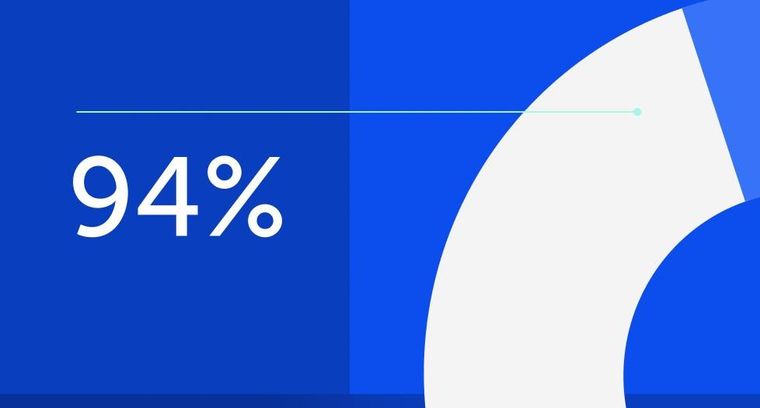
94% of researchers rate our articles as excellent or good
Learn more about the work of our research integrity team to safeguard the quality of each article we publish.
Find out more
MINI REVIEW article
Front. Plant Physiol., 13 November 2023
Sec. Molecular and Cellular Biology
Volume 1 - 2023 | https://doi.org/10.3389/fphgy.2023.1285373
Plants may lack mobility, but they are not defenseless against the constant threats posed by pathogens and pests. Pattern Recognition Receptors (PRRs), which are located on the plasma membrane, enable plants to effectively recognize intruders. These receptors function by sensing elicitors or fragments of the cell wall that arise from damage. Recent studies underscore the significance of maintaining cell wall integrity in the coordination of defense mechanisms following the detection of parasitism. Pathogen invasion often triggers alterations in cell wall structure, which leads to the release of molecules like β-glucans and oligogalacturonides. These small molecules are then recognized by PRRs, which stimulate downstream signaling pathways that involve both receptor-like kinases and calcium-dependent signaling. Here, we present the latest insights into plant signaling that play a vital role in immunity: the maintenance of cell wall integrity; the intricate interplay between receptor-like kinases; and the involvement of calcium ions. The goal of the review is to provide readers with a deeper understanding of the intricate mechanisms underlying plant defense strategies.
The sessile nature of the plant kingdom has driven the evolution of intricate signaling mechanisms that can effectively detect, as well as combat, the biotic stresses imposed by pathogens and pests (Glazebrook, 2005). The plant cell wall serves as the primary barrier against these stressors, and is crucial not only for protection, but also for sensing external attack (Cosgrove, 2005). In the quest to identify potential threats, plants have developed a sophisticated surveillance system that relies on the recognition of distinct molecules known as microbe-associated molecular patterns (MAMPs), pathogen-associated molecular patterns (PAMPs), damage-associated molecular patterns (DAMPs), and even herbivore-associated molecular patterns (HAMPs) (Chisholm et al., 2006). These molecular patterns are detected by specialized pattern recognition receptors (PRRs) (Jones and Dangl, 2006) as well as surface receptors, e.g., receptor-like kinases (RLKs) and wall-associated kinases (WAKs) (Decreux and Messiaen, 2005; Decreux et al., 2006). Recent advances have provided insight into how plants maintain cell wall integrity (Bacete and Hamann, 2020). In addition to orchestrating the immune response, the cell wall has the vital function of detecting both mechanical and pathogen-induced stress (Cosgrove, 2005). Specific RLKs play pivotal roles in detecting cell wall damages and can engage plant defense complexes to subsequently initiate pattern-triggered immunity (PTI). Alongside protons (H+), calcium (Ca2+) has recently emerged as a pivotal bivalent cation that is essential for plant nutrition, cellular structure, and stress responses (Thor, 2019). In plants, the maintenance of the cell wall integrity along with the perception of cell wall damages, are intricately linked to Ca2+ ions, which can participate in the cross-linking of negative charges at the cell wall interface; as such, the cell wall is effectively a Ca2+ reservoir in plants (Hepler and Winship, 2010). In plants, the primary role of Ca2+ is to transmit electrical signals to neighboring cells and other plant organs so that specific messages can be relayed once distinct cues are detected (Bush, 1995; Choi et al., 2017). Interestingly, recent research has revealed that calcium ions act as crucial secondary messengers during cell wall damages perception, cell wall integrity regulation, and the plant immune response.
In this review, we initially describe the realm of cell wall-related plant immunity with a specific focus on the role of RLKs in both plant defense mechanisms and cell wall integrity regulation. Next, we explore the emerging evidence that Ca2+ acts as a pivotal secondary messenger in both cell wall integrity regulation and plant immunity.
The plant cell wall (CW) serves as the primary barrier for deterring pathogens; as such, there has been strong evolutionary pressure for the development of cell wall perception, remodeling, and reinforcement mechanisms in plant defense strategies (Cosgrove, 2005; Glazebrook, 2005). The primary cell wall is predominantly composed of oligosaccharides (e.g., cellulose, hemicellulose, and pectin), while the secondary cell wall has a similar composition but lower lignin content (Bidlack, 1992; Cosgrove and Jarvis, 2012). Additionally, the plant cell wall contains a substantial proportion of proteins, which account for approximately one-tenth of the total biomass (Keller, 1993; Cassab, 1998). These proteins play a pivotal role in modifying the CW, facilitating perception, and enabling signal transduction. Recent empirical findings highlight how the maintenance of CW integrity is critical to detecting parasitism and monitoring plant defense responses (Vaahtera et al., 2019; Wan et al., 2021). Intrusion by pathogens or parasites is frequently correlated with changes in CW structure (Gigli-Bisceglia et al., 2020). For instance, pathogens secrete cell-wall degrading enzymes (CWDEs), such as cellulases, glucanases, and xylanases (van den Brink and de Vries, 2011), and the action of these enzymes releases various elicitors into the apoplast. These elicitors include β-1,2-glucans, degradation product of cellulose, β-1,3-glucans, degradation product of callose, and α-1,4-oligogalacturonides (OGs), which is the degradation product of pectins. As a result of cell wall degradation, Ca2+ cations, which were originally present in the pectin and hemicellulose components of the cell wall, are released into the apoplast (Jarvis, 1982; Engelsdorf et al., 2016). This triggers an influx of Ca2+ into the cytoplasm (Clapham, 2007). The degradation products of CWDE activity fall under a class of molecules known as DAMPs, which are recognized by pattern PRRs (Bacete and Hamann, 2020; Souza et al., 2017). Following the perception of cell wall damage, PRRs transmit downstream signals via receptor-like kinases (RLKs) or calcium (Ca2+)-dependent signaling to ultimately initiate MAPK signaling cascades, which stimulates the production of phytohormones such as jasmonate (JA), salicylic acid (SA), ethylene (ET), and abscisic acid (ABA) (Figure 1, Zhou and Zhang, 2020). The rapid influx of Ca2+ into the cytoplasm activates enzymes that are responsible for the biosynthesis of phytohormones, as well as stimulates the transcription factors that regulate genes linked with phytohormone production (Zhou and Zhang, 2020). Changes in apoplastic Ca2+ levels also mediate cell wall loosening and adjustments via the action of PECTIN METHYL-TRANSFERASES (PMEs), which require Ca2+ for activation and the cross-linking of pectin (Wu et al., 2018; Gale et al., 2019). Moreover, shifts in distinct phytohormone pools will activate the expression of specific genes, leading to modifications in MAPKs signaling and subsequent changes in cell wall structure and the defense response (Engelsdorf and Hamann, 2014; Miedes et al., 2014; Zipfel, 2014; Bacete et al., 2017; Wolf, 2017).
Figure 1 Plant cell wall damages perception. PRR involved in CWD, and MAMPS/PAMPS/DAMPs are specifically recognizing their substrate and transducing the signal to the cytoplasm. This signal consists in Ca2+ channels and RBOHD activation, leading to an oxidative burst and Ca2+ influx. RLCKs are also PRR activated and phosphorylated, leading to Ca2+ channel activation such as CNGCs. The different Ca2+ influx transduce a specific Ca2+ signature decoded via CPS CMLs, CBLs, CDPKs or CIPKS. The different CPS will then specifically TF to induce defence or cell wall maintenance related genes. PRR are also directly activating MAPKs cascade signalling leading also to TF activation and defence genes expression. The different genes activated will in turn activate specific phytohormones biosynthesis as well as cell modification enzyme or components (e.g., lignin).
The membrane-localized PRRs are a central part of how plants detect damage from external pathogens via the perception of PAMPs/DAMPs/MAMPs. Among these, the most extensively studied is the LRR-RKs receptor FLAGELLIN-SENSING2 (FLS2), which operates in conjunction with the co-receptor BRASSINOSTEROID INSENSITIVE 1-ASSOCIATED KINASE 1 (BAK1 or SERK3) from the SOMATIC EMBRYOGENESIS RECEPTOR KINASES (SERK) family (Table 1, Goméz-Goméz and Boller, 2000; Chinchilla et al., 2007; Heese et al., 2007). In terms of the mechanism, FLS2 binds the PAMP-peptide flg22, which promotes FLS2/BAK1/BIK1 complex formation and the subsequent phosphorylation of BOTRYTIS INDUCED KINASE 1 (BIK1) cytoplasmic domains by BRI1-ASSOCIATED RECEPTOR KINASE 1 (BAK1) (Lu et al., 2010). These phosphorylation events are sensed by RECEPTOR-LIKE CYTOPLASMIC KINASES (RLCKs) to initiate phosphorylation cascades that culminate in the activation of target proteins; this is an example of PAMP-triggered immunity (PTI) (Liang and Zhou, 2018; DeFalco and Zipfel, 2021). Another significant LRR-RK receptor, PEPR1, has recently emerged as a key player in the perception of CW damages (Yamaguchi et al., 2010). Like FLS2, PEPR1 is a ligand-specific receptor that recognizes the endogenous peptide signal DAMP-associated PEPTIDE 1 (AtPep1). This AtPep1 peptide forms following the C-terminal cleavage of AtProPep1, a product of the PROPEP1 gene. PROPEP1 expression is strongly induced during cell wall degradation, wound responses, and the recognition of elicitors such as jasmonic acid (JA) and ethylene (Et) (Huffaker et al., 2006). PEPR1 also operates by activating the immune response, which exemplifies the convergence of bacterial elicitor sensing and the perception of degradation products from cell wall damage. The modes of action of both FLS2 and PEPR1, which are members of the LRR-RKs family, highlight the intricate interplay between MAMP/PAMP recognition and the perception of cell wall degradation products (Figure 1; Table 1).
Table 1 Membrane localized Pattern-Recognition-Receptors (PRRs) and their specific DAMPS identified in in Arabidopsis thaliana.
WALL-ASSOCIATED KINASES (WAKs) are a type of pattern recognition receptors (PRRs) that are responsible for detecting oligogalacturonides (OGs), the degradation products of pectin (Brutus et al., 2010). The WAK-like (WAKL) family, which comprises 26 members in Arabidopsis thaliana, plays a crucial role in this process (Verica and He, 2002). More specifically, WAK1 and WAK2 are receptors that can bind OGs, yet require Ca2+ for binding to the OG backbone (Vallarino and Osorio, 2012; Benedetti et al., 2015). While WAK1 is not directly involved in early PTI, it eventually forms a complex with FLS2 (Danna et al., 2012). WAKL5 and WALK7 are induced in response to mechanical damage (Verica et al., 2003). Following the perception of OGs, WAK1 and WAK2 activate downstream signaling pathways via stimulating a Ca2+ influx, reactive oxygen species (ROS) production, CDPK activation, and MAPK3,6 phosphorylation, all of which can subsequently activate the transcription of genes linked with the defense response (Brutus et al., 2010; Galletti et al., 2011; Kohorn and Kohorn, 2012; Gravino et al., 2015). The redundancy among the WAKL genes complicates the comprehensive characterization of specific functions; as such, further investigation is required because distinct WAKL members appear to be differentially involved in the specific defense responses against pests across various crop species (Li et al., 2009; Hurni et al., 2015; Zuo et al., 2015; Tripathi et al., 2021; Barghahn et al., 2021). Another PRR receptor that is activated upon OG binding is the PROLINE-RICH EXTENSIN-LIKE RECEPTOR KINASE 4 (PERK4). Unlike members of the WAK family, PERK4 binds pectin and is involved in cell-wall loosening. Remarkably, PERK4 can inhibit root growth by stimulating ABA signaling and, intriguingly, disrupts Ca2+ homeostasis to induce CW loosening under mechanical stress (Bai et al., 2009). LYSIN MOTIF-RECEPTOR KINASE 5 (LYK5) and CHITIN ELICITOR RECEPTOR KINASE 1 (CERK1/LYK1) represent well-documented PRRs that are essential for the recognition of chitin, which is a fungal elicitor (Petutschnig et al., 2010; Liu et al., 2012; Cao et al., 2014). CERK1, in particular, has a critical role in glycan-based MAMP perception and was recently identified as vital for 1,3-β-D-glucan-triggered immunity in Plectosphaerella cucumerina (Mélida et al., 2018). CERK1 initiates ROS production via the Rho GTPase pathway, which triggers an oxidative burst that prompts a Ca2+ influx. Upon sensing this transient increase in Ca2+ levels, CDPK activates downstream signaling cascades that trigger specific immune responses (Cao et al., 2014; Keinath et al., 2015; Espinoza et al., 2017; Yuan et al., 2017).
Recently, MALECTIN-LIKE RECEPTOR KINASES (MLRKs) or Catharanthus roseus RECEPTOR-LIKE KINASE 1-LIKE PROTEINS (CrRLK1Ls) have received increased research attention. At present, 17 members of this family have been identified, with 10 well-characterized in Arabidopsis thaliana (Lindner et al., 2012; Li et al., 2016; Nissen et al., 2016; Franck et al., 2018). Prior research has shown that CrRLK1Ls are involved in various cellular processes, including cell growth, morphogenesis, reproduction, hormone signaling, immunity, and stress responses (Duan et al., 2010; Schoenaers et al., 2017; Feng et al., 2018; Solis-Miranda et al., 2021).
The THESEUS1 (THE1) and FERONIA/SIRENE (FER/SIR) receptors, both of which are MLRKs, were recently found to perceive cell wall damage as well as play key roles in innate plant immunity (Franck et al., 2018; Bacete and Hamann, 2020; Gonneau et al., 2018). In this signaling cascade, THE1 operates upstream of MID1-COMPLEMENTING ACTIVITY 1 (MCA1), with the activation of signaling ultimately inducing jasmonic acid (JA), salicylic acid (SA), and lignin production (Figure 1, Denness et al., 2011); this chain of signaling hints at collaborative interactions between maintenance of CW integrity and immune responses during the reaction to biotic stressors (Furuichi et al., 2012; Engelsdorf et al., 2018; Basu and Haswell, 2020; Hématy et al., 2007). Research has also revealed that THE1 interacts with GUANINE EXCHANGE FACTOR4 (GEF4) to elicit downstream responses, yet further exploration is needed to completely unravel the signaling pathway (Qu et al., 2017). FER, a member of the CrRLK1Ls family, is also involved in CW integrity maintenance and has a direct impact on innate immunity. More specifically, FER can bind rapid alkalinization 23 (RALF23; peptide secreted in response to a rapid alkalinization of the extracellular compartment; Blackburn et al., 2020) peptide OGs and trigger cell specific Ca2+ fluxes, especially in response to salt stress; thus, this receptor contributes to CW integrity stability (Feng et al., 2018). Recent research has revealed that FER activity positively influences PTI by enhancing interactions between FLS2, BAK1, and EFR (Stegmann et al., 2017; Smakowska-Luzan et al., 2018).
The recently identified CELLO-OLIGOMER RECEPTOR 1 (CORK1)/IMPAIRED IN GLYCAN PERCEPTION 1 (IGP1), which is an LRR/MALECTIN-RECEPTOR-LIKE KINASE (LRR-MAL-RLK), is involved in activating PTI upon the recognition of cello-oligomers, such as cellotriose, which are another degradation products of cellulose (Tseng et al., 2022; Martin-Dacal et al., 2022). In addition, IGP4 has been shown to be involved in plant immunity not only through the perception of cell wall damage, but also via the stimulation of Ca2+ influx following the binding of β-1,3/1,4 glucans and glucan-derived oligosaccharides (Martín-Dacal et al., 2023; and Rebaque et al., 2021). However, the downstream signaling mechanisms of the associated PRR have not yet been fully elucidated. Despite evidence that several CrRLK1Ls serve as sensors of CW integrity (Shimizu et al., 2010), the precise intracellular targets of this class of proteins, along with the signaling pathways involved, remain incompletely understood. As such, extensive experimental data is needed to better illuminate cell wall remodeling following exposure to both biotic and abiotic stressors. Moreover, further investigation of the signaling network(s) through which the newly identified CW integrity sensors transmit information across the cell wall is needed (Table 1). The comprehensive characterization of FER exemplifies how cell-specific Ca2+ signals are triggered, and underscores the need for further research, which could potentially lead to the identification of additional cell-specific receptors and the associated Ca2+ signatures.
In another study, Arabidopsis plant tissue was treated with CW fragments, and the results demonstrated how exposure to cellobiose elicited the strongest cytosolic Ca2+ spike. Interestingly, separate treatment with the MAMP-peptide flg22 and cellobiose (DAMP) returned distinct calcium spikes, both of which had a quantifiable pattern (Souza et al., 2017). This finding suggests that CW damages perception and PAMP recognition might operate synergistically, even if the responses exhibit distinct Ca2+ signatures; nevertheless, the evidence suggests that either can induce an immune response when activated alone. This novel perspective warrants further exploration of the intricacies of CW damages perception and PTI, as the conventional focus on individual elicitors might not comprehensively reflect all of the simultaneous environmental interactions that occur in plants following an attack by a pathogen.
In summary, numerous PRRs/RLKs demonstrate distinct and precise Ca2+ spikes (Figure 1), frequently transmitted through CDPKs, when activated; this knowledge suggests that these receptors have a pivotal role in calcium-mediated signaling within plants. Deciphering which calcium-dependent signaling pathways are activated by distinct RLKs following damage perception has the potential to offer invaluable insight for the field of plant immunity.
Upon pathogen detection at the CW, diverse PRR signaling pathways coincide with rapid Ca2+ influx into the cytoplasm (Figure 1; Clapham, 2007; Boller and Felix, 2009; Yu et al., 2017; DeFalco and Zipfel, 2021). Ca2+ serves as a universal secondary messenger, yet can also exhibit specific concentration patterns which plant cells decode to adequately activate the immune response. Termed Ca2+ signatures, these transient fluctuations in Ca2+ levels depend on factors like duration and amplitude (Knight, 1996). Ca2+ signaling comprises three stages: (1) encoding a specific trigger via Ca2+ flux magnitude and duration; (2) decoding of these signals by specific sensor proteins; and (3) initiation of downstream responses (Köhler et al., 1999; Bhar et al., 2023; Köster et al., 2022). Ca2+ signature features can be determined by the type of PAMPs/MAMPs and their concentration (Yu et al., 2017). A Ca2+ influx primarily involves CYCLIC NUCLEOTIDE-GATED CHANNELS (CNGCs) (Kohler et al., 1999), GLUTAMATE RECEPTOR-LIKE CHANNELS (GLRs) (Lam et al., 1998; Wudick et al., 2018; Alfieri et al., 2020), and REDUCED HYPEROSMOLARITY INDUCED Ca2+ INCREASE (OSCA1) (Yuan et al., 2014). The efflux of calcium ions involves Ca2+/H+ exchangers (CAXs) and autoinhibited Ca2+-ATPases (ACAs) (Geisler et al., 2000; Shigaki and Hirschi, 2000; García Bossi et al., 2020). PRRs can induce transient Ca2+ increases via three mechanisms: the generation of signaling molecules like ROS or cyclic nucleotide monophosphate (cNMP); direct activation of ion channels by binding to, and activating, Ca2+ pumps or channels; and the stimulation of downstream RLCKs to trigger ROS production and Ca2+ influx. For example, in Arabidopsis, during PAMP-induced signalling, two genes encoding the Ca2+ channels CNGC2 and CNGC4 are required (Ma et al., 2012; Tian et al., 2019). CNGC2 and CNGC4 can form a channel that is phosphorylated by the effector-kinase BIK1, thus triggering Ca2+ influx into the cytosol (Tian et al., 2019). Studies also reported that PEPR/Pep-signalling during DAMP-triggered immunity generates Ca2+ burst through activation of CNGC2 and act synergistically with PAMP-receptor FLS2 (Ma et al., 2012). Hence, the CNGC-mediated calcium influx establishes a crucial connection between the calcium-dependent immunity during PAMP-triggered immunity signalling pathways and PRR complex. Interestingly, it was also shown that the MRLK FER, involved in CW damages perception and CW integrity maintenance, can induce cell-specific calcium burst upon salt stress (Feng et al., 2018).
These changes in cytosolic Ca2+ levels are sensed by Ca2+ SENSOR PROTEINS (CSPs), which relay information through downstream cascades (Figure 1; Ranty et al., 2016). CSPs encompass CALMODULIN (CaM), CaM-LIKE PROTEINS (CMLs), CALCINEURIN B-LIKE PROTEINS (CBLs), and CALCIUM-DEPENDENT PROTEIN KINASES (CDPKs; CPKs in Arabidopsis) (Cheng et al., 2002; Yang and Poovaiah, 2003; Luan, 2009; Batistič and Kudla, 2012; Delormel and Boudsocq, 2019). CBLs interact with CBL-INTERACTING KINASES (CIPKs), while CDPKs are well-documented Ca2+ sensors that include phosphorylation sites within the CaM binding domain (Larrainzar et al., 2007; Xie et al., 2012). In response to changes in Ca2+ levels, CDPKs activate the kinase domains of downstream signaling molecules (Harmon et al., 1994; Harper et al., 1994; Yoo and Harmon, 1996; Schulz et al., 2013). As such, CIPKs/CDPKs transform pathogen-induced Ca2+ signals into phosphorylation events (Seybold et al., 2014; Delormel and Boudsocq, 2019). CDPKs directly influence ROS production, Ca2+ influx and transcriptional reprogramming (Kobayashi et al., 2007; Boudsocq and Sheen, 2013; Dubiella et al., 2013; Shinya et al., 2014). In Arabidopsis, AtCPK4, AtCPK5, AtCPK6 and AtCPK11 are described as early transcriptional regulators in MAMP-signalling pathway (Boudsocq et al., 2010). In Solanum tuberosum (St), StCDPK4/5 (At homologues: AtCPK5/6) induce ROS production by direct phosphorylation of the NADPH oxidase RBOHB (Kobayashi et al., 2012). Although many studies and advances put CDPKs as a central regulator of Ca2+-mediated stress and immune responses, further research, particularly studies involving elicitor treatment, is needed if we are to comprehensively understand the myriad roles of CDPKs in the immune response.
Well-documented Ca2+ sensors, like CCaMK, possess multiple phosphorylation sites (Larrainzar et al., 2007; Xie et al., 2012). This means that Ca2+ sensors could participate in various signaling pathways, and more research in Arabidopsis is needed to clarify the roles of these sensors in immunity, such as decoding the Ca2+ spikes that arise following DAMP release and perception. While the role of Ca2+ influx in plant immunity is already quite well understood, clarifying the specific targets of various Ca2+ signatures remain an understudied aspect of the response to pathogens. This is especially relevant for differences in Ca2+ influx signatures are particularly relevant during ETI and HR.
Recent research has unveiled the intricate interplay between calcium-mediated signaling and the triggering of immune responses by the perception of pathogens and CW damage. Despite advancements in the field, a comprehensive understanding of how distinct Ca2+ signaling patterns correspond to specific elicitors, as well as how these patterns translate into effective immune reactions against pathogens, is lacking. To address these knowledge gaps, future research should apply the latest conceptual frameworks and analytical tools. High-resolution techniques, which could be used to monitor transient Ca2+ fluxes, are imperative for locating the subcellular sites at which cytosolic calcium fluxes occur. Furthermore, unraveling the intricate networks involving Ca2+ receptors could provide insight into how distinct Ca2+ signatures correspond with certain target proteins. In addition, understanding the interactions between Ca2+ and EF-hand domains in Ca2+ sensors could clarify the underlying mechanisms of these signaling proteins.
RLKs, particularly PRRs, are inducing Ca2+ bursts after activation and then modulate the downstream signalling necessary for PTI. However, the specific Ca2+ signatures induced by each specific PRRs remain enigmatic. Investigating calcium pumps at the single-cell level, could offer valuable insight into the dynamics of Ca2+ fluxes and the respective targets of this form of signaling. Ca2+ signaling has been shown to influence cell wall perception and modification, both of which are crucial aspects of plant defense against pathogen infection. More specifically, Ca2+ signaling regulates the activities of CWDE and the deposition of reinforcing components. This intricate interplay results in the finely tuned cell wall modifications that are pivotal to defense mechanisms in plants. In this way, plants can stimulate specific signaling pathways to fortify cell walls in a bid to hinder pathogen entry and constrain pathogen proliferation. In summary, exploring Ca2+ signaling in the context of plant immunity, especially the early detection of pathogens via cell wall damage perception, is critical to advancing our understanding of plant resilience.
JG: Figure design, Writing – original draft. PM: Writing – original draft, Funding acquisition, Supervision, Writing – review & editing.
The author(s) declare financial support was received for the research, authorship, and/or publication of this article. This work was supported by funds that were awarded to PM (Vetenskapsrådet grant 2019-05634, Knut and Alice Wallenberg Foundation (KAW 2022.0029-Fate)).
We apologize to authors whose relevant work we have not cited, either inadvertently or due to space restrictions. We would like to thank Xuemin Ma, Muhammad Anjam, and Vaughan Hurry for their constructive comments and suggestions on the manuscript.
The authors declare that the research was conducted in the absence of any commercial or financial relationships that could be construed as a potential conflict of interest.
The author(s) PM declared that they were an editorial board member of Frontiers, at the time of submission. This had no impact on the peer review process and the final decision.
All claims expressed in this article are solely those of the authors and do not necessarily represent those of their affiliated organizations, or those of the publisher, the editors and the reviewers. Any product that may be evaluated in this article, or claim that may be made by its manufacturer, is not guaranteed or endorsed by the publisher.
Alfieri A., Doccula F. G., Pederzoli R., Grenzi M., Bonza M. C., Luoni L., et al. (2020). The structural bases for agonist diversity in an Arabidopsis thaliana glutamate receptor-like channel. Proc. Natl. Acad. Sci. U.S.A. 117, 752–760. doi: 10.1073/pnas.1905142117
Bacete L., Hamann T. (2020). The role of mechanoperception in plant cell wall integrity maintenance. Plants 9, 574. doi: 10.3390/plants9050574
Bacete L., Mélida H., Pattathil S., Hahn M. G., Molina A., Miedes E. (2017). “Characterization of plant cell wall damage-associated molecular patterns regulating immune responses,” in Plant pattern recognition receptors, methods in molecular biology. Eds. Shan L., He P. (New York, NY: Springer New York), 13–23. doi: 10.1007/978-1-4939-6859-6_2
Bai L., Ma X., Zhang G., Song S., Zhou Y., Gao L., et al. (2014). A receptor-like kinase mediates ammonium homeostasis and is important for the polar growth of root hairs in arabidopsis. Plant Cell 26, 1497–1511. doi: 10.1105/tpc.114.124586
Bai L., Zhang G., Zhou Y., Zhang Z., Wang W., Du Y., et al. (2009). Plasma membrane-associated proline-rich extensin-like receptor kinase 4, a novel regulator of Ca 2+ signalling, is required for abscisic acid responses in Arabidopsis thaliana. Plant J. 60, 314–327. doi: 10.1111/j.1365-313X.2009.03956.x
Barghahn S., Arnal G., Jain N., Petutschnig E., Brumer H., Lipka V. (2021). Mixed Linkage β-1,3/1,4-Glucan Oligosaccharides Induce Defense Responses in Hordeum vulgare and Arabidopsis thaliana. Front. Plant Sci. 12. doi: 10.3389/fpls.2021.682439
Basu D., Haswell E. S. (2020). The mechanosensitive ion channel MSL10 potentiates responses to cell swelling in arabidopsis seedlings. Curr. Biol. 30, 2716–2728.e6. doi: 10.1016/j.cub.2020.05.015
Batistič O., Kudla J. (2012). Analysis of calcium signaling pathways in plants. Biochim. Biophys. Acta (BBA) - Gen. Subj. 1820, 1283–1293. doi: 10.1016/j.bbagen.2011.10.012
Benedetti M., Pontiggia D., Raggi S., Cheng Z., Scaloni F., Ferrari S., et al. (2015). Plant immunity triggered by engineered in vivo release of oligogalacturonides, damage-associated molecular patterns. Proc. Natl. Acad. Sci. U.S.A. 112, 5533–5538. doi: 10.1073/pnas.1504154112
Bhar A., Chakraborty A., Roy A. (2023). The captivating role of calcium in plant-microbe interaction. Front. Plant Sci. 14. doi: 10.3389/fpls.2023.1138252
Bidlack J. (1992). Molecular structure and component integration of secondary cell walls in plants. 72, 51–56.
Blackburn M. R., Haruta M., Moura D. S. (2020). Twenty years of progress in physiological and biochemical investigation of RALF peptides. Plant Physiol. 182, 1657–1666. doi: 10.1104/pp.19.01310
Boller T., Felix G. (2009). A renaissance of elicitors: perception of microbe-associated molecular patterns and danger signals by pattern-recognition receptors. Annu. Rev. Plant Biol. 60, 379–406. doi: 10.1146/annurev.arplant.57.032905.105346
Boudsocq M., Sheen J. (2013). CDPKs in immune and stress signaling. Trends Plant Sci. 18, 30–40. doi: 10.1016/j.tplants.2012.08.008
Boudsocq M., Willmann M. R., McCormack M., Lee H., Shan L., He P., et al. (2010). Differential innate immune signalling via Ca2+ sensor protein kinases. Nature 464, 418–422. doi: 10.1038/nature08794
Brutus A., Sicilia F., Macone A., Cervone F., De Lorenzo G. (2010). A domain swap approach reveals a role of the plant wall-associated kinase 1 (WAK1) as a receptor of oligogalacturonides. Proc. Natl. Acad. Sci. U.S.A. 107, 9452–9457. doi: 10.1073/pnas.1000675107
Bush D. S. (1995). Calcium regulation in plant cells and its role in signaling. Annu. Rev. Plant Physiol. Plant Mol. Biol. 46, 95–122.
Cao Y., Liang Y., Tanaka K., Nguyen C. T., Jedrzejczak R. P., Joachimiak A., et al. (2014). The kinase LYK5 is a major chitin receptor in Arabidopsis and forms a chitin-induced complex with related kinase CERK1. eLife 3, e03766. doi: 10.7554/eLife.03766
Cassab G. I. (1998). PLANT CELL WALL PROTEINS. Annu. Rev. Plant Physiol. Plant Mol. Biol. 49, 281–309. doi: 10.1146/annurev.arplant.49.1.281
Cheng S.-H., Willmann M. R., Chen H.-C., Sheen J. (2002). Calcium signaling through protein kinases. The arabidopsis calcium-dependent protein kinase gene family. Plant Physiol. 129, 469–485. doi: 10.1104/pp.005645
Chinchilla D., Zipfel C., Robatzek S., Kemmerling B., Nürnberger T., Jones J. D. G., et al. (2007). A flagellin-induced complex of the receptor FLS2 and BAK1 initiates plant defence. Nature 448, 497–500. doi: 10.1038/nature05999
Chisholm S. T., Coaker G., Day B., Staskawicz B. J. (2006). Host-microbe interactions: shaping the evolution of the plant immune response. Cell 124, 803–814. doi: 10.1016/j.cell.2006.02.008
Choi W., Miller G., Wallace I., Harper J., Mittler R., Gilroy S. (2017). Orchestrating rapid long-distance signaling in plants with Ca 2+ , ROS and electrical signals. Plant J. 90, 698–707. doi: 10.1111/tpj.13492
Cosgrove D. J. (2005). Growth of the plant cell wall. Nat. Rev. Mol. Cell Biol. 6, 850–861. doi: 10.1038/nrm1746
Cosgrove D. J., Jarvis M. C. (2012). Comparative structure and biomechanics of plant primary and secondary cell walls. Front. Plant Sci. 3. doi: 10.3389/fpls.2012.00204
Danna C. H., Zhang X.-C., Khatri A., Bent A. F., Ronald P. C., Ausubel F. M., et al. (2012). FLS2-Mediated Responses to Ax21-Derived Peptides: Response to the Mueller Commentary. Plant Cell 24, 3174–3176. doi: 10.1105/tpc.112.099275
Decreux A., Messiaen J. (2005). Wall-associated kinase WAK1 interacts with cell wall pectins in a calcium-induced conformation. Plant Cell Physiol. 46, 268–278. doi: 10.1093/pcp/pci026
Decreux A., Thomas A., Spies B., Brasseur R., Cutsem P., Messiaen J. (2006). In vitro characterization of the homogalacturonan-binding domain of the wall-associated kinase WAK1 using site-directed mutagenesis. Phytochemistry 67, 1068–1079. doi: 10.1016/j.phytochem.2006.03.009
DeFalco T. A., Zipfel C. (2021). Molecular mechanisms of early plant pattern-triggered immune signaling. Mol. Cell 81, 3449–3467. doi: 10.1016/j.molcel.2021.07.029
Delormel T., Boudsocq M. (2019). Properties and functions of calcium-dependent protein kinases and their relatives in Arabidopsis thaliana. New Phytol. 224, 585–604. doi: 10.1111/nph.16088
Denness L., McKenna J. F., Segonzac C., Wormit A., Madhou P., Bennett M., et al. (2011). Cell wall damage-induced lignin biosynthesis is regulated by a reactive oxygen species- and jasmonic acid-dependent process in arabidopsis. Plant Physiol. 156, 1364–1374. doi: 10.1104/pp.111.175737
Duan Q., Kita D., Li C., Cheung A. Y., Wu H.-M. (2010). FERONIA receptor-like kinase regulates RHO GTPase signaling of root hair development. Proc. Natl. Acad. Sci. U.S.A. 107, 17821–17826. doi: 10.1073/pnas.1005366107
Dubiella U., Seybold H., Durian G., Komander E., Lassig R., Witte C.-P., et al. (2013). Calcium-dependent protein kinase/NADPH oxidase activation circuit is required for rapid defense signal propagation. Proc. Natl. Acad. Sci. U.S.A. 110, 8744–8749. doi: 10.1073/pnas.1221294110
Engelsdorf T., Gigli-Bisceglia N., Veerabagu M., McKenna J. F., Vaahtera L., Augstein F., et al. (2018). The plant cell wall integrity maintenance and immune signaling systems cooperate to control stress responses in Arabidopsis thaliana. Sci. Signal. 11, eaao3070. doi: 10.1126/scisignal.aao3070
Engelsdorf T., Hamann T. (2014). An update on receptor-like kinase involvement in the maintenance of plant cell wall integrity. Ann. Bot. 114, 1339–1347. doi: 10.1093/aob/mcu043
Engelsdorf T., Will C., Hofmann J., Schmitt C., Merritt B. B., Rieger L., et al. (2016). Cell wall composition and penetration resistance against the fungal pathogen Colletotrichum higginsianum are affected by impaired starch turnover in Arabidopsis mutants. Journal of Exp. Botany 68 (3), 701–713, erw434. doi: 10.1093/jxb/erw434
Espinoza C., Liang Y., Stacey G. (2017). Chitin receptor CERK 1 links salt stress and chitin-triggered innate immunity in Arabidopsis. Plant J. 89, 984–995. doi: 10.1111/tpj.13437
Feng W., Kita D., Peaucelle A., Cartwright H. N., Doan V., Duan Q., et al. (2018). The FERONIA Receptor Kinase Maintains Cell-Wall Integrity during Salt Stress through Ca2+ Signaling. Curr. Biol. 28, 666–675.e5. doi: 10.1016/j.cub.2018.01.023
Franck C. M., Westermann J., Boisson-Dernier A. (2018). Plant malectin-like receptor kinases: from cell wall integrity to immunity and beyond. Annu. Rev. Plant Biol. 69, 301–328. doi: 10.1146/annurev-arplant-042817-040557
Furuichi T., Iida H., Sokabe M., Tatsumi H. (2012). Expression of Arabidopsis MCA1 enhanced mechanosensitive channel activity in the Xenopus laevis oocyte plasma membrane. Plant Signaling Behav. 7, 1022–1026. doi: 10.4161/psb.20783
Gachomo E. W., Jno Baptiste L., Kefela T., Saidel W. M., Kotchoni S. O. (2014). The Arabidopsis CURVY1 (CVY1) gene encoding a novel receptor-like protein kinase regulates cell morphogenesis, flowering time and seed production. BMC Plant Biol. 14, 221. doi: 10.1186/s12870-014-0221-7
Gale U., Kemat A., Krens F. (2019). Effect of calcium (Ca2+) on pectin and pectin methyl esterases (PME) in normal and hyperhydric leaves of arabidopsis cultured in vitro.
Galindo-Trigo S., Blanco-Touriñán N., DeFalco T. A., Wells E. S., Gray J. E., Zipfel C., et al. (2020). CrRLK 1L receptor-like kinases HERK 1 and ANJEA are female determinants of pollen tube reception. EMBO Rep. 21, e48466. doi: 10.15252/embr.201948466
Galletti R., Ferrari S., De Lorenzo G. (2011). Arabidopsis MPK3 and MPK6 Play Different Roles in Basal and Oligogalacturonide- or Flagellin-Induced Resistance against Botrytis cinerea. Plant Physiol. 157, 804–814. doi: 10.1104/pp.111.174003
García Bossi J., Kumar K., Barberini M. L., Domínguez G. D., Rondón Guerrero Y. D. C., Marino-Buslje C., et al. (2020). The role of P-type IIA and P-type IIB Ca2+-ATPases in plant development and growth. J. Exp. Bot. 71, 1239–1248. doi: 10.1093/jxb/erz521
Geisler M., Axelsen K. B., Harper J. F., Palmgren M. G. (2000). Molecular aspects of higher plant P-type Ca2+-ATPases. Biochim. Biophys. Acta (BBA) - Biomembranes 1465, 52–78. doi: 10.1016/S0005-2736(00)00131-0
Gigli-Bisceglia N., Engelsdorf T., Hamann T. (2020). Plant cell wall integrity maintenance in model plants and crop species-relevant cell wall components and underlying guiding principles. Cell. Mol. Life Sci. 77, 2049–2077. doi: 10.1007/s00018-019-03388-8
Gigli-Bisceglia N., van Zelm E., Huo W., Lamers J., Testerink C. (2022). Arabidopsis root responses to salinity depend on pectin modification and cell wall sensing. Development 149, dev200363. doi: 10.1242/dev.200363
Glazebrook J. (2005). Contrasting mechanisms of defense against biotrophic and necrotrophic pathogens. Annu. Rev. Phytopathol. 43, 205–227. doi: 10.1146/annurev.phyto.43.040204.135923
Gonneau M., Desprez T., Martin M., Doblas V. G., Bacete L., Miart F., et al. (2018). Receptor kinase THESEUS1 is a rapid alkalinization factor 34 receptor in arabidopsis. Curr. Biol. 28, 2452–2458.e4. doi: 10.1016/j.cub.2018.05.075
Gómez-Gómez, Boller. (2000). P. O. FLS2: an LRR receptor–like kinase involved in the perception of the bacterial elicitor flagellin in arabidopsis. Mol. Cell.
Gravino M., Savatin D. V., Macone A., De Lorenzo G. (2015). Ethylene production in B otrytis cinerea- and oligogalacturonide-induced immunity requires calcium-dependent protein kinases. Plant J. 84, 1073–1086. doi: 10.1111/tpj.13057
Harmon A. C., Yoo B.-C., McCaffery C. (1994). Pseudosubstrate inhibition of CDPK, a protein kinase with a calmodulin-like domain. Biochemistry 33, 7278–7287. doi: 10.1021/bi00189a032
Harper J. F., Huang J.-F., Lloyd S. J. (1994). Genetic identification of an autoinhibitor in CDPK, a protein kinase with a calmodulin-like domain. Biochemistry 33, 7267–7277. doi: 10.1021/bi00189a031
Haruta M., Sabat G., Stecker K., Minkoff B. B., Sussman M. R. (2014). A peptide hormone and its receptor protein kinase regulate plant cell expansion. Science 343, 408–411. doi: 10.1126/science.1244454
Heese A., Hann D. R., Gimenez-Ibanez S., Jones A. M. E., He K., Li J., et al. (2007). The receptor-like kinase SERK3/BAK1 is a central regulator of innate immunity in plants. Proc. Natl. Acad. Sci. U.S.A. 104, 12217–12222. doi: 10.1073/pnas.0705306104
Hématy K., Sado P.-E., Van Tuinen A., Rochange S., Desnos T., Balzergue S., et al. (2007). A receptor-like kinase mediates the response of arabidopsis cells to the inhibition of cellulose synthesis. Curr. Biol. 17, 922–931. doi: 10.1016/j.cub.2007.05.018
Hepler P. K., Winship L. J. (2010). Calcium at the cell wall-cytoplast interface. J. Integr. Plant Biol. 52, 147–160. doi: 10.1111/j.1744-7909.2010.00923.x
Huffaker A., Pearce G., Ryan C. A. (2006). An endogenous peptide signal in Arabidopsis activates components of the innate immune response. Proc. Natl. Acad. Sci. U.S.A. 103, 10098–10103. doi: 10.1073/pnas.0603727103
Hurni S., Scheuermann D., Krattinger S. G., Kessel B., Wicker T., Herren G., et al. (2015). The maize disease resistance gene Htn1 against northern corn leaf blight encodes a wall-associated receptor-like kinase. Proc. Natl. Acad. Sci. U.S.A. 112, 8780–8785. doi: 10.1073/pnas.1502522112
Jarvis M. C. (1982). The proportion of calcium-bound pectin in plant cell walls. Planta 154, 344–346. doi: 10.1007/BF00393913
Jones J. D. G., Dangl J. L. (2006). The plant immune system. Nature 444, 323–329. doi: 10.1038/nature05286
Kaku H., Nishizawa Y., Ishii-Minami N., Akimoto-Tomiyama C., Dohmae N., Takio K., et al. (2006). Plant cells recognize chitin fragments for defense signaling through a plasma membrane receptor. Proc. Natl. Acad. Sci. U.S.A. 103, 11086–11091. doi: 10.1073/pnas.0508882103
Keinath N. F., Waadt R., Brugman R., Schroeder J. I., Grossmann G., Schumacher K., et al. (2015). Live Cell Imaging with R-GECO1 Sheds Light on flg22- and Chitin-Induced Transient [Ca 2+ ] cyt Patterns in Arabidopsis. Mol. Plant 8, 1188–1200. doi: 10.1016/j.molp.2015.05.006
Keller B. (1993). Structural cell wall proteins. Plant Physiol. 101, 1127–1130. doi: 10.1104/pp.101.4.1127
Knight H. (1996). Cold Calcium Signaling in Arabidopsis lnvolves Two Cellular Pools and a Change in Calcium Signature after Acclimation. The Plant Cell. 8, 489–503.
Kobayashi M., Ohura I., Kawakita K., Yokota N., Fujiwara M., Shimamoto K., et al. (2007). Calcium-dependent protein kinases regulate the production of reactive oxygen species by potato NADPH oxidase. Plant Cell 19, 1065–1080. doi: 10.1105/tpc.106.048884
Kobayashi M., Yoshioka M., Asai S., Nomura H., Kuchimura K., Mori H., et al. (2012). StCDPK5 confers resistance to late blight pathogen but increases susceptibility to early blight pathogen in potato via reactive oxygen species burst. New Phytol. 196, 223–237. doi: 10.1111/j.1469-8137.2012.04226.x
Kohler C., Merkle T., Neuhaus G. (1999). Characterisation of a novel gene family of putative cyclic nucleotide- and calmodulin-regulated ion channels in Arabidopsis thaliana. Plant J. 18, 97–104. doi: 10.1046/j.1365-313X.1999.00422.x
Kohorn B. D., Kohorn S. L. (2012). The cell wall-associated kinases, WAKs, as pectin receptors. Front. Plant Sci. 3. doi: 10.3389/fpls.2012.00088
Köster P., DeFalco T. A., Zipfel C. (2022). Ca 2+ signals in plant immunity. EMBO J. 41, e110741. doi: 10.15252/embj.2022110741
Krol E., Mentzel T., Chinchilla D., Boller T., Felix G., Kemmerling B., et al. (2010). Perception of the arabidopsis danger signal peptide 1 involves the pattern recognition receptor atPEPR1 and its close homologue atPEPR2. J. Biol. Chem. 285, 13471–13479. doi: 10.1074/jbc.M109.097394
Lam H.-M., Chiu J., Hsieh M.-H., Meisel L., Oliveira I. C., Shin M., et al. (1998). Glutamate-receptor genes in plants. Nature 396, 125–126. doi: 10.1038/24066
Larrainzar E., Wienkoop S., Weckwerth W., Ladrera R., Arrese-Igor C., González E. M. (2007). Medicago truncatula root nodule proteome analysis reveals differential plant and bacteroid responses to drought stress. Plant Physiol. 144, 1495–1507. doi: 10.1104/pp.107.101618
Li C., Wu H.-M., Cheung A. Y. (2016). FERONIA and her pals: functions and mechanisms. Plant Physiol. 171, 2379–2392. doi: 10.1104/pp.16.00667
Li H., Zhou S.-Y., Zhao W.-S., Su S.-C., Peng Y.-L. (2009). A novel wall-associated receptor-like protein kinase gene, OsWAK1, plays important roles in rice blast disease resistance. Plant Mol. Biol. 69, 337–346. doi: 10.1007/s11103-008-9430-5
Liang X., Zhou J.-M. (2018). Receptor-like cytoplasmic kinases: central players in plant receptor kinase–mediated signaling. Annu. Rev. Plant Biol. 69, 267–299. doi: 10.1146/annurev-arplant-042817-040540
Lindner H., Müller L. M., Boisson-Dernier A., Grossniklaus U. (2012). CrRLK1L receptor-like kinases: not just another brick in the wall. Curr. Opin. Plant Biol. 15, 659–669. doi: 10.1016/j.pbi.2012.07.003
Liu T., Liu Z., Song C., Hu Y., Han Z., She J., et al. (2012). Chitin-induced dimerization activates a plant immune receptor. Science 336, 1160–1164. doi: 10.1126/science.1218867
Lu D., Wu S., He P., Shan L. (2010). Phosphorylation of receptor-like cytoplasmic kinases by bacterial Flagellin. Plant Signaling Behav. 5, 598–600. doi: 10.4161/psb.11500
Luan S. (2009). The CBL–CIPK network in plant calcium signaling. Trends Plant Sci. 14, 37–42. doi: 10.1016/j.tplants.2008.10.005
Ma Y., Walker R. K., Zhao Y., Berkowitz G. A. (2012). Linking ligand perception by PEPR pattern recognition receptors to cytosolic Ca 2+ elevation and downstream immune signaling in plants. Proc. Natl. Acad. Sci. U.S.A. 109, 19852–19857. doi: 10.1073/pnas.1205448109
Martín-Dacal M., Fernández-Calvo P., Jiménez-Sandoval P., López G., Garrido-Arandía M., Rebaque D., et al. (2023). Arabidopsis immune responses triggered by cellulose- and mixed-linked glucan-derived oligosaccharides require a group of leucine-rich repeat malectin receptor kinases. Plant J. 113, 833–850. doi: 10.1111/tpj.16088
Martín-Dacal M., Fernández-Calvo P., Jiménez-Sandoval P., López G., Garrido-Arandía M., Rebaque D., et al. (2022). Arabidopsis Leucine Rich Repeat-Malectin Receptor Kinases in immunity triggered by cellulose and mixed-linked glucan oligosaccharides (preprint). Plant Biol. doi: 10.1101/2022.08.09.503277
Martín-Dacal M., Fernández-Calvo P., Jiménez-Sandoval P., López G., Garrido-Arandía M., Rebaque D., et al. (2022). Arabidopsis Leucine Rich Repeat-Malectin Receptor Kinases in immunity triggered by cellulose and mixed-linked glucan oligosaccharides (preprint). Plant Biol. doi: 10.1101/2022.08.09.503277
Mélida H., Sopeña-Torres S., Bacete L., Garrido-Arandia M., Jordá L., López G., et al. (2018). Non-branched β-1,3-glucan oligosaccharides trigger immune responses in Arabidopsis. Plant J. 93, 34–49. doi: 10.1111/tpj.13755
Miedes E., Vanholme R., Boerjan W., Molina A. (2014). The role of the secondary cell wall in plant resistance to pathogens. Front. Plant Sci. 5. doi: 10.3389/fpls.2014.00358
Miya A., Albert P., Shinya T., Desaki Y., Ichimura K., Shirasu K., et al. (2007). CERK1, a LysM receptor kinase, is essential for chitin elicitor signaling in Arabidopsis. Proc. Natl. Acad. Sci. U.S.A. 104, 19613–19618. doi: 10.1073/pnas.0705147104
Nissen K. S., Willats W. G. T., Malinovsky F. G. (2016). Understanding crRLK1L function: cell walls and growth control. Trends Plant Sci. 21, 516–527. doi: 10.1016/j.tplants.2015.12.004
Petutschnig E. K., Jones A. M. E., Serazetdinova L., Lipka U., Lipka V. (2010). The lysin motif receptor-like kinase (LysM-RLK) CERK1 is a major chitin-binding protein in arabidopsis thaliana and subject to chitin-induced phosphorylation. J. Biol. Chem. 285, 28902–28911. doi: 10.1074/jbc.M110.116657
Qu S., Zhang X., Song Y., Lin J., Shan X. (2017). THESEUS1 positively modulates plant defense responses against Botrytis cinerea through GUANINE EXCHANGE FACTOR4 signaling: THE1 functions in plant defense responses. J. Integr. Plant Biol. 59, 797–804. doi: 10.1111/jipb.12565
Ranty B., Aldon D., Cotelle V., Galaud J.-P., Thuleau P., Mazars C. (2016). Calcium sensors as key hubs in plant responses to biotic and abiotic stresses. Front. Plant Sci 7, 327. doi: 10.3389/fpls.2016.00327
Rebaque D., Del Hierro I., López G., Bacete L., Vilaplana F., Dallabernardina P., et al. (2021). Cell wall-derived mixed-linked β-1,3/1,4-glucans trigger immune responses and disease resistance in plants. Plant J. 106, 601–615. doi: 10.1111/tpj.15185
Roux M., Schwessinger B., Albrecht C., Chinchilla D., Jones A., Holton N., et al. (2011). The arabidopsis leucine-rich repeat receptor–like kinases BAK1/SERK3 and BKK1/SERK4 are required for innate immunity to hemibiotrophic and biotrophic pathogens. Plant Cell 23, 2440–2455. doi: 10.1105/tpc.111.084301
Schoenaers S., Balcerowicz D., Costa A., Vissenberg K. (2017). The kinase ERULUS controls pollen tube targeting and growth in arabidopsis thaliana. Front. Plant Sci. 8. doi: 10.3389/fpls.2017.01942
Schulz P., Herde M., Romeis T. (2013). Calcium-dependent protein kinases: hubs in plant stress signaling and development. Plant Physiol. 163, 523–530. doi: 10.1104/pp.113.222539
Shimizu T., Nakano T., Takamizawa D., Desaki Y., Ishii-Minami N., Nishizawa Y., et al. (2010). Two LysM receptor molecules, CEBiP and OsCERK1, cooperatively regulate chitin elicitor signaling in rice: LysM receptors for rice chitin signaling. Plant J. 64, 204–214. doi: 10.1111/j.1365-313X.2010.04324.x
Seybold H., Trempel F., Ranf S., Scheel D., Romeis T., Lee J. (2014). Ca2+ signalling in plant immune response: from pattern recognition receptors to Ca2+ decoding mechanisms. New Phytol. 204, 782–790. doi: 10.1111/nph.13031
Shigaki T., Hirschi K. (2000). Characterization of CAX-like genes in plants: implications for functional diversity. Gene 257, 291–298. doi: 10.1016/S0378-1119(00)00390-5
Shinya T., Yamaguchi K., Desaki Y., Yamada K., Narisawa T., Kobayashi Y., et al. (2014). Selective regulation of the chitin-induced defense response by the Arabidopsis receptor-like cytoplasmic kinase PBL27. Plant J. 79, 56–66. doi: 10.1111/tpj.12535
Smakowska-Luzan E., Mott G. A., Parys K., Stegmann M., Howton T. C., Layeghifard M., et al. (2018). An extracellular network of Arabidopsis leucine-rich repeat receptor kinases. Nature 553, 342–346. doi: 10.1038/nature25184
Snedden W. A., Fromm H. (2001). Calmodulin as a versatile calcium signal transducer in plants. New Phytol. 151, 35–66. doi: 10.1046/j.1469-8137.2001.00154.x
Solis-Miranda J., Quinto C. (2021). The CrRLK1L subfamily: One of the keys to versatility in plants. Plant Physiol. Biochem. 166, 88–102. doi: 10.1016/j.plaphy.2021.05.028
Souza C., de A., Li S., Lin A. Z., Boutrot F., Grossmann G., et al. (2017). Cellulose-derived oligomers act as damage-associated molecular patterns and trigger defense-like responses. Plant Physiol. 173, 2383–2398. doi: 10.1104/pp.16.01680
Souza C. D. A., Li S., Lin A. Z., Boutrot F., Grossmann G., Zipfel C., et al. (2017). Cellulose-derived oligomers act as damage-associated molecular patterns and trigger defense-like responses. Plant Physiol. 173, 2383–2398. doi: 10.1104/pp.16.01680
Stegmann M., Monaghan J., Smakowska-Luzan E., Rovenich H., Lehner A., Holton N., et al. (2017). The receptor kinase FER is a RALF-regulated scaffold controlling plant immune signaling. Science 355, 287–289. doi: 10.1126/science.aal2541
Tian W., Hou C., Ren Z., Wang C., Zhao F., Dahlbeck D., et al. (2019). A calmodulin-gated calcium channel links pathogen patterns to plant immunity. Nature 572, 131–135. doi: 10.1038/s41586-019-1413-y
Tripathi R. K., Aguirre J. A., Singh J. (2021). Genome-wide analysis of wall associated kinase (WAK) gene family in barley. Genomics 113, 523–530. doi: 10.1016/j.ygeno.2020.09.045
Tseng Y.-H., Scholz S. S., Fliegmann J., Krüger T., Gandhi A., Furch A. C. U., et al. (2022). CORK1 is required for cellooligomer-induced responses in arabidopsis thaliana. Cells 11, 2960. doi: 10.3390/cells11192960
Vaahtera L., Schulz J., Hamann T. (2019). Cell wall integrity maintenance during plant development and interaction with the environment. Nat. Plants 5, 924–932. doi: 10.1038/s41477-019-0502-0
Vallarino J. G., Osorio S. (2012). Signaling role of oligogalacturonides derived during cell wall degradation. Plant Signaling Behav. 7, 1447–1449. doi: 10.4161/psb.21779
van den Brink J., de Vries R. P. (2011). Fungal enzyme sets for plant polysaccharide degradation. Appl. Microbiol. Biotechnol. 91, 1477–1492. doi: 10.1007/s00253-011-3473-2
Van Der Does D., Boutrot F., Engelsdorf T., Rhodes J., McKenna J. F., Vernhettes S., et al. (2017). The Arabidopsis leucine-rich repeat receptor kinase MIK2/LRR-KISS connects cell wall integrity sensing, root growth and response to abiotic and biotic stresses. PloS Genet. 13, e1006832. doi: 10.1371/journal.pgen.1006832
Verica J. A., Chae L., Tong H., Ingmire P., He Z.-H. (2003). Tissue-specific and developmentally regulated expression of a cluster of tandemly arrayed cell wall-associated kinase-like kinase genes in arabidopsis. Plant Physiol. 133, 1732–1746. doi: 10.1104/pp.103.028530
Verica J. A., He Z.-H. (2002). The cell wall-associated kinase ( WAK ) and WAK -like kinase gene family. Plant Physiol. 129, 455–459. doi: 10.1104/pp.011028
Wan J., He M., Hou Q., Zou L., Yang Y., Wei Y., et al. (2021). Cell wall associated immunity in plants. Stress Biol. 1, 3. doi: 10.1007/s44154-021-00003-4
Wolf S. (2017). Plant cell wall signalling and receptor-like kinases. Biochem. J. 474, 471–492. doi: 10.1042/BCJ20160238
Wu H.-C., Bulgakov V. P., Jinn T.-L. (2018). Pectin methylesterases: cell wall remodeling proteins are required for plant response to heat stress. Front. Plant Sci. 9. doi: 10.3389/fpls.2018.01612
Wudick M. M., Michard E., Oliveira Nunes C., Feijó J. A. (2018). Comparing plant and animal glutamate receptors: common traits but different fates? J. Exp. Bot. 69, 4151–4163. doi: 10.1093/jxb/ery153
Xie F., Murray J. D., Kim J., Heckmann A. B., Edwards A., Oldroyd G. E. D., et al. (2012). Legume pectate lyase required for root infection by rhizobia. Proc. Natl. Acad. Sci. U.S.A. 109, 633–638. doi: 10.1073/pnas.1113992109
Yamaguchi Y., Huffaker A., Bryan A. C., Tax F. E., Ryan C. A. (2010). PEPR2 is a second receptor for the pep1 and pep2 peptides and contributes to defense responses in arabidopsis. Plant Cell 22, 508–522. doi: 10.1105/tpc.109.068874
Yang T., Poovaiah B. W. (2003). Calcium/calmodulin-mediated signal network in plants. Trends Plant Sci. 8, 505–512. doi: 10.1016/j.tplants.2003.09.004
Yoo B.-C., Harmon A. C. (1996). Intramolecular binding contributes to the activation of CDPK, a protein kinase with a calmodulin-like domain. Biochemistry 35, 12029–12037. doi: 10.1021/bi9606612
Yu X., Feng B., He P., Shan L. (2017). From chaos to harmony: responses and signaling upon microbial pattern recognition. Annu. Rev. Phytopathol. 55, 109–137. doi: 10.1146/annurev-phyto-080516-035649
Yuan P., Jauregui E., Du L., Tanaka K., Poovaiah B. (2017). Calcium signatures and signaling events orchestrate plant–microbe interactions. Curr. Opin. Plant Biol. 38, 173–183. doi: 10.1016/j.pbi.2017.06.003
Yuan F., Yang H., Xue Y., Kong D., Ye R., Li C., et al. (2014). OSCA1 mediates osmotic-stress-evoked Ca2+ increases vital for osmosensing in Arabidopsis. Nature 514, 367–371. doi: 10.1038/nature13593
Zhou J.-M., Zhang Y. (2020). Plant immunity: danger perception and signaling. Cell 181, 978–989. doi: 10.1016/j.cell.2020.04.028
Zipfel C. (2014). Plant pattern-recognition receptors. Trends Immunol. 35, 345–351. doi: 10.1016/j.it.2014.05.004
Keywords: cell wall, calcium, damage, immune responses, pathogens
Citation: Guerreiro J and Marhavý P (2023) Unveiling the intricate mechanisms of plant defense. Front. Plant Physiol. 1:1285373. doi: 10.3389/fphgy.2023.1285373
Received: 29 August 2023; Accepted: 19 October 2023;
Published: 13 November 2023.
Edited by:
Ondřej Plíhal, Palacký University, CzechiaReviewed by:
Markéta Pernisová, Masaryk University, CzechiaCopyright © 2023 Guerreiro and Marhavý. This is an open-access article distributed under the terms of the Creative Commons Attribution License (CC BY). The use, distribution or reproduction in other forums is permitted, provided the original author(s) and the copyright owner(s) are credited and that the original publication in this journal is cited, in accordance with accepted academic practice. No use, distribution or reproduction is permitted which does not comply with these terms.
*Correspondence: Peter Marhavý, cGV0ZXIubWFyaGF2eUBzbHUuc2U=
Disclaimer: All claims expressed in this article are solely those of the authors and do not necessarily represent those of their affiliated organizations, or those of the publisher, the editors and the reviewers. Any product that may be evaluated in this article or claim that may be made by its manufacturer is not guaranteed or endorsed by the publisher.
Research integrity at Frontiers
Learn more about the work of our research integrity team to safeguard the quality of each article we publish.