- 1Department of Forest and Soil Sciences, Institute of Forest Ecology, University of Natural Resources and Life Sciences (BOKU), Vienna, Austria
- 2Department of Agroecology, Aarhus University, Slagelse, Denmark
- 3Department of Functional and Evolutionary Ecology, Faculty of Life Sciences, Molecular Systems Biology (MoSys), University of Vienna, Wien, Austria
- 4Vienna Metabolomics Center (VIME), University of Vienna, Wien, Austria
- 5Laboratory of Hormonal Regulations in Plants, Institute of Experimental Botany, Czech Academy of Sciences, Prague, Czechia
The need for increasing for crop productivity leads to a higher usage of synthetic fertilizers, which has tremendous effects on the environment. Nitrogen (N) is a crucial plant macronutrient, but the production of synthetic N fertilizer and its leakage into aquatic systems represent sources of environmental damage. To reduce the usage of synthetic fertilizers, current studies addressed innovative approaches to develop “N-self-fertilizing” crops that can utilize atmospheric nitrogen through enhanced interaction with the root microbiome. In this review we discuss recently obtained knowledge about the role of root hairs and their functions in root exudate secretion for plant-microbiome interactions. Recent studies have shown the beneficial impact of root hairs and exudate secretion on the recruitment of N2 fixing bacteria. Root hair plays a crucial role in shaping the rhizosphere, and first insights into the biological processes that underpin root hair formation and function in relation to microbiome interaction were gained. We summarize to which extent this knowledge can be applied to develop cereals with an enhanced ability to benefit from N2 fixing bacteria. Finally, we describe non-destructive methods and their limitations to study root hair growth directly in the field under natural growth conditions.
1 Introduction
The increasing population and the loss of fertile soil requires holistic approaches to enhance sustainable agriculture (Retzer and Weckwerth, 2023). Studies have shown that cereal crops deficient in root hair outgrowth exhibit reduced fitness (Giehl and von Wirén, 2014; Lopez et al., 2023; Xu et al., 2023). Root hair formation is a highly conserved process among plant species (Cui et al., 2018). The fate of root epidermal cells, which differentiate into either root hair or non-hair cells, is determined by a complex interplay of intrinsic and extrinsic cues that results in a predictable but highly plastic pattern of epidermal cells that can vary in shape, size, and function (Datta et al., 2011; Grierson et al., 2014; Mangano et al., 2017; Retzer and Weckwerth, 2021). Environmental information, which also includes the nutrient status of the soil, can be integrated at multiple points in the root hair morphogenetic pathway and affects multifaceted processes at the chromatin, transcriptional, and post-transcriptional levels (Datta et al., 2011; Giehl and von Wirén, 2014; Wu et al., 2019; Retzer and Weckwerth, 2021; Jia et al., 2023; Xu et al., 2023). Root hair outgrowth enlarges the root surface area in an energy and resource efficient way, facilitating efficient nutrient and water uptake, while root exudate secretion via root hairs may regulate the associate microbiome and enhance the prevalence of beneficial microorganisms that protect plants from pathogens and that provide additional nutrients (Pang et al., 2017; Pečenková et al., 2017; Holz et al., 2018; Kohli et al., 2022). The rhizosphere, which is the region of soil affected by the roots, is home to a diverse microbial community that can influence plant growth, fitness, and productivity (Canarini et al., 2019; Cadot et al., 2021). In addition, the presence of root hairs can affect the composition and function of the rhizosphere microbiome by providing a physical substrate for microbial attachment and colonization (Canarini et al., 2019; Kohli et al., 2022). The role of root hairs in exudate secretion and its importance particularly in attracting nitrogen-fixing bacteria, and the putative role of symbiotic cooperation for plant N-self-fertilization is intensively discussed (Rodriguez et al., 2019; Trivedi et al., 2020; Zhang et al., 2023). Recent research has shown that symbiotic bacteria may be key to producing better crops and reduce the application of synthetic fertilizers (Saleem et al., 2018).
Efficient nitrogen (N) acquisition is vital for plant fitness, as it is one of the most important macronutrients alongside phosphorus (Weckwerth et al., 2020; Burgess et al., 2023; Sepp et al., 2023). Synthetic N fertilizers have played a significant role in modern agriculture, however, they have negative effects on the environment due to their impact on greenhouse gas emissions and pollution (Rosenblueth et al., 2018; Burgess et al., 2023; Ghatak et al., 2023). Overuse of synthetic fertilizers has resulted in a significant environmental crisis, including reduced biodiversity in aquatic environments, among others caused by increased algae growth (Chen et al., 2020). The production of synthetic nitrogen fertilizer is an energy-intensive process that requires the use large amounts of energy, resulting in significant carbon dioxide emissions into the atmosphere (Chai et al., 2019) along with nitrogen gas emissions, which can exacerbate climate change even more compared to carbon dioxide (Chai et al., 2019; Pan et al., 2022).
In this review we summarize the recent progress in understanding how root hairs contribute to microbiome assembly and the potential of cereals to interact with N2 fixing bacteria. Improved cereal and root-microbiome interactions have the potential to substantially enhance biological N fixation (BNF), which would reduce pollution (Guo et al., 2023).
2 The potential of cereals to benefit from N2 fixing bacteria
Diazotrophic bacteria are capable of fixing atmospheric nitrogen into e.g. ammonia and providing it to plants in exchange for carbon resources (Rosenblueth et al., 2018; Ghatak et al., 2023; Guo et al., 2023; Zhang et al., 2023). Especially, the symbiosis between legumes and nitrogen-fixing bacteria is well studied, because it contributes to efficient N-self-fertilization, which can also be beneficial for other crops grown at the same field (Rosenblueth et al., 2018; Guo et al., 2023). Also cereals possess the potential to interact with N2 fixing bacteria, although these interactions do not reach the N fixing potential of legumes (Rosenblueth et al., 2018). Monocots were shown to interact with both associative and endophytic diazotrophs (Carvalho et al., 2014). Associative diazotrophic bacteria colonize the rhizosphere or root surface without forming an endosymbiotic relationship with them, whereas endophytic diazotrophic bacteria reside within plant tissues without causing any apparent harm to their hosts (Carvalho et al., 2014). The term self-fertilizing crops is recently frequently used to describe crops that form relationships with nitrogen-fixing microbes and the goal of recent research is to develop cereals that draw gaseous nitrogen directly from the soil, reducing the need for fertilizer and lowering farmer costs while mitigating environmental impacts.
In the past decade, several studies have shown that engineering plants to more efficiently harness microbiome interactions to enhance nitrogen availability and N use efficiency (NUE) is a promising approach to improve cereal fitness (Haskett et al., 2022; Yang et al., 2023). Modifications of cereals were divided into three categories, from first to third generation of N-self-fertilizing cereals, depending on the biological process targeted to improve N allocation (Guo et al., 2023). In the first generation, directly interaction with N2 fixing microbial communities is enhanced, whereby especially barley showed a high potential to attract N2 fixing bacteria with subsequent plant uptake of the fixed ammonia from the surrounding soil (Kozhemyakov, 1989; Haskett et al., 2022; Escudero-Martinez and Bulgarelli, 2023). The second-generation targets to transfer N fixation into the root, similar to the nodulation process of legumes, whereas third generation includes autonomous N fixation in plant organelles (Chakraborty et al., 2023; Guo et al., 2023). Other approaches for NUE enhancement in cereals are related to biological nitrification inhibition by root exudates and were discussed recently for more information see Ghatak et al. (2023).
All approaches to enhance NUE are still under investigation but have the potential to reduce the problems caused by the overuse of synthetic nitrogen fertilizer. Different strategies can be followed here. Genome editing of involved processes and screening of natural genetic variation for marker-assisted breeding (Weckwerth et al., 2020). The laws governing the usage and commercialization of genetically modified crops (GMO) for agricultural purposes vary across the globe (Turnbull et al., 2021). Some countries have banned GMOs altogether, while others have strict regulations in place (Weckwerth et al., 2020; Turnbull et al., 2021). In countries that banned in-field studies of GMOs the screening of large cereal germplasm to breed cereal cultivars with genetically enhanced NUE is still one of the most promising strategies (Weckwerth et al., 2020; Ghatak et al., 2023). In countries that don’t banned GMOs on the other hand it is highly promising to genetically engineer cereals to secrete enhanced amounts of certain root exudates that are tailored for specific diazotrophs (Chakraborty et al., 2023). Rhizopines are compounds that serve as C and N source for rhizobia, and recently engineered barley plants secreting rhizopines managed to attract diazotrophs (Haskett et al., 2022). Also here, the systematic analysis of large germplasm collections and natural variation of their root exudate metabolomes is a highly promising approach (Ghatak et al., 2023).
Selection of more efficient varieties or engineered plants could facilitate field symbiosis with N-fixing microorganisms. Moreover, the enrichment of N2 fixing microorganisms could also attract other beneficial bacteria. Plant interactions with non-diazotrophic bacteria can steer root growth plasticity. As an example, auxin-producing bacteria in barley rhizosheath have been shown to promote root hair outgrowth, which enhances barley yield by increasing spike number during drought (Xu et al., 2023). Furthermore, root hair function was already linked to efficient microbiome attraction in barley, as root hairless barley mutants showed reduced secretion and rhizosheath accumulation (Robertson-Albertyn et al., 2017; Galloway et al., 2022).
3 Root exudates govern root microbiome assembly
Plants can allocate as much as 50% of their carbon-based metabolites belowground in root biomass and root exudates (Kuzyakov and Domanski, 2000). Root exudates play an important role in the recruitment of mycorrhizal fungi and plant growth-promoting rhizobacteria (PGPR) (Vives-Peris et al., 2020). Exudates are composed of varying mixtures of primary and secondary metabolites, depending on available resources and the sum of environmental stimuli (Ghatak et al., 2023). Carbon and nitrogen are key elements that build up and keep a plant body efficiently growing, and at the end determine biomass and yield production (Ghatak et al., 2023). C is fixed by plants in the leaves through photosynthesis as carbohydrates, which are transported through the plant body and are further metabolized to contribute as signaling molecules, energy source or cellular building blocks (Wu et al., 2019; Retzer and Weckwerth, 2021; Galloway et al., 2022). Root exudates include carbohydrates, amino acids, flavonoids and other primary and secondary metabolites, that supply microorganisms with carbon, and beneficial microbes secrete ammonia and other compounds that are on the other hand beneficial for the host (Vives-Peris et al., 2020; Ghatak et al., 2023). So far, it is not fully understood if composition and secretion rate is actively modulated depending on external stimuli of the root. However, there is ample of evidence that e.g. drought stress changes subsequently root exudation and its impact on the soil microbiome (Ghatak et al., 2023). To heterotrophic microorganisms, the primary metabolites exuded by roots are a source of easily available carbon for growth and energy. The rhizosphere is therefore a zone of high microbial activity, and the enhanced availability of easily accessible organic substrates typically favors the growth of selected microbial taxa characterized by high growth rates under conditions of ample nutrient supply (Ghatak et al., 2023). In addition, the composition of root microbiomes is governed by root secondary metabolites. Comparisons of microbiomes of wildtype Arabidopsis thaliana and accessions carrying mutations in secondary metabolic pathways revealed that root production of various glucosinolates, flavonoids, camalexins, coumarins and defense hormones modulate the composition and diversity of bacterial and fungal root communities (Stringlis et al., 2018; Kudjordjie et al., 2021; Sikder et al., 2022). Similarly, the production of benzoxazinoids in Zea mays plays a significant role for the selection of root microbiomes (Kudjordjie et al., 2019; Cadot et al., 2021).
Interestingly, flavonoids in root exudates of non-leguminous plants appear to play a role in the enrichment of diazotrophs in the root microbiome. Exogenous addition of the flavonoids naringenin and daidzein enhanced root colonization of the N2 fixing bacteria Azorhizobium caulinodans and Herbaspirillum seropedicae in A. thaliana and wheat (Gough et al., 1997; Webster et al., 1998). Likewise, in wheat, exogenous addition of naringenin, daidzein, genisten, myricetin and apigenin promoted N2 fixing A. brasiliense (Webster et al., 1998). Whereas the above studies demonstrated that experimental supplementation of flavonoid compounds stimulate diazotroph colonization, a recent study demonstrated that excess flavonoid production by A. thaliana plants resulted in an enrichment of N2 fixing Azospirillum in the root microbiome (Kudjordjie et al., 2021). Further, root microbiomes of rice mutants with excess apigenin contents in roots and root exudates were enriched with diazotrophic bacteria, probably coupled to apigenin-enhanced diazoptrophic biofilm formation and root colonization (Yan et al., 2022). Hence, these studies suggest that flavonoids may not only regulate the establishment of N2 fixing Rhizobia symbionts in legumes, but play a similar role for the establishment of non-symbiotic diazotrophs in the root microbiome of non-legumes. The identification of several naringenin-regulated genes in the diazotroph H. seropedicae which are predicted to be involved in root colonization (Tadra-Sfeir et al., 2011) further lends support to the significance of flavonoids in non-leguminous root recruitment of N2 fixing bacteria.
Root exudates also modify soil properties, which includes alteration of soil pH to solubilize nutrients into assimilable forms. Modulation of pH also defines which microbiomes accumulate in the rhizosphere (Xu et al., 2023). The open research questions are therefore a, how exudates are released into the soil and b, how the release is regulated at cellular and molecular level, and c, how are exudates regulating microbes and stress resilience. Although crucial for plant productivity and studied in different model and crop plants, details about molecular mechanisms that underpin root exudate secretion are still elusive.
4 Root hairs are important for plant-microbe interactions
Plants evolved a fine-tuned network of intracellular processes to adapt to environmental changes leading to fast adaptation responses. Among other root growth responses, root hair outgrowth and exudate release belong to the so-called fast responses (Retzer and Weckwerth, 2021; Retzer and Weckwerth, 2023). Root hairs are extensions of the epidermal cells of roots that increase the surface area of the root and facilitate nutrient and water uptake from the soil (Grierson et al., 2014; Wu et al., 2019; Retzer and Weckwerth, 2021). Root hair outgrowth is a spatially and temporally highly regulated process and continuously further regulatory molecular players are identified and characterized (Rounds and Bezanilla, 2013; Grierson et al., 2014; Leyser, 2018; Zhu et al., 2020; Eljebbawi et al., 2021; García-González et al., 2021; Kuběnová et al., 2022; Starodubtseva et al., 2022). Xu et al. (2023) identified beneficial microbes that secrete indole-3-acetic acid (IAA), also known as auxin, a phytohormone that regulates root hair outgrowth in plants (Xu et al., 2023). This correlates with studies that show that root hair less barley plants interact less with microbes (Robertson-Albertyn et al., 2017; Xu et al., 2023).
Plant produced auxin is actively transported to different areas of the root to orchestrate root hair outgrowth (Jones et al., 2009; Grierson et al., 2014). In Arabidopsis thaliana auxin signaling pathways are activated to induce root hair formation to enhance nitrogen uptake under low nitrogen growth conditions (Jia et al., 2023). Evolutionary conserved auxin transporters, including AUXIN RESISTANT1 (AUX1) and PIN-FORMED2 (PIN2), canalize auxin from the root apex towards the root elongation zone to orchestrate root hair spacing, abundance, elongation rate and function (Rigas et al., 2013; Grierson et al., 2014; Dindas et al., 2018; Retzer et al., 2018; Lacek et al., 2021; Villaécija-Aguilar et al., 2022; Jia et al., 2023). Overall, exact spatial and temporal modulation of auxin synthesis, transport and signaling defines from early developmental stages every aspect of growth adaptation and cellular function (Pitts et al., 1998; Dolan, 2001; Grebe, 2004; Kiefer et al., 2015). Changing growth conditions require the adjustment of auxin homeostasis modulation to ensure efficient plant growth and fitness (Barrada et al., 2015; Zwiewka et al., 2015; Mroue et al., 2018; García-González et al., 2021). Plasticity of the root metabolome, and therefore the pool of available exudates, is also tightly coupled to auxin regulated signaling pathways (Hildreth et al., 2020). The photo assimilates and their metabolic products are transported through the plant body or directly produced in the root or even root hair and depending on their properties actively or passively secreted into the soil (Canarini et al., 2019; Retzer and Weckwerth, 2021; Ghatak et al., 2023).
Root hair outgrowth and function were extensively studied in the model plant A. thaliana to understand the molecular mechanisms underlying root hair development, that form a multi-layered gene regulatory network that enables the plant to respond flexibly and adequately to multiple signals (García-González et al., 2021; Retzer and Weckwerth, 2021). Cereals, including barley, share conserved molecular mechanisms with A. thaliana in regulating root hair growth (He et al., 2015; Alexander et al., 2019). Rice auxin-resistant mutants show the same root growth defects known from A. thaliana, including strongly reduced potential of auxin regulated root hair outgrowth (Meng et al., 2009; Meng et al., 2019). The continuously growing genetic and molecular tool box of mutants and reporter lines to study auxin homeostasis in barley shows that molecular processes that underpin auxin regulated root growth processes are conserved between cereals and A. thaliana (Kirschner et al., 2017; Kirschner et al., 2018). Also other molecular key players that are associated with efficient root hair formation and elongation were found to be crucial in barley for root hair development. Hence, homologues of β-expansin HvEXPB7 that steer efficient root hair elongation were also described in other plant species (He et al., 2015). HvEXB7 is furthermore a positive regulator of barley drought tolerance, which makes it further interesting to investigate the interplay of root hairs, exudate secretion and influence of PGPRs under extreme environmental conditions in cereals (He et al., 2015). The obtained knowledge can be transferred also to other crop species.
5 Ongoing development of research tools to dissect the role of root hair in field experiments
Phenotyping root hair growth plasticity is a crucial aspect of plant research (Müller and Schmidt, 2004; Wu et al., 2019; Retzer and Weckwerth, 2021). Recently, new phenotyping approaches have been developed to track root growth, including root hairs, in the field (York, 2019; Song et al., 2021). Root phenotyping techniques have seen significant improvements in terms of reduced destructiveness, image resolution and processing time (Atkinson et al., 2019; Song et al., 2021), and have enabled researchers to study root systems in greater detail, which is essential for crop selection and adaptation to emerging environmental challenges. Among advanced high-throughput root phenotyping approaches is the development of so-called minirhizotrons, devices that allow researchers to observe and study root systems in situ, allows tracking of dynamic root growth directly in the soil and are the least destructive method in the field (Arnaud et al., 2019; Rajurkar et al., 2022). Minirhizotrons are transparent tubes that are inserted into the soil, and root images are taken with cameras that are moved along the tube, and new imaging and detection systems are continuously developed to enhance resolution of the images (Figure 1) (Svane et al., 2019; Yu et al., 2020). In combination with high-resolution cameras, the application of minirhizotrons can support evaluation of root hair outgrowth deep in the soil over longer time periods. Together with further root sampling and phenotyping methods, such as shovelomics and soil coring, which are destructive because the roots are separated from the soil, the usage of minirhizotrons enables to depict dynamic root growth processes under natural conditions directly in the field (Arnaud et al., 2019; Freschet et al., 2021; York et al., 2022). Tracking root hair outgrowth directly in the soil will result in a better understanding of how plants interact with their environment and how they respond to individual stimuli.
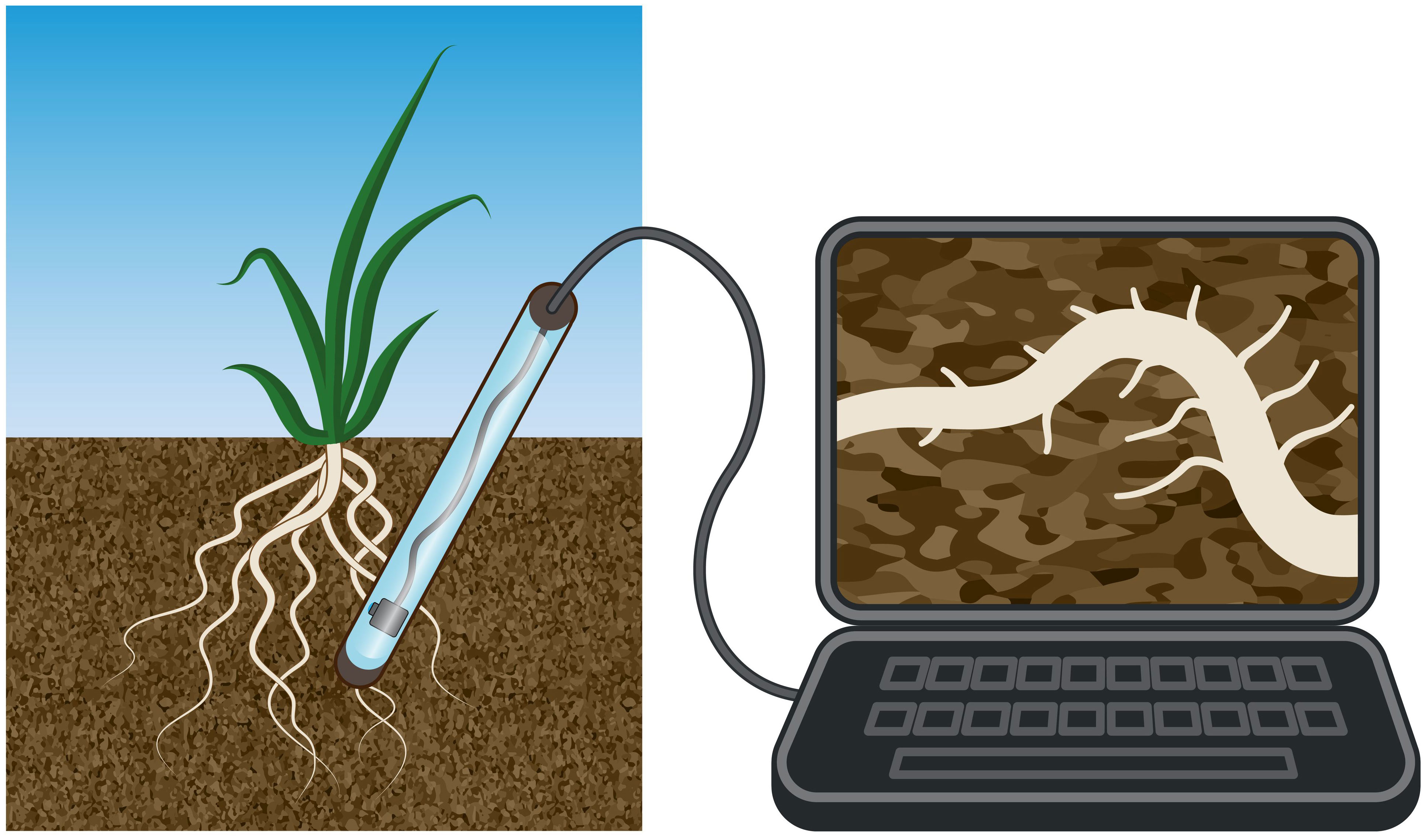
Figure 1 The minirhizotron system is a powerful tool for studying root growth and development in plants. Its non-destructive nature allows researchers to observe living roots in soil from season to season, while its high-resolution imaging capabilities enable tracking of root growth and hair outgrowth under natural growth conditions. The minirhizotron system has been used in many studies to investigate the effects of various environmental factors on root growth and development.
Functional root phenotyping can be linked with high-throughput OMICS analysis of samples taken from the plants and soil in the field (York, 2019; Ghatak et al., 2023; Retzer and Weckwerth, 2023). The high-throughput approaches result in a large amount of collected molecular and image data, which nowadays often becomes the bottleneck in phenomics (Song et al., 2021). New deep learning software tools are constantly being developed and possess the potential to increase data processing speed and accuracy (Danilevicz et al., 2021; Arya et al., 2022). Root hairs are often hard to distinguish from the soil, which can make it challenging to study them in image analysis tools. High-quality images of roots that show a good contrast between the root hairs and the soil are required for efficient image analysis, therefore usage of good cameras is beneficial for further processing, which includes enhancing of image quality by pre-processing, segmentation of the root and root hairs from the soil, feature extraction and finally the classification of obtained data (Taylor et al., 1990).
6 Conclusions
To cope with energy-demanding and harmful growth conditions, plants adjust their growth pattern and cell functions. Modulation of root growth allows the plant to grow towards resources, whereby especially root hair outgrowth enlarges the surface area to enhance uptake of nutrients and water. Furthermore, root hairs play a crucial role for plant-microbiome interaction, among others by secreting root exudates to enhance the recruitment of beneficial microorganisms. Nitrogen-fixing bacteria are particularly important, because they secure crucial N input that is required for sustainable agriculture.
Further studies are required to better understand the biological processes that positively influence root-microbe interactions and their impact on plant growth. In particular, we need to gain a better understanding of the role of root hairs in the interaction of plants with N2 fixing microbes. It is also essential to improve undisruptive, high-throughput phenotyping of roots to study root hair growth directly in the field. Combining the data obtained from phenotyping under natural conditions with studies of the dynamic adaptation in exudate secretion will provide valuable insights into the complex mechanisms that underpin plant-microbiome interactions.
In addition, future applications of enhanced symbiosis of cereals with nitrogen-fixing bacteria will help mitigate climate change by reducing the need for synthetic fertilizers and promoting sustainable agricultural practices.
Author contributions
SP: Writing – original draft, Writing – review & editing. BM: Writing – review & editing. HS: Writing – review & editing. MN: Writing – review & editing. WW: Writing – original draft. MV: Writing – original draft, Writing – review & editing. KR: Conceptualization, Writing – original draft, Writing – review & editing.
Funding
The author(s) declare financial support was received for the research, authorship, and/or publication of this article. SP, BM, MN, MV and KR are financially supported by the BarleyMicroBreed project, that has received funding from the European Union´s Horizon Europe research and innovation program under Grant Agreement No. 101060057.
Conflict of interest
The authors declare that the research was conducted in the absence of any commercial or financial relationships that could be construed as a potential conflict of interest.
The author(s) declared that they were an editorial board member of Frontiers, at the time of submission. This had no impact on the peer review process and the final decision.
Publisher’s note
All claims expressed in this article are solely those of the authors and do not necessarily represent those of their affiliated organizations, or those of the publisher, the editors and the reviewers. Any product that may be evaluated in this article, or claim that may be made by its manufacturer, is not guaranteed or endorsed by the publisher.
Author disclaimer
Views and opinions expressed are however those of the author(s) only and do not necessarily reflect those of the European Union or the European Research Executive Agency (REA). Neither the European Union nor the granting authority can be held responsible for them.
References
Alexander R. D., Wendelboe-Nelson C., Morris P. C. (2019). The barley transcription factor HvMYB1 is a positive regulator of drought tolerance. Plant Physiol. Biochem. PPB. 142, 246–53. doi: 10.1016/j.plaphy.2019.07.014
Arnaud M., Baird A. J., Morris P. J., Harris A., Huck J. J. (2019). EnRoot: a narrow-diameter, inexpensive and partially 3D-printable minirhizotron for imaging fine root production. Plant Methods 15 (1), 101. doi: 10.1186/s13007-019-0489-6
Arya S., Sandhu K. S., Jagamohanasimha, kumar S. (2022). Deep learning: as the new frontier in high-throughput plant phenotyping. Euphytica Netherlands J. Plant Breed 218 (4), 47. doi: 10.1007/s10681-022-02992-3
Atkinson J. A., Pound M. P., Bennett M. J., Wells D. M. (2019). Uncovering the hidden half of plants using new advances in root phenotyping. Curr. Opin. Biotechnol. 55, 1–8. doi: 10.1016/j.copbio.2018.06.002
Barrada A., Montané M. H., Robaglia C., Menand B. (2015). Spatial regulation of root growth: Placing the plant TOR pathway in a developmental perspective. Int. J. Mol. Sci. 16 (8), 19671–19697. doi: 10.3390/ijms160819671
Burgess A. J., Masclaux-Daubresse C., Strittmatter G., Weber A. P. M., Taylor S. H., Harbinson J., et al. (2023). Improving crop yield potential: Underlying biological processes and future prospects. Food Energy Secur 12 (1), e435. doi: 10.1002/fes3.435
Cadot S., Guan H., Bigalke M., Walser J.-C., Jander G., Erb M., et al. (2021). Specific and conserved patterns of microbiota-structuring by maize benzoxazinoids in the field. Microbiome 9 (1), 103. doi: 10.1186/s40168-021-01049-2
Canarini A., Kaiser C., Merchant A., Richter A., Wanek W. (2019). Root exudation of primary metabolites: mechanisms and their roles in plant responses to environmental stimuli. Front. Plant Sci. 10. doi: 10.3389/fpls.2019.00157
Carvalho T. L. G., Balsemão-Pires E., Saraiva R. M., Ferreira P. C. G., Hemerly A. S. (2014). Nitrogen signalling in plant interactions with associative and endophytic diazotrophic bacteria. J. Exp. Bot. 65 (19), 5631–5642. doi: 10.1093/jxb/eru319
Chai R., Ye X., Ma C., Wang Q., Tu R., Zhang L., et al. (2019). Greenhouse gas emissions from synthetic nitrogen manufacture and fertilization for main upland crops in China. Carbon Balance Manag 14 (1), 20. doi: 10.1186/s13021-019-0133-9
Chakraborty S., Venkataraman M., Infante V., Pfleger B. F., Ané J.-M. (2023). Scripting a new dialogue between diazotrophs and crops. Trends Microbiol., S0966-842X(23)00239-1. doi: 10.1016/j.tim.2023.08.007
Chen X., Strokal M., Kroeze C., Supit I., Wang M., Ma L., et al. (2020). Modeling the contribution of crops to nitrogen pollution in the yangtze river. Environ. Sci. Technol. 54 (19), 11929–11939. doi: 10.1021/acs.est.0c01333
Cui S., Suzaki T., Tominaga-Wada R., Yoshida S. (2018). Regulation and functional diversification of root hairs. Semin. Cell Dev. Biol. 83, 115–122. doi: 10.1016/j.semcdb.2017.10.003
Danilevicz M. F., Bayer P. E., Nestor B. J., Bennamoun M., Edwards D. (2021). Resources for image-based high-throughput phenotyping in crops and data sharing challenges. Plant Physiol. 187 (2), 699–715. doi: 10.1093/plphys/kiab301
Datta S., Kim C. M., Pernas M., Pires N. D., Proust H., Tam T., et al. (2011). Root hairs: Development, growth and evolution at the plant-soil interface. Plant Soil. 346, 1–14. doi: 10.1007/s11104-011-0845-4
Dindas J., Scherzer S., Roelfsema M. R. G., Von Meyer K., Müller H. M., Al-Rasheid K. A. S., et al. (2018). AUX1-mediated root hair auxin influx governs SCFTIR1/AFB-type Ca2+ signaling. Nat. Commun. 9 (1), 1174. doi: 10.1038/s41467-018-03582-5
Dolan L. (2001). How and where to build a root hair. Curr. Opin. Plant Biol. 4 (6), 550–554. doi: 10.1016/S1369-5266(00)00214-4
Eljebbawi A., Guerrero Y. D. C. R., Dunand C., Estevez J. M. (2021). Highlighting reactive oxygen species as multitaskers in root development. iScience 24 (1), 101978. doi: 10.1016/j.isci.2020.101978
Escudero-Martinez C., Bulgarelli D. (2023). Engineering the crop microbiota through host genetics. Annu. Rev. Phytopathol. 61, 257–277. doi: 10.1146/annurev-phyto-021621-121447
Freschet G. T., Pagès L., Iversen C. M., Comas L. H., Rewald B., Roumet C., et al. (2021). A starting guide to root ecology: strengthening ecological concepts and standardising root classification, sampling, processing and trait measurements. New Phytol. 232 (3), 973–1122. doi: 10.1111/nph.17572
Galloway A. F., Akhtar J., Burak E., Marcus S. E., Field K. J., Dodd I. C., et al. (2022). Altered properties and structures of root exudate polysaccharides in a root hairless mutant of barley. Plant Physiol. 190 (2), 1214–1227. doi: 10.1093/plphys/kiac341
García-González J., Lacek J., Retzer K. (2021). Dissecting hierarchies between light, sugar and auxin action underpinning root and root hair growth. Plants (Basel, Switzerland). 10 (1), 111. https://doi.org/10.3390/plants10010111
Ghatak A., Chaturvedi P., Waldherr S., Subbarao G. V., Weckwerth W. (2023). PANOMICS at the interface of root-soil microbiome and BNI. Trends Plant Sci. 28 (1), 106–122. doi: 10.1016/j.tplants.2022.08.016
Giehl R. F. H., von Wirén N. (2014). Root nutrient foraging. Plant Physiol. [Internet]. 166 (2), 509–517. doi: 10.1104/pp.114.245225
Gough C., Galera C., Vasse J., Webster G., Cocking E. C., Dénarié J. (1997). Specific flavonoids promote intercellular root colonization of Arabidopsis thaliana by Azorhizobium caulinodans ORS571. Mol. Plant Microbe Interact. 10 (5), 560–570. doi: 10.1094/MPMI.1997.10.5.560
Grebe M. (2004). Ups of and downs of tissue and planar polarity in plants. BioEssays. 26 (7), 719–729. doi: 10.1002/bies.20065
Grierson C., Nielsen E., Ketelaarc T., Schiefelbein J. (2014). Root hairs. Arab B 12, e0172–e0172. doi: 10.1199/tab.0172
Guo K., Yang J., Yu N., Luo L., Wang E. (2023). Biological nitrogen fixation in cereal crops: Progress, strategies, and perspectives. Plant Commun. 4 (2), 100499. doi: 10.1016/j.xplc.2022.100499
Haskett T. L., Paramasivan P., Mendes M. D., Green P., Geddes B. A., Knights H. E., et al. (2022). Engineered plant control of associative nitrogen fixation. Proc. Natl. Acad. Sci. U. S. A. 119 (16), e2117465119. doi: 10.1073/pnas.2117465119
He X., Zeng J., Cao F., Ahmed I. M., Zhang G., Vincze E., et al. (2015). HvEXPB7, a novel β-expansin gene revealed by the root hair transcriptome of Tibetan wild barley, improves root hair growth under drought stress. J. Exp. Bot. 66 (22), 7405–7419. doi: 10.1093/jxb/erv436
Hildreth S. B., Foley E. E., Muday G. K., Helm R. F., Winkel B. S. J. (2020). The dynamic response of the Arabidopsis root metabolome to auxin and ethylene is not predicted by changes in the transcriptome. Sci. Rep. 10 (1), 679. doi: 10.1038/s41598-019-57161-9
Holz M., Zarebanadkouki M., Kuzyakov Y., Pausch J., Carminati A. (2018). Root hairs increase rhizosphere extension and carbon input to soil. Ann. Bot. 121 (1), 61–69. doi: 10.1093/aob/mcx127
Jia Z., Giehl R. F. H., Hartmann A., Estevez J. M., Bennett M. J., von Wirén N. (2023). A spatially concerted epidermal auxin signaling framework steers the root hair foraging response under low nitrogen. Curr. Biol. 33 (18), 3926–3941.e5. doi: 10.1016/j.cub.2023.08.040
Jones A. R., Kramer E. M., Knox K., Swarup R., Bennett M. J., Lazarus C. M., et al. (2009). Auxin transport through non-hair cells sustains root-hair development. Nat. Cell Biol. 11 (1), 78–84. doi: 10.1038/ncb1815
Kiefer C. S., Claes A. R., Nzayisenga J. C., Pietra S., Stanislas T., Hüser A., et al. (2015). Arabidopsis AIP1-2 restricted by WER-mediated patterning modulates planar polarity. Dev (Cambridge, England). 142 (1), 151–161. doi: 10.1242/dev.111013
Kirschner G. K., Stahl Y., Imani J., von Korff M., Simon R. (2018). Fluorescent reporter lines for auxin and cytokinin signalling in barley (Hordeum vulgare). PloS One 13 (4), e0196086. doi: 10.1371/journal.pone.0196086
Kirschner G. K., Stahl Y., Von Korff M., Simon R. (2017). Unique and conserved features of the barley root meristem. Front. Plant Sci. 8, 1240. doi: 10.3389/fpls.2017.01240
Kohli P. S., Maurya K., Thakur J. K., Bhosale R., Giri J. (2022). Significance of root hairs in developing stress-resilient plants for sustainable crop production. Plant Cell Environ. 45 (3), 677–694. doi: 10.1111/pce.14237
Kozhemyakov A. P. (1989). “Quantitative Estimation of Nitrogen Fixation by Barley Associative Bacteria Using Tracer Technique,” in Interrelationships between Microorganisms and Plants in Soil. Eds. Vančura V., Kunc FBT-D (Elsevier), 273–276. Available at: https://www.sciencedirect.com/science/article/pii/S0166248108702241.
Kuběnová L., Tichá M., Šamaj J., Ovečka M. (2022). ROOT HAIR DEFECTIVE 2 vesicular delivery to the apical plasma membrane domain during Arabidopsis root hair development. Plant Physiol. 188 (3), 1563–1585. doi: 10.1093/plphys/kiab595
Kudjordjie E. N., Sapkota R., Nicolaisen M. (2021). Arabidopsis assemble distinct root-associated microbiomes through the synthesis of an array of defense metabolites. PloS One 16 (10), e0259171. doi: 10.1371/journal.pone.0259171
Kudjordjie E. N., Sapkota R., Steffensen S. K., Fomsgaard I. S., Nicolaisen M. (2019). Maize synthesized benzoxazinoids affect the host associated microbiome. Microbiome 7 (1), 59. doi: 10.1186/s40168-019-0677-7
Kuzyakov Y., Domanski G. (2000). Carbon input by plants into the soil. Review. J. Plant Nutr. Soil Sci. 163 (4), 421–431. doi: 10.1002/1522-2624(200008)163:4%3C421::AID-JPLN421%3E3.0.CO
Lacek J., García-González J., Weckwerth W., Retzer K. (2021). Lessons learned from the studies of roots shaded from direct root illumination. Int. J. Mol. Sci. 22 (23), 12784. doi: 10.3390/ijms222312784
Lopez G., Ahmadi S. H., Amelung W., Athmann M., Ewert F., Gaiser T., et al. (2023). Nutrient deficiency effects on root architecture and root-to-shoot ratio in arable crops. Front. Plant Sci. 13. doi: 10.3389/fpls.2022.1067498
Mangano S., Denita-Juarez S. P., Choi H.-S., Marzol E., Hwang Y., Ranocha P., et al. (2017). Molecular link between auxin and ROS-mediated polar growth. Proc. Natl. Acad. Sci. U S A. 114 (20), 5289–5294. doi: 10.1073/pnas.1701536114
Meng F., Xiang D., Zhu J., Li Y., Mao C. (2019). Molecular mechanisms of root development in rice. Rice (N Y). 12 (1), 1. doi: 10.1186/s12284-018-0262-x
Meng Y., Huang F., Shi Q., Cao J., Chen D., Zhang J., et al. (2009). Genome-wide survey of rice microRNAs and microRNA–target pairs in the root of a novel auxin-resistant mutant. Planta 230 (5), 883–898. doi: 10.1007/s00425-009-0994-3
Mroue S., Simeunovic A., Robert H. S. (2018). Auxin production as an integrator of environmental cues for developmental growth regulation. J. Exp. Bot. 69 (2), 201–212. doi: 10.1093/jxb/erx259
Müller M., Schmidt W. (2004). Environmentally induced plasticity of root hair development in arabidopsis. Plant Physiol. 134 (1), 409–419. doi: 10.1104/pp.103.029066
Pan S.-Y., He K.-H., Lin K.-T., Fan C., Chang C.-T. (2022). Addressing nitrogenous gases from croplands toward low-emission agriculture. NPJ Clim Atmos Sci. 5 (1), 43. doi: 10.1038/s41612-022-00265-3
Pang J., Ryan M. H., Siddique K. H. M., Simpson R. J. (2017). Unwrapping the rhizosheath. Plant Soil 418 (1), 129–139. doi: 10.1007/s11104-017-3358-y
Pečenková T., Janda M., Ortmannová J., Hajná V., Stehlíková Z., Žárský V. (2017). Early Arabidopsis root hair growth stimulation by pathogenic strains of Pseudomonas syringae. Ann. Bot. 120 (3), 437–446. doi: 10.1093/aob/mcx073
Pitts R. J., Cernac A., Estelle M. (1998). Auxin and ethylene promote root hair elongation in Arabidopsis. Plant J. 16 (5), 553–560. doi: 10.1046/j.1365-313x.1998.00321.x
Rajurkar A. B., McCoy S. M., Ruhter J., Mulcrone J., Freyfogle L., Leakey A. D. B. (2022). Installation and imaging of thousands of minirhizotrons to phenotype root systems of field-grown plants. Plant Methods 18 (1), 39. doi: 10.1186/s13007-022-00874-2
Retzer K., Singh G., Napier R. M. (2018). It starts with TIRs. Nat. Plants. 4 (7), 410–411. doi: 10.1038/s41477-018-0196-8
Retzer K., Weckwerth W. (2021). The tor–auxin connection upstream of root hair growth. Plants (Basel, Switzerland). 10 (1), 150. doi: 10.3390/plants10010150
Retzer K., Weckwerth W. (2023). Recent insights into metabolic and signalling events of directional root growth regulation and its implications for sustainable crop production systems. Front. Plant Sci. 14, 1154088. doi: 10.3389/fpls.2023.1154088
Rigas S., Ditengou F. A., Ljung K., Daras G., Tietz O., Palme K., et al. (2013). Root gravitropism and root hair development constitute coupled developmental responses regulated by auxin homeostasis in the Arabidopsis root apex. New Phytol. 197 (4), 1130–1141. doi: 10.1111/nph.12092
Robertson-Albertyn S., Alegria Terrazas R., Balbirnie K., Blank M., Janiak A., Szarejko I., et al. (2017). Root hair mutations displace the barley rhizosphere microbiota. Front. Plant Sci. 8, 1094. doi: 10.3389/fpls.2017.01094
Rodriguez P. A., Rothballer M., Chowdhury S. P., Nussbaumer T., Gutjahr C., Falter-Braun P. (2019). Systems biology of plant-microbiome interactions. Mol. Plant 12 (6), 804–821. doi: 10.1016/j.molp.2019.05.006
Rosenblueth M., Ormeño-Orrillo E., López-López A., Rogel M. A., Reyes-Hernández B. J., Martínez-Romero J. C., et al. (2018). Nitrogen fixation in cereals. Front. Microbiol. 9, 1794. doi: 10.3389/fmicb.2018.01794
Rounds C. M., Bezanilla M. (2013). Growth mechanisms in tip-growing plant cells. Annu. Rev. Plant Biol. 64, 243–265. doi: 10.1146/annurev-arplant-050312-120150
Saleem M., Law A. D., Sahib M. R., Pervaiz Z. H., Zhang Q. (2018). Impact of root system architecture on rhizosphere and root microbiome. Rhizosphere 6, 47–51. doi: 10.1016/j.rhisph.2018.02.003
Sepp S.-K., Vasar M., Davison J., Oja J., Anslan S., Al-Quraishy S., et al. (2023). Global diversity and distribution of nitrogen-fixing bacteria in the soil. Front. Plant Sci. 14. doi: 10.3389/fpls.2023.1100235
Sikder M. M., Vestergård M., Kyndt T., Topalović O., Kudjordjie E. N., Nicolaisen M. (2022). Genetic disruption of Arabidopsis secondary metabolite synthesis leads to microbiome-mediated modulation of nematode invasion. ISME J. 16 (9), 2230–2241. doi: 10.1038/s41396-022-01276-x
Song P., Wang J., Guo X., Yang W., Zhao C. (2021). High-throughput phenotyping: Breaking through the bottleneck in future crop breeding. Crop J. 9 (3), 633–645. doi: 10.1016/j.cj.2021.03.015
Starodubtseva A., Kalachova T., Retzer K., Jelínková A., Dobrev P., Lacek J., et al. (2022). An Arabidopsis mutant deficient in phosphatidylinositol-4-phosphate kinases ß1 and ß2 displays altered auxin-related responses in roots. Sci. Rep. 12 (1), 6947. doi: 10.1038/s41598-022-10458-8
Stringlis I. A., Yu K., Feussner K., de Jonge R., Van Bentum S., Van Verk M. C., et al. (2018). MYB72-dependent coumarin exudation shapes root microbiome assembly to promote plant health. Proc. Natl. Acad. Sci. U S A. 115 (22), E5213–E5222. doi: 10.1073/pnas.1722335115
Svane S. F., Dam E. B., Carstensen J. M., Thorup-Kristensen K. (2019). A multispectral camera system for automated minirhizotron image analysis. Plant Soil 441 (1), 657–672. doi: 10.1007/s11104-019-04132-8
Tadra-Sfeir M. Z., Souza E. M., Faoro H., Müller-Santos M., Baura V. A., Tuleski T. R., et al. (2011). Naringenin regulates expression of genes involved in cell wall synthesis in Herbaspirillum seropedicae. Appl. Environ. Microbiol. 77 (6), 2180–2183. doi: 10.1128/AEM.02071-10
Taylor H. M., Upchurch D. R., McMichael B. L. (1990). Applications and limitations of rhizotrons and minirhizotrons for root studies. Plant Soil 129 (1), 29–35. doi: 10.1007/BF00011688
Trivedi P., Leach J. E., Tringe S. G., Sa T., Singh B. K. (2020). Plant–microbiome interactions: from community assembly to plant health. Nat. Rev. Microbiol. 18 (11), 607–621. doi: 10.1038/s41579-020-0412-1
Turnbull C., Lillemo M., Hvoslef-Eide T. A. K. (2021). Global regulation of genetically modified crops amid the gene edited crop boom - A review. Front. Plant Sci. 12, 630396. doi: 10.3389/fpls.2021.630396
Villaécija-Aguilar J. A., Körösy C., Maisch L., Hamon-Josse M., Petrich A., Magosch S., et al. (2022). KAI2 promotes Arabidopsis root hair elongation at low external phosphate by controlling local accumulation of AUX1 and PIN2. Curr. Biol. 32 (1), 228–236.e3. doi: 10.1016/j.cub.2021.10.044
Vives-Peris V., de Ollas C., Gómez-Cadenas A., Pérez-Clemente R. M. (2020). Root exudates: from plant to rhizosphere and beyond. Plant Cell Rep. 39 (1), 3–17. doi: 10.1007/s00299-019-02447-5
Webster G., Jain V., Davey M. R., Gough C., Vasse J., Dénarié J., et al. (1998). The flavonoid naringenin stimulates the intercellular colonization of wheat roots by Azorhizobium caulinodans. Plant Cell \& Environ. 21 (4), 373–383. doi: 10.1046/j.1365-3040.1998.00278.x
Weckwerth W., Ghatak A., Bellaire A., Chaturvedi P., Varshney R. K. (2020). PANOMICS meets germplasm. Plant Biotechnol. J. 18 (7), 1507–1525. doi: 10.1111/pbi.13372
Wu Y., Shi L., Li L., Fu L., Liu Y., Xiong Y., et al. (2019). Integration of nutrient, energy, light, and hormone signalling via TOR in plants. J. Exp. Bot. 70 (8), 2227–2238. doi: 10.1093/jxb/erz028
Xu F., Liao H., Yang J., Zhang Y., Yu P., Cao Y., et al. (2023). Auxin-producing bacteria promote barley rhizosheath formation. Nat. Commun. [Internet]. 14 (1), 5800. doi: 10.1038/s41467-023-40916-4
Yan D., Tajima H., Cline L. C., Fong R. Y., Ottaviani J. I., Shapiro H.-Y., et al. (2022). Genetic modification of flavone biosynthesis in rice enhances biofilm formation of soil diazotrophic bacteria and biological nitrogen fixation. Plant Biotechnol. J. 20 (11), 2135–2148. doi: 10.1111/pbi.13894
Yang H., Berckx F., Fransson P., Weih M. (2023). Harnessing plant–microbe interactions to promote nitrogen use efficiency in cereal crops. Plant Soil. doi: 10.1007/s11104-023-06278-y
York L. M. (2019). Functional phenomics: an emerging field integrating high-throughput phenotyping, physiology, and bioinformatics. J. Exp. Bot. 70 (2), 379–386. doi: 10.1093/jxb/ery379
York L. M., Griffiths M., Maaz T. M. (2022). Whole-plant phenotypic engineering: moving beyond ratios for multi-objective optimization of nutrient use efficiency. Curr. Opin. Biotechnol. 75, 102682. doi: 10.1016/j.copbio.2022.102682
Yu G., Zare A., Sheng H., Matamala R., Reyes-Cabrera J., Fritschi F. B., et al. (2020). Root identification in minirhizotron imagery with multiple instance learning. Mach. Vis. Appl. 31 (6), 43. doi: 10.1007/s00138-020-01088-z
Zhang W., Chen Y., Huang K., Wang F., Mei Z. (2023). Molecular mechanism and agricultural application of the nifA-nifL system for nitrogen fixation. Int. J. Mol. Sci. 24 (2), 907. doi: 10.3390/ijms24020907
Zhu S., Martínez Pacheco J., Estevez J. M., Yu F. (2020). Autocrine regulation of root hair size by the RALF-FERONIA-RSL4 signaling pathway. New Phytol. 227 (1), 45–49. doi: 10.1111/nph.16497
Keywords: root hair, root hair phenotyping, in-field phenotyping, minirhizotron, root-microbiome interactions
Citation: Pree S, Malekian B, Sandén H, Nicolaisen M, Weckwerth W, Vestergård M and Retzer K (2023) Deciphering the biological processes in root hairs required for N-self-fertilizing cereals. Front. Plant Physiol. 1:1308534. doi: 10.3389/fphgy.2023.1308534
Received: 06 October 2023; Accepted: 08 December 2023;
Published: 21 December 2023.
Edited by:
Yasuyuki Kawaharada, Iwate University, JapanReviewed by:
Kenji Yamada, Jagiellonian University, PolandCopyright © 2023 Pree, Malekian, Sandén, Nicolaisen, Weckwerth, Vestergård and Retzer. This is an open-access article distributed under the terms of the Creative Commons Attribution License (CC BY). The use, distribution or reproduction in other forums is permitted, provided the original author(s) and the copyright owner(s) are credited and that the original publication in this journal is cited, in accordance with accepted academic practice. No use, distribution or reproduction is permitted which does not comply with these terms.
*Correspondence: Katarzyna Retzer, retzer@ueb.cas.cz