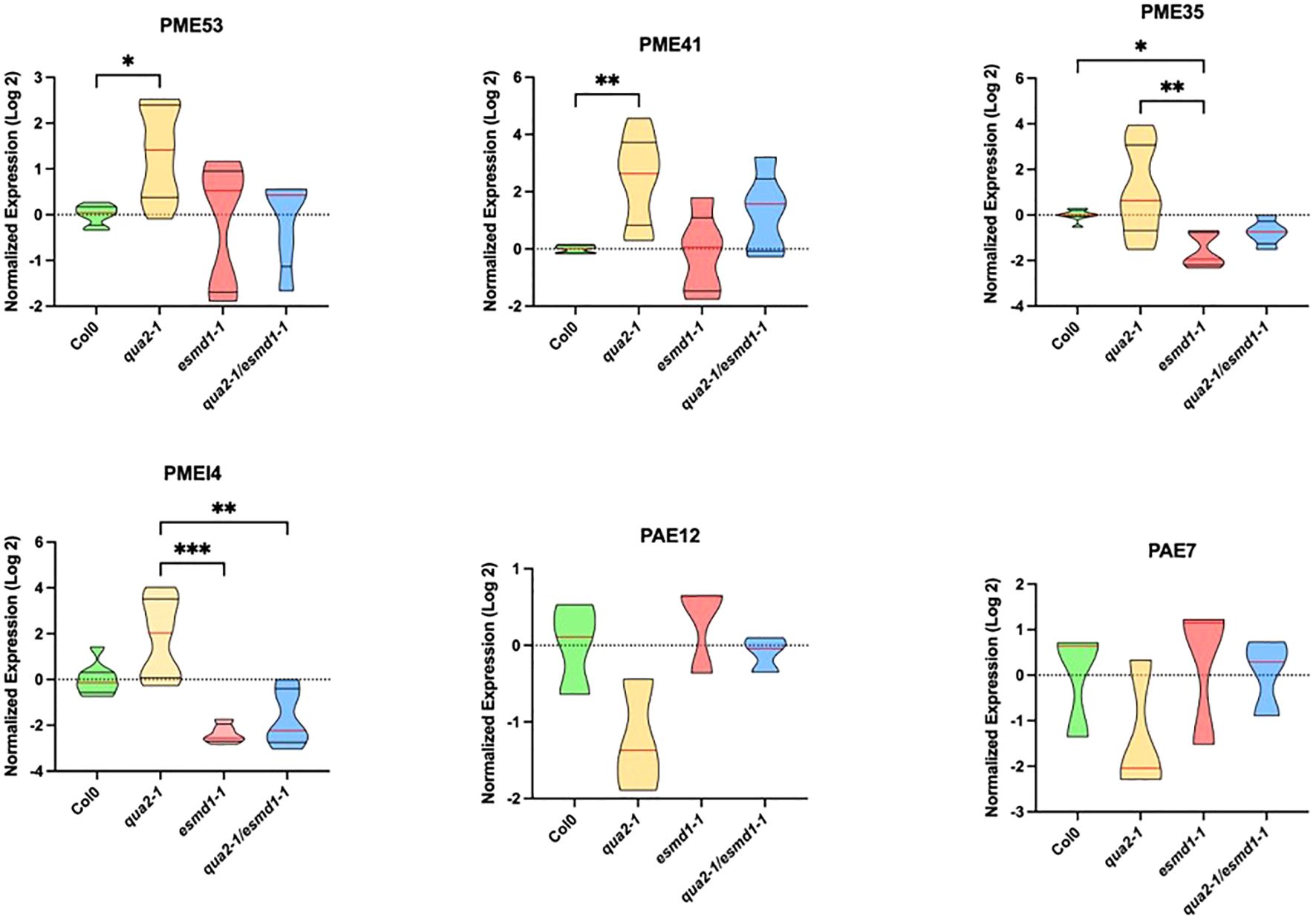
Figure 3. PME/PMEI and PAE expression. Truncated violin plot of Normalized expression levels (log2) of PMEI4, PME, 35, 41, 53 and PAE 7 and 12, from 4-day-old, etiolated seedlings of Col, qua2, esmd1 and qua2/esmd1 by RT-qPCR. APT1 & CLATHRIN were amplified as reference genes. Normalized expression levels (log2) were calculated according to a normalization factor obtained from the 2 reference genes and calibrated to the expression of the wild type (Taylor et al., 2019). Red line represents the median, black line the quartiles (n ≥ 5 biological replicates per genotype for PME and PMEI)(n = 3 biological replicates per genotype for PAE). *, P < 0.05 and **, P < 0.01, ***, P < 0.001, Krustall Wallis test(Dunn’s multiple comparisons test).
We produced and tested those proteins, which potentially contribute to restoring the acetyl- and methylesterified pattern in qua2, by application to the double mutant for 48 hours (Supplementary Figure S4). It resulted in visible cell adhesion defects only for PME35, PME53, and PMEI4 application (Supplementary Figures S4L–N), while no effect was observed on the wild type (Supplementary Figures S4E–G), underscoring their significance in HG pattern management concerning cell adhesion.
Discussion
The mutation in the ESMERALDA1 gene has been shown to restore the phenotypes of a number of mutants with cell adhesion defects (Verger et al., 2016) including the qua1/gaut8 mutant affected in a galacturonosyl transferase, qua2 affected in a pectin methyltransferase and friable1 affected in a rhamnosyltransferase. Hence, ESMERALDA1 emerges as a pivotal and adaptable component in regulating cell adhesion (Verger et al., 2016). The esmd1 mutation results in reduced expression of the FADlox gene, which is known to respond to pectic signals (Denoux et al., 2008), without restoring the qua2 HG content defect (Verger et al., 2016). While WAK receptors are recognized for their affinity to OGs (Kohorn and Kohorn, 2012) and were previously considered prime candidates for mediating the ESMERALDA signal, the loss of function of the five WAKs did not impede esmd1’s ability to suppress cell adhesion defects (Kohorn et al., 2021). Consequently, given the limited understanding of the activity and targeted substrate of this putative O-fucosyltransferase, our study focused on how OG and associated cell wall modifications in esmd1 could enable cell adhesion. OGs stand out as plausible candidates for mediating diverse signals that regulate cell adhesion in Arabidopsis. However, the role of oligosaccharides in plants has primarily been explored through exogenous applications (Branca et al., 1988; Denoux et al., 2008; Sinclair et al., 2017; Voxeur et al., 2019).
In our study, we successfully identified endogenous OGs in dark-grown hypocotyls. Notably, these endogenous OGs appeared to be of low degrees of polymerization and esterification, indicating that the plant may undergo cell wall remodeling through hydrolysis of HG by enzymes of peculiar processivities generating small OGs. Intriguingly, the esmd mutation restored the abundance of nearly all OGs in qua2 to levels equivalent to those found in the wild type. Some of these OGs, previously identified as potential elicitors, such as the trimer of galacturonic acid (GalA) known to trigger a dark-grown signal (Sinclair et al., 2017), and GalA2Ox, an OG that accumulates during later stages of Botrytis cinerea infection, were among those restored. These findings collectively suggest that the overall quantity of endogenous OGs restored by esmd in qua2 may correspond to an eliciting threshold necessary to reinstate or uphold cell adhesion.
Regarding cell wall modifications, we found significant alterations primarily in galacturonic acid and arabinose monomers in qua2, consistent with previous studies (Mouille et al., 2007; Verger et al., 2016; Du et al., 2020a) with lower levels of change observed in xylose and glucose. Consequently, we focused our investigation on the detailed analysis of pectic and xyloglucan patterns to elucidate the fine structure of these polymers. Notably, cellulose quantity did not emerge as a pivotal factor in our model, as no discernible differences were observed among genotypes. However, recent evidence indicates that the qua2 mutant affects both HGs quantity and crystalline cellulose, along with microfibril orientation (Du et al., 2020a).
Despite similar digestible HG content across genotypes, detailed analysis of pectins revealed that esmeralda1 restores a defective proportion of methyl esterified, methyl acetyl esterified, and acetyl esterified HGs in qua2. Recently, Barnes et al., 2022 showed that the global DM is highest in qua2 compared to wild type and double mutant. We would add, that the degree of the DM of qua2 is more affected in the remodeling part of pectin due to its different HG pattern. This restoration occurs through specific modulation of the HG pattern, exhibiting both similarities and differences compared to the wild type, resulting in an overall pattern almost indistinguishable from the wild type (Figure 2B) as mentioned by (Barnes et al., 2022), but without recovering HGs’ quantity (Verger et al., 2016). This modulation potentially leads to the formation of more cross-linkable microdomains interspersed (Barnes et al., 2022) within the homogalacturonan domain or with other cell wall polymers such as arabinogalactan proteins (AGPs), extensins, or cellulose (Valentin et al., 2010; Hijazi et al., 2012; Nunes et al., 2012; Sala et al., 2019, Hijazi et al, 2014), thereby enhancing mechanical equilibrium and facilitating restoration of cell adhesion.
Additionally, qua2 appears to exhibit higher levels of digestible xyloglucan, and the esmeralda1 mutation does not seem to influence this characteristic. It is noteworthy that xyloglucans may contribute to the maintenance of cell adhesion, as demonstrated by immunolocalization studies (Ordaz-Ortiz et al., 2009). Furthermore, xyloglucans play a crucial role in interlacing cellulose microfibrils and are heavily involved in regulating cell wall extension. Loss of xyloglucan can impact the mechanical properties and stability of the microtubule cytoskeleton (Xiao et al., 2016). The fact that esmd1 mutation does not alter the digestible xyloglucan content in qua2 may suggest a combination of maintaining xyloglucan levels and restoring the proportions of the homogalacturonan pattern, thereby facilitating the maintenance of cell adhesion.
To gain deeper insights into how these pectin modifications are orchestrated by the plant, we identified six genes encoding pectin remodeling enzymes whose expression varies significantly and correlates with the observed variations in pectic structure among genotypes. The esmd1 mutation resulted in the underexpression of PMEI4, which is known to regulate pectin de-methyl-esterification during growth acceleration, although not directly affecting the growth process itself (Pelletier et al., 2010). We can hypothesize that the growth restoration by esmd1 in qua2 (Verger et al., 2016) may be linked to the repression of PMEI4, indicating a growth process already initiated in these mutants. Conversely, in the case of qua2, given its reduced hypocotyl length (Mouille et al., 2007; Verger et al., 2016; Du et al., 2020a) overexpression of PMEI4 may be likened to an untriggered or dysfunctional growth acceleration. esmd1 also impact PME35, which plays a role in regulating the mechanical resistance of supporting tissue in Arabidopsis stems (Hongo et al., 2012). The underexpression of these two genes in esmd1 and the double mutant may be necessary to restore the proportion of methylesterified HGs pattern.
In qua2, PME53 and PME41 are overexpressed compared to the wild type. Intriguingly, PME41 is also overexpressed in frb1(Neumetzler et al., 2012) and regulated in various stress conditions (Qu et al., 2011; Lionetti et al., 2012), suggesting that these genes may contribute to an unstable pectic pattern, thereby leading to cell adhesion defects in qua2. With the two PAE whose expression is also deregulated, these PME may contribute to an unstable pectic pattern, thereby leading to cell adhesion defects in qua2. Contrary to the lack of effect of orange PME on cellular adhesion (Barnes et al., 2022), the application of our candidates on the double mutant, in particular PME35, PME53 and PMEI4, underlines their importance in managing the HG pattern for cell adhesion.
In summary, our research sheds light on the involvement of PMEs, PMEIs, and PAEs in cell adhesion. Given that these enzymes belong to extensive multigenic families, conventional reverse genetic methods often yield compensatory expression, resulting in subtle phenotypes. However, by directing our focus toward a specific biological phenomenon such as cell adhesion, we were able to highlight the potential impact of pectin-modifying enzymes.
Thus, we propose that the restoration of cell adhesion in qua2-1 by the esmd1-1 mutation is a multifaceted process involving the production of endogenous OGs, fine-tuning of HGs pattern, and transcriptional modulation of specific pectin-modifying enzymes, suggesting a feedback loop regulation (see Figure 4). Further exploration into the roles of these enzymes and the signaling pathways implicated in this process will enhance our understanding of the mechanisms governing cell adhesion in plants.
Experimental procedures
Plant material and growth conditions
Arabidopsis thaliana seedlings (150 seeds/genotype) were grown in the dark at 21°C on solid medium (Agar 0.8%, Duchefa Biochemie) supplemented with 0.328 g/L Ca(NO3)2 at pH 5.7. This medium was only used for the endogenous OGs analysis. Otherwise, the
Arabidopsis thaliana seedlings were grown on liquid medium H2O ultra-pure, supplemented
with 0.5 g/L of MES (Duchefa M1503.0100) buffered at pH 5.7 by adding KOH 0.04 M to
stabilize it. To synchronize germination, the seeds were cold-treated for 48 hours. After exposure to light for 4 hours, the plates were wrapped in two layers of aluminum foil and cultivated for 92 hours. To not disturb the dark grown condition, all cultures were harvested in a dark room under green light.
Endogenous OGs extraction
Four biological replicates of 150 four-days-old dark-grown seedlings per genotype were dissected in a dark room to isolate the hypocotyls. The 150 hypocotyls were split into 3 tubes (50 in each) and directly frozen. Subsequently, 300 µl of 70% ethanol were added to each tube. The 3 tubes were then incubated in a thermomixer at 80°C for one hour. Ethanol-extracted OGs were pooled in the same tube and dried in a speed vacuum concentrator at room temperature. The resulting pellet was re-suspended in 100 µL of ultra-pure water, centrifuged, and the soluble fraction was transferred in a vial and 10 μl was injected for MS analysis.
Enzymatic fingerprinting of homogalacturonans and xyloglucan
Four biological replicates of approximately 300 four-days-old dark-grown seedlings were harvested in a dark room and placed in tubes containing 1 mL of 96% ethanol. The tubes were incubated in a thermomixer at 80°C for one hour. The previous step with ethanol was repeated for 20 min. The ethanol was then replaced with 1 mL of acetone, and the tubes were incubated in a thermomixer at 25°C for 20 minutes. This acetone wash step was repeated two more times. The hypocotyls were dried in a speed vacuum concentrator at room temperature overnight, and their dry weight was measured(approximately 1-2 mg per sample). The samples were digested with Aspergillus aculeatus endo-polygalacturonase M2 or Endo-cellulase(Megazyme, Bray, Ireland) in 50 mM ammonium acetate buffer pH 5 at 37°C for 18 hours. After inactivating the enzyme by heating, the digested fractions were collected for MS analysis.
OGs characterization and quantification by LC/HRMS analysis
The endogenous OGs and the OGs released from the digestion were analyzed using High-performance size-exclusion chromatography (HP-SEC) coupled with MS. The chromatographic separation was performed on an ACQUITY UPLC Protein BEH SEC Column (125 A°C, 1.7 μm, 4.6 mm x 300 mm) at a flow rate of 400 µL/min and a column oven temperature of 40°C. The MS detection was performed in negative mode with the parameters described in the supporting experimental procedures.
RNA extraction and RT-qPCR analysis
Five biological replicates of approximately 150 seedlings grown in the dark for four days were harvested in a dark room. Total RNA was isolated using the RNeasy Plant Mini Kit (Qiagen) with on-column DNA digestion using Rnase-Free DNase (Qiagen). Two µg of total RNAs were used to synthesize cDNAs using RevertAid H minus reverse transcriptase, according to the manufacturer’s instructions Thermofisher. Transcript levels were assessed by quantitative RT-PCR using a Light Cycler® 480 System (ROCHE), as previously described by (Gutierrez et al., 2009). The expression levels of the target genes were normalized to the reference genes Clathrin (At5g46630) and APT1 (At1g27450), which were selected with GENORM (Vandesompele et al., 2002), according to the method described in (Taylor et al., 2019). The primer sequences for RT-qPCR are provided in the supporting Supplementary Table S1.
Statistical analyses
The Software used was Grapdprism 9. The multiple comparison test applied is a non-parametric Krustall Wallis or Mann-Whitney test for single comparison.
Data availability statement
The original contributions presented in the study are included in the article/Supplementary Material. Further inquiries can be directed to the corresponding author.
Author contributions
CG: Writing – original draft, Writing – review & editing. AV: Writing – review & editing. SC: Writing – review & editing. FJ: Writing – review & editing. LG: Writing – review & editing. JP: Writing – review & editing. GM: Writing – review & editing. SB: Writing – review & editing, Writing – original draft.
Funding
The author(s) declare financial support was received for the research, authorship, and/or publication of this article. The IJPB benefits from the support of Saclay Plant Sciences-SPS(ANR-17-EUR-0007). CG’s PhD grant was funded by the Conseil Regional Hauts-de-France and BAP INRAE.
Conflict of interest
The authors declare that the research was conducted in the absence of any commercial or financial relationships that could be construed as a potential conflict of interest.
Publisher’s note
All claims expressed in this article are solely those of the authors and do not necessarily represent those of their affiliated organizations, or those of the publisher, the editors and the reviewers. Any product that may be evaluated in this article, or claim that may be made by its manufacturer, is not guaranteed or endorsed by the publisher.
Supplementary material
The Supplementary Material for this article can be found online at: https://www.frontiersin.org/articles/10.3389/fphgy.2024.1441158/full#supplementary-material
References
Atakhani A., Bogdziewiez Léa, Verger Stéphane (2022). Characterising the mechanics of cell–cell adhesion in plants. Quantitative Plant Biol. 3, e2. doi: 10.1017/qpb.2021.16
Barnes W. J., Zelinsky E., Anderson C. T. (2022). Polygalacturonase activity promotes aberrant cell separation in the quasimodo2 mutant of arabidopsis thaliana. Cell Surface 8, 100069. doi: 10.1016/j.tcsw.2021.100069
Bouton S., Leboeuf E., Mouille G., Leydecker M. Thérèse, Talbotec Joël, Granier F., et al. (2002). QUASIMODO1 encodes a putative membrane-bound glycosyltransferase required for normal pectin synthesis and cell adhesion in arabidopsis. Plant Cell 14, 2577–2905. doi: 10.1105/tpc.004259
Branca C., De Lorenzo G., Cervone F. (1988). Competitive inhibition of the auxin-induced elongation by α-D-oligogalacturonides in pea stem segments. Physiologia Plantarum 72, 499–504. doi: 10.1111/j.1399-3054.1988.tb09157.x
Daher F. B., Braybrook S. A. (2015). How to let go: pectin and plant cell adhesion. Front. Plant Sci. 6. doi: 10.3389/fpls.2015.00523
Denoux C., Galletti R., Mammarella N., Gopalan S., Werck Danièle, Lorenzo G. De, et al. (2008). Activation of defense response pathways by OGs and flg22 elicitors in arabidopsis seedlings. Mol. Plant 1, 423–455. doi: 10.1093/mp/ssn019
Du J., Kirui A., Huang S., Wang L., Barnes W. J., Kiemle S. N., et al. (2020a). Mutations in the pectin methyltransferase QUASIMODO2 influence cellulose biosynthesis and wall integrity in arabidopsis. Plant Cell 32, 3576–3597. doi: 10.1105/tpc.20.00252
Du J., Kirui A., Huang S., Wang L., Barnes W. J., Kiemle S. N., et al. (2020b). Mutations in the pectin methyltransferase QUASIMODO2 influence cellulose biosynthesis and wall integrity in arabidopsis [OPEN]. Plant Cell 32, 3576–3597. doi: 10.1105/tpc.20.00252
Ferrari S., Savatin D. V., Sicilia F., Gramegna G., Cervone F., Lorenzo. G. De (2013). Oligogalacturonides: plant damage-associated molecular patterns and regulators of growth and development. Front. Plant Sci. 4. doi: 10.3389/fpls.2013.00049
Gutierrez L., Bussell J. D., Pǎcurar D. I., Schwambach Josèli, Pǎcurar M., Bellini C. (2009). Phenotypic plasticity of adventitious rooting in arabidopsis is controlled by complex regulation of AUXIN RESPONSE FACTOR transcripts and microRNA abundance. Plant Cell 21, 3119–3325. doi: 10.1105/tpc.108.064758
Hijazi M., Durand J., Pichereaux C., Pont Frédéric, Jamet E., Albenne. Cécile (2012). Characterization of the arabinogalactan protein 31(AGP31) of arabidopsis thaliana: new advances on the hyp-O-Glycosylation of the pro-Rich domain. J. Biol. Chem. 287, 9623–9325. doi: 10.1074/jbc.M111.247874
Hijazi M., Roujol D., Nguyen-Kim H., Castillo L. D. R. C., Saland E., Jamet E., et al. (2014). Arabinogalactan protein 31(AGP31), a putative network-Forming protein in arabidopsis thaliana cell walls? Ann. Bot. 114, 1087–1975. doi: 10.1093/aob/mcu038
Hongo S., Sato K., yokoyama R., nishitani k. (2012). Demethylesterification of the primary wall by PECTIN METHYLESTERASE35 provides mechanical support to the arabidopsis stem. Plant Cell 24, 2624–2345. doi: 10.1105/tpc.112.099325
Kelly-Bellow R., Lee K., Kennaway R., Barclay J.E., Whibley A., Bushell C., et al. (2023). Brassinosteroid coordinates cell layer interactions in plants via cell wall and tissue. Science 380, 1275–1281. doi: 10.1126/science.adf0752
Kohorn B. D., Greed B. E., Mouille G., Verger Stéphane, Kohorn. S. L. (2021). Effects of arabidopsis wall associated kinase mutations on ESMERALDA1 and elicitor induced ROS. PloS One 16, 1–20. doi: 10.1371/journal.pone.0251922
Kohorn B. D., Kohorn S. L. (2012). The cell wall-associated kinases, WAKs, as pectin receptors. Front. Plant Sci. 3. doi: 10.3389/fpls.2012.00088
Lin W., Tang W., Pan X., Huang A., Gao X., Anderson C. T., et al. (2022)Arabidopsis pavement cell morphogenesis requires FERONIA binding to pectin for activation of ROP GTPase signalingCurr. Biol. 32, 497–5075.e4doi: 10.1016/j.cub.2021.11.030
Lionetti V., Cervone F., bellincampi d. (2012). Methyl esterification of pectin plays a role during plant–pathogen interactions and affects plant resistance to diseases. J. Plant Physiol. 169, 1623–1305. doi: 10.1016/j.jplph.2012.05.006
Mouille Grégory, Ralet M. C., Cavelier Céline, Eland C., Effroy D., Hématy K., et al. (2007). Homogalacturonan synthesis in arabidopsis thaliana requires a golgi-localized protein with a putative methyltransferase domain. Plant J. 50, 605–614. doi: 10.1111/j.1365-313X.2007.03086.x
Neumetzler L., Humphrey T., Lumba S., Snyder S., Yeats T. H., Usadel Björn, et al. (2012). The FRIABLE1 gene product affects cell adhesion in arabidopsis. PloS One 7 (8), e42914. doi: 10.1371/journal.pone.0042914
Nunes Cláudia, Silva L., Fernandes A. P., Guiné R. P. F., Domingues M.RosárioM., Coimbra. M. A. (2012). Occurrence of cellobiose residues directly linked to galacturonic acid in pectic polysaccharides. Carbohydr. Polymers 87, 620–265. doi: 10.1016/j.carbpol.2011.08.027
Ordaz-Ortiz JoséJ., Marcus S. E., Knox J.P. (2009). Cell wall microstructure analysis implicates hemicellulose polysaccharides in cell adhesion in tomato fruit pericarp parenchyma. Mol. Plant 2, 910–215. doi: 10.1093/mp/ssp049
Pelletier S., Orden JürgenV., Wolf S., Vissenberg K., Delacourt J., Ndong Y. A., et al. (2010). A role for pectin de-methylesterification in a developmentally regulated growth acceleration in dark-grown arabidopsis hypocotyls. New Phytol. 188, 726–739. doi: 10.1111/j.1469-8137.2010.03409.x
Pelloux Jérôme, Rustérucci C., Mellerowicz E. J. (2007). New insights into pectin methylesterase structure and function. Trends Plant Sci. 12, 267–775. doi: 10.1016/j.tplants.2007.04.001
Philippe F., Pelloux Jérôme, Rayon C. (2017). Plant pectin acetylesterase structure and function: new insights from bioinformatic analysis. BMC Genomics 18, 1–185. doi: 10.1186/s12864-017-3833-0
Qu T., Liu R., Wang W., An L., Chen T., Liu G., et al. (2011). Brassinosteroids regulate pectin methylesterase activity and atPME41 expression in arabidopsis under chilling stress. Cryobiology 63, 111–175. doi: 10.1016/j.cryobiol.2011.07.003
Sala K., Karcz J., Rypień A., Kurczyńska E. U. (2019). Unmethyl-Esterified homogalacturonan and extensins seal arabidopsis graft union. BMC Plant Biol. 19, 1–165. doi: 10.1186/s12870-019-1748-4
Sinclair S. A., Larue C., Bonk L., Khan A., Castillo-Michel H., Stein R. J., et al. (2017). Etiolated seedling development requires repression of photomorphogenesis by a small cell-wall-derived dark signal. Curr. Biol. 27, 3403–3418.e7. doi: 10.1016/j.cub.2017.09.063
Somerville C., Bauer S., Brininstool G., Facette M., Hamann T., Milne J., et al. (2004). Toward a systems approach toUnderstanding plant cell walls. Science 306, 2206–2211. doi: 10.1126/science.1102765
Taylor S. C., Nadeau K., Abbasi M., Lachance C., Nguyen M., Fenrich J. (2019). The ultimate QPCR experiment: producing publication quality, reproducible data the first time. Trends Biotechnol. 37, 761–745. doi: 10.1016/j.tibtech.2018.12.002
Valentin R., Cerclier C., Geneix N., Aguiée-Béeghin V., Gaillard C., Ralet M.-C., et al. (2010). Elaboration of extensin-pectin thin film model ofPrimary plant cell wall. Langmuir. 26, 9891–9898. doi: 10.1021/la100265d
Vandesompele Jo, Preter K. De, Pattyn F., Poppe B., Roy N. V., Paepe A. De, et al. (2002). Accurate normalization of real-time quantitative RT-PCR data by geometric averaging of multiple internal control genes. Genome Biol. 3, research0034.1. doi: 10.1186/gb-2002-3-7-research0034
Verger Stéphane, Chabout S., Gineau E., Mouille. Grégory (2016). Cell adhesion in plants is under the control of putative O-fucosyltransferases. Development 143, 2536–2540. doi: 10.1242/dev.132308
Voxeur A., Habrylo O., Guénin Stéphanie, Miart F., Soulié M. C., Rihouey C., et al. (2019). Oligogalacturonide production upon arabidopsis thaliana-botrytis cinerea interaction. Proc. Natl. Acad. Sci. United States America 116, 19743–19752. doi: 10.1073/pnas.1900317116
Wachananawat B., Kuroha T., Takenaka Y., Kajiura H., Naramoto S., Yokoyama R., et al. (2020). Diversity of pectin rhamnogalacturonan I rhamnosyltransferases in glycosyltransferase family 106. Front. Plant Sci. 11. doi: 10.3389/fpls.2020.00997
Wolf S. (2022). Cell wall signaling in plant development and defense. Annu. Rev. Plant Biol. 73, 323–353. doi: 10.1146/annurev-arplant-102820-095312
Xiao C., Zhang T., Zheng Y., Daniel J. (2016). Xyloglucan deficiency disrupts microtubule stability and cellulose biosynthesis in arabidopsis, altering cell growth and morphogenesis1[OPEN]. Plant Physiol. 170, 234–495. doi: 10.1104/pp.15.01395
Keywords: arabidopsis, cell wall, cell adhesion, pectin, oligogalacturonides (OGs)
Citation: Grandjean C, Voxeur A, Chabout S, Jobert F, Gutierrez L, Pelloux J, Mouille G and Bouton S (2024) Fine-tuning and remodeling of pectins play a key role in the maintenance of cell adhesion. Front. Plant Physiol. 2:1441158. doi: 10.3389/fphgy.2024.1441158
Received: 30 May 2024; Accepted: 26 July 2024;
Published: 15 August 2024.
Edited by:
Aleš Soukup, Charles University, CzechiaReviewed by:
Kenji Yamada, Jagiellonian University, PolandKatarzyna Sokołowska, University of Wrocław, Poland
Copyright © 2024 Grandjean, Voxeur, Chabout, Jobert, Gutierrez, Pelloux, Mouille and Bouton. This is an open-access article distributed under the terms of the Creative Commons Attribution License (CC BY). The use, distribution or reproduction in other forums is permitted, provided the original author(s) and the copyright owner(s) are credited and that the original publication in this journal is cited, in accordance with accepted academic practice. No use, distribution or reproduction is permitted which does not comply with these terms.
*Correspondence: Sophie Bouton, c29waGllLmJvdXRvbkB1LXBpY2FyZGllLmZy