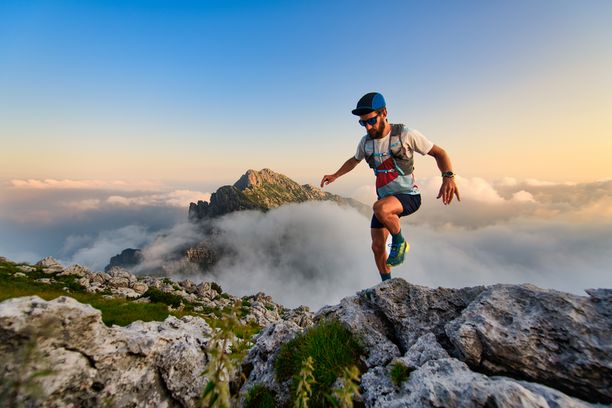
95% of researchers rate our articles as excellent or good
Learn more about the work of our research integrity team to safeguard the quality of each article we publish.
Find out more
ORIGINAL RESEARCH article
Front. Photobiol., 09 September 2024
Sec. Photoecology and Environmental Photobiology
Volume 2 - 2024 | https://doi.org/10.3389/fphbi.2024.1441713
This article is part of the Research TopicAquatic Phototrophic Communities: Insights into Light-driven Ecological DynamicsView all 3 articles
Microphytobenthos (MPB) are a diatom-dominated microbial community of primary producers that inhabit mudflat sediments. The benthic diatoms display photo-protective strategies to face extreme light variations susceptible to generate cellular oxidative stress. However, oxidative stress induces the production of reactive oxygen species (ROS) that generate oxylipins—oxygenated metabolites of polyunsaturated fatty acids (PUFAs) —which are among the known chemical mediators in diatoms. Non-enzymatically generated oxylipins known as “isoprostanoids” or “isofuranoids” are poorly studied in diatoms. To better understand the roles of the latter in migrational MPB light response, we investigated the effect of different irradiances corresponding to dark (D), low light (LL, 50 and 100 μmol. photons. m−2. s−1PAR), medium light (ML, 250 μmol. photons. m−2. s−1 PAR), and high light (HL, 500, 750, and 1000 μmol. photons. m−2. s−1 PAR) on isoprostanoid production by the biofilm’s organisms. The PUFA precursors of the varying oxylipins evidenced a diatom response to irradiance. Under 1000 PAR, the total amount of isoprotanoids increased, indicating an oxidative stress response. Isoprostanes (IsoPs) and prostaglandins (PGs) characterized HL conditions and evidenced lipid peroxidation, probably linked to the higher generation of ROS by photosynthesis. In contrast, phytoprostanes (PhytoPs) characterized LL and ML, where the ROS scavengers were probably not overwhelmed. This first investigation of non-enzymatic oxylipin production by a microphytobenthic biofilm under different irradiances highlighted the potential of exploring their possible signaling roles related to MPB light responses.
Microphytobenthos (MPB) are a microbial community of primary producers usually dominated by motile pennate diatoms (Haubois et al., 2005; Méléder et al., 2007; Ribeiro et al., 2013). MPB make a major contribution to the total primary production of the oceans, representing a substantial food source for invertebrates, fish, and wading birds (Beninger and Paterson, 2018; Macintyre et al., 1996; Underwood and Kromkamp, 1999; Werner et al., 2006) and having an essential role in local socioeconomic activities (Lebreton et al., 2019) and the global carbon cycle (Hope et al., 2020).
Those MPB present in mudflat sediments are subject to the strong variability characteristic of the intertidal environments which have notably high changes of solar irradiance (Prelle and Karsten, 2022; Woelfel et al., 2014). Diatoms, such as the other photosynthetic organisms, generate reactive oxygen species (ROS) as byproducts during photosynthesis. Under high irradiance exposure, the ROS production of PS II reaction centers can overwhelm the antioxidant systems of microalgae (Foyer, 2018). Because ROS are highly reactive, they can damage important cell components such as membranes and DNA (Dall’Osto et al., 2010; Havaux and Niyogi, 1999; Krieger-Liszkay et al., 2008; Triantaphylidès and Havaux, 2009). To cope with extreme intertidal light variations, which can cause cellular oxidative stress, benthic diatoms present in muddy sediment habitats employ both physiological and behavioral photo-protective strategies (Barnett et al., 2020; Cartaxana et al., 2011). Their physiological responses consist of dissipating excess energy through the non-photochemical quenching (NPQ) of chlorophyll (Chl), fluorescence, the adjustment of light-harvesting pigment production, and ROS detoxification (Lavaud and Goss, 2014; Lepetit et al., 2013; Nymark et al., 2009). Among environmental factors such as tide or inorganic carbon availability, high irradiances trigger the vertical migration of diatoms (Consalvey et al., 2004; Marques da Silva et al., 2017). This behavior is strongly suspected to be a behavioral photoprotective mechanism (Consalvey et al., 2004; Jesus et al., 2023). Indeed, it appears to be a strategy of diatoms to adapt their vertical positioning to their optimal photon irradiance threshold, which also depend on wavelength and the spectral quality of light (Cartaxana et al., 2011; Jesus et al., 2006; Prins et al., 2020; Serôdio et al., 2012).
Given that some studies have indicated the involvement of ROS in the signaling processes of microorganisms (D’Autréaux and Toledano, 2007), and considering their purposeful generation by plants to regulate various metabolic activities such as defense against pathogens, programmed cell death, and stomatal behavior (Apel and Hirt, 2004), our understanding of ROS has evolved in recent decades (Foyer et al., 2017; Noctor and Foyer, 2016). It now seems clear that their roles are diverse and are not only detrimental to cellular functioning. In diatoms, ROS are directly generated during photosynthesis and depend on its efficiency (Ezequiel et al., 2023; Krieger-Liszkay, 2005; Nishiyama et al., 2006). Therefore, they probably play an important role in the signal of photo-protective response induction such as NPQ and vertical migration. ROS can act locally but also as a signaling molecule by being transported to different organelles with maximal distances ranging from 1 nm for hydroxyl radical (•OH) to more than 1 µm for hydrogen peroxide (H2O2) (Dumanović et al., 2021; Knieper et al., 2023; Mittler, 2017). The oxidation by-products of ROS detoxification molecules such as oxidized glutathione (Meyer, 2008) and those given by 1O2 and carotenoids also can give rise to signaling molecules (Ramel et al., 2012). These byproducts can modulate various biological processes, including transcription, post-translational modification, and protein–protein interactions, notably by impacting the oxidation state of thiol groups in redox-sensitive proteins (Dietz, 2008; Meyer, 2008).
Oxylipins are among the byproducts generated by ROS reaction with polyunsaturated fatty acids (PUFAs), called “lipid peroxidation” (Améras et al., 2003; Jahn et al., 2008; Triantaphylidès et al., 2008). These compounds represent the best described signaling molecules in diatoms (Orefice et al., 2022; Ruocco et al., 2020). Oxylipins can influence other species’ abundance and fitness through their antipredator, antibacterial, info-chemical, and allelochemical functions (Meyer et al., 2018; Ruocco et al., 2020; Russo et al., 2020). They can be produced by several enzymatic and non-enzymatic processes, giving rise to an important diversity in structures (Galano et al., 2017; Gerwick et al., 1991; Longini et al., 2017). Enzymatic lipoxygenase pathways have been shown to be species-dependent in the marine diatom genus Pseudonitzschia (Lamari et al., 2013), while the oxylipin structures from non-enzymatic processes—isoprostanoids—depend only on the ROS reaction within a bis-allylic position of PUFAs’ double bonds in the cells (Galano et al., 2017). Non-enzymatic oxylipins are likely less species-specific, making isoprostanoids promising candidates for transmitting signals between various kingdoms of organisms present in the microphytobenthic biofilm. In addition, previous research has shown that the presence of H2O2 and copper in culture media induced C18-, C20-, and C22-derived isoprostanoid production changes in several diatoms and other microalgae species; some have been observed to trigger biological responses (Linares-Maurizi et al., 2023; Lupette et al., 2018; Vigor et al., 2020).These oxylipins could thus be involved in the MPB responses to physiological changes and environmental variations such as light exposure.
Oxylipin biosynthesis by microalgae, especially diatoms, has triggered much recent interest (Di Dato et al., 2020a; 2020b; 2019), but little emphasis has been placed on non-enzymatic pathways (Orefice et al., 2022; Vigor et al., 2020). In addition, their production in microphytobenthic biofilm has never been studied. Thus, to better understand the roles of the latter in migrational MPB light response, we investigated the effect of different irradiances corresponding to dark (D), low light (LL, 50 and 100 µmol photons m−2 s−1 PAR), medium light (ML, 250 µmol photons m−2 s−1 PAR), and high light (HL, 500, 750, and 1000 µmol photons m−2 s−1 PAR) on their presence in the biofilm’s organisms.
The biofilm samples used in this study were the same as those generated for our previous untargeted metabolomic analysis (Doose and Hubas, 2024). The first 2 cm of sediment present in an empty breeding pond of the Marine Station of Concarneau (France; 47°52.5804′N; 3°55.026′W) at low tide was sampled to collect the MPB biofilm. It was dominated by communities of epipelic diatoms where Pleurosigma formosum and Gyrosigma balticum appeared to be the two main species.
After 24 h under very low light conditions (PAR <6 µmol photons m⁻2 s⁻1) to allow settling, the top 5 mm of sediment containing MPB was re-sampled and re-suspended in 250 mL of filtered seawater to homogenize the biofilm. A volume of 6 mL of the homogenized biofilm was then added to 5-cm-diameter Petri dishes to ensure an equal amount of biofilm in each dish; the dishes were left for 24 h under very low light (PAR <6 µmol photons m⁻2 s⁻1) to allow biofilm reformation.
Five Petri dishes (n = 5) were then placed under dark (D), 50, 100 (LL), 250 (ML), 500, 750, and 1000 (HL) µmol. photons. m−2. s−1 PAR where light was generated by LEDs (SL 3500, white warm, Photon Systems Instruments). The Petri dishes containing the biofilm were shifted under the different irradiance concomitantly with the MPB presence at the sediment surface. After 30 min of exposure, liquid nitrogen was poured into the Petri dishes to immediately freeze the sediment without disturbance. The samples were freeze-dried and stored at −80°C awaiting subsequent analysis.
Non-enzymatic oxylipins were extracted using a protocol published in Vigor et al. (2018) on marine macroalgae with some modifications. For the extraction, 150 mg of dried biomass was placed in lysing matrix tubes (lysing matrix D, MP Biochemicals, Illkirch, France) with 25 µL of BHT (butylated hydroxytoluene 1% in methanol), 1 mL of H2O (HPLC grade), and 4 µL of Internal Standards Mixture (ISM n°18) (1 ng/μL). The sample was then ground using a FastPrep-24 (MP Biochemicals) at 6.5 m/s for 30 s. The mixture was transferred into a 15-mL Falcon tube with 3 × 1 mL of cold chloroform/methanol mixture (2:1) and was stirred with a vortex mixer for 30 s between each transfer. A volume of 0.5 mL phosphate buffer (50 mM, pH 2, prepared with NaH2PO4 and H3PO4, saturated in NaCl and stirred with a vortex mixer for 30 s) was added to the mixture. Then, 3 mL of the cold chloroform/methanol mixture (2:1) was added and stirred with a vortex for 30 s. The samples were then centrifuged at 4000 rpm for 5 min at 4 °C. The lower organic phase was collected in Pyrex tubes and was then dried using a SpeedVac apparatus at 60 °C for 1 h.
To extract the lipid fraction, the dried extract was hydrolyzed by adding 950 µL of 1 M KOH and incubated at 40 °C for 30 min with a vertical rotator (100 rpm). To the mixture was added 1 mL of 40 mM formic acid prior to starting the solid-phase extraction. Samples were then loaded on pre-conditioned Oasis mixed polymeric sorbent cartridges (Oasis MAX Cartridge, 60 mg, Waters). The undesired compound was then eliminated using 2 mL of NH3 2% (v/v), 2 mL of MeOH/20 mM formic acid (30:70; v/v), 2 mL of hexane, and 2 mL of hexane/ethyl acetate (70:30; v/v). Finally, isoprostanoids/isofuranoids/PG were eluted by adding 2 × 1 mL of a mixture of hexane/EtOH/acetic acid (70:29.4:0.6; v/v/v). The samples were dried using a SpeedVac at 60 °C for an average of 1 h.
The dried extracts were reconstituted with 100 µL of mobile phase solvents (H2O/ACN; 83:17; v/v) and then stirred via vortex, ultrasound 2 min, and then vortex, and later filtered in 0.45-µm Eppendorf (Nanosep Centrifugal Devices) with centrifugation at 10,000 rpm for 1 min at room temperature. A volume of 80 µL was transferred in an HPLC analytic vial for further analysis, and the remaining 20 µL was transferred in another HLPC vial for spiking QC. Note that for the QC, 4 µL of Prostamix GR57 SM0.5 contained all oxylipin standards at 0.5 ng/μL. The analysis was completed by injecting 5 µL of the extract into the micro-LC-MS/MS 5500 QTrap system, which uses high-performance liquid chromatography coupled with tandem mass spectrometry.
An Eksigent micro- High performance liquid chromatography (HPLC) 200 Plus (Sciex Applied Biosystems, Framingham, MA, United Statesa) equipped with CTC Analytics AG (Zwingen, Switzerland) was used; all analyses were performed on a HALO C18 analytical column (100 × 0.5 mm, 2.7 µm; Eksigent Technologies, CA, United Statesa) maintained at 40 °C. The mobile phases consisted of a binary gradient of H2O with 0.1% (v/v) HCO2H (solvent A) and ACN/MeOH 80:20 (v/v) (solvent B) with a flow rate of 0.03 mL.min−1 and an injection volume of 5 µL. The elution gradient was: 17% B at 0 min, 17% B at 2.6 min; 21% B at 2.85 min; 25% B at 7.3 min; 28.5% B at 8.8 min; 33.3% B at 11 min; 40% B at 15 min; 95% B at 16.5 min for 1.5 min.
Using electrospray ionization (ESI) in negative mode, mass spectrometry analyses were performed on an AB Sciex QTRAP 5500 (Sciex Applied Biosystems, ON, Canada). The source was maintained at −4.5 kV, and nitrogen flow served as curtain gas at 30 psi and a nebulization assist at 20 psi at room temperature.
In order to analyze the targeted compounds in a detection window of 90 s, the monitoring of the ionic fragmentation products of each deprotonated analyte [M-H]- molecule was carried out in multiple ion monitoring (MRM) detection mode using nitrogen as the collision gas. Two transitions for quantification (T1) and specification (T2) were predetermined by MS/MS analysis of standards. LC-MS/MS data acquisition was performed using Analyst® software (Sciex Applied Biosystems) to drive the mass spectrometer. The peak integration and quantification of analytes were processed by MultiQuant 3.0 software (Sciex Applied Biosystems).
Of the 54 oxylipin standards in hand from several omega 3 and 6 PUFAs, eight PhytoPs and two PhytoFs from α-linolenic acid (ALA), six IsoPs and one PG from eicosapentaenoic acid (EPA), four IsoPs and one PG from arachidonic acid (AA), one dihomo-IsoP from adrenic acid (AdA), seven neuroprostanes from docosahexaenoic acid (DHA) for the non-enzymatic oxylipins, and three prostaglandins are highlighted here (see “Results” below).
The mean de-epoxydation state data were calculated per light group (LL, 50-100 PAR; ML, 250 PAR; HL, 500,750, and 1000 PAR). The object generated by multiple factor analysis (MFA) performed on oxylipin measurement data was used to run a between-class analysis on R under the package ade4 following the original script available on Github (https://github.com/Hubas-prog/BC-MFA). The result is termed “BC-MFA”. The normality of the oxylipin data and their residual distribution was tested using the Shapiro–Wilk test. When residuals followed a normal distribution, one-way ANOVA was performed to detect significant light effects on oxylipin concentrations in the biofilm. When normality was not verified, a non-parametric Van der Waerden test was performed with the R package “agricolae”. Outliers were tested using the “1.5 times the interquartile range (IQR)” rule. The correlation test was performed on R with the Pearson method.
The LC-MS targeted analysis allowed the measurement and quantification of 29 oxylipins (Supplementary Material S1) from five different families: neuroprostanes (NeuroPs), isoprostranes (IsoPs), phytoprostanes (PhytoPs), phytofuranes (PhytoFs), and prostaglandins (PG). The BC-MFA analysis presented in Figure 1 shows the partitioning of MPB samples depending on their amount of oxylipin measured. The total inertia of the dataset explained (TIE) by the light treatments was 37%. The first dimension of the BC-MFA score plot distinguished the dark from the 1000 PAR treatment as well as the LL and ML from the HL treatments. The dark, LL, and ML treatments were characterized by oxylipins from the PhytoP family, while the HL were characterized by oxylipins from the IsoP and PG families. This analysis allowed identification of 12 compounds (out of 29) significantly influenced by this light gradient. The production of molecules forming the NeuroP and the PhytoF families were not affected by the irradiances.
Figure 1. Between-class (BC) analysis realized on a multiple factor analysis (A). MFA object (B). BC-MFA and its variables (C). on oxylipins measured in the biofilm exposed to dark (D, n = 10) and to irradiances of low (LL: 50, 100), medium (ML: 250), and high light (HL: 500, 750, 1000) in µmol. photons. m−2. s−1 PAR (n = 5).
The highest total amount of oxylipins presented in Figure 2 was measured in biofilm exposed to the 1000 PAR treatment with 484 ± 27 pg/mg dw. The lowest values were observed in biofilm under dark (383 ± 39 pg/mg dw), 250, and 500 PAR (385 ± 17 pg/mg dw for both). Significant differences were observed between biofilm under the 1000 PAR treatment and all the other irradiances, except for the 100 PAR conditions (ANOVA, p < 0.05). The major oxylipins, constituting more than 5% of the total oxylipin measured in the biofilm, included 18-epi-18-F3t-IsoP (EPA), 5-epi-5-F3t-IsoP (EPA), 5-F3t-IsoP, 9-epi-9-F1t-PhytoP + ent-16-F1t-PhytoP, 9-F1t-PhytoP, and PGF3.
Figure 2. Quantity of oxylipins in pg/mg dw per fatty acid precursors and in total measured in biofilm exposed to dark (D, n = 10), and to irradiances of low (LL: 50 and 100), medium (ML: 250), and high light (HL: 500, 750, and 1000) in µmol photons m−2 s−1 PAR (n = 5). AA (arachidonic acid), AdA (adrenic acid), ALA (α-linolenic acid), DHA (docosahexaenoic acid), EPA (eicosapentaenoic acid). Box plot represents median (middle line), 25th and 75th percentiles (ends of box), and minimum and maximum values (at bottom and top of line, respectively). Outliers were identified only in the total oxylipin data using the IQR for the 100, 250, 500, and 750 PAR conditions (for which n = 4). One-way ANOVA was performed on the data to detect significant differences between treatments, as indicated by letters (p < 0.05).
Figure 3 shows the proportion of the metabolite’s precursor. The oxylipins measured in the biofilm were mainly derived from eicosapentaenoic acid (EPA) (Guy et al., 2014; Morrow et al., 1990), representing more than 60% of the total amount. The total of α-linolenic acid (ALA) metabolites (Parchmann and Mueller, 1998) represented more than 10%, and arachidonic acid (AA) (Guy et al., 2014; Morrow et al., 1990) and docosahexaenoic acid (DHA) (Nourooz-Zadeh et al., 1998; Roberts et al., 1998) represented approximately 7% and 5%, respectively. One metabolite measured was derived from adrenic acid (AdA), representing less than 1% of the total FA precursor. Only the total of EPA and ALA metabolites varied under different irradiances. The total EPA metabolites increased with increasing irradiances from 250 PAR to 1000 PAR conditions. Conversely, the total ALA metabolites followed the exact opposite pattern, with the highest value under the 250 PAR condition. The 750 and 1000 PAR conditions are significantly lower than the dark, LL, and ML conditions. The total amount of oxylipins per FA precursor (Figure 2) showed significantly higher amounts of oxylipins derived from EPA under the 1000 PAR irradiance than in the other light conditions. Conversely, the amount of oxylipins derived from ALA was significantly higher under the 100 PAR irradiances than under HL. The variations in the EPA- and ALA-derived oxylipin percentages are thus explained by both the increase of EPA-derived compounds and the decrease of ALA-derived compounds.
Figure 3. Relative abundance distribution in percentage of oxidated polyunsaturated fatty acids (NEO-PUFAs) in the MPB biofilm: AA (arachidonic acid), AdA (adrenic acid, <1%), ALA (α-linolenic acid), DHA (docosahexaenoic acid), EPA (eicosapentaenoic acid). Significant differences between light exposure indicated by letters (ANOVA, p < 0.05; n = 5).
Figure 4 presents the values of the oxylipins which significantly varied between the different light conditions, half of which are derived from the EPA. The amount of the six EPA-derived oxylipins and two AA-derivatives which varied under light were all significantly higher under 1000 PAR than in the dark condition (ANOVA, p < 0.05). However, significant differences were also observed between the 1000 PAR and the LL and ML conditions, notably for the 18-epi-18-F3t-IsoP and the 5-epi-5-F3t-IsoP in LL.
Figure 4. Concentration of oxylipins in pg/mg dw which significantly varied in biofilm exposed to dark (D, n = 10) and to irradiances of low (LL: 50 and 100), medium (ML: 250), and high light (HL: 500, 750, and 1000) in µmol photons m−2 s−1 PAR. One-way ANOVA was performed on the data to detect significant differences between treatments, as indicated by letters (p < 0.05; n = 5). Oxylipins were gathered in groups corresponding to their fatty acid precursors AA (arachidonic acid), AdA (adrenic acid), ALA (α-linolenic acid), DHA (docosahexaenoic acid), and EPA (eicosapentaenoic acid).
The oxylipins derived from ALA exhibited variations exclusively within the F1t-series and followed an inverse trend to that observed in EPA metabolites. The amount of ALA-derived oxylipins reached a maximum under 100 PAR, with 47 ± 10 pg/mg dw for 9-epi-9-F1t-PhytoP + ent-16-F1t-PhytoP and a decrease following the increasing irradiance level to reach a minimum value under 1000 PAR with 8 ± 1 pg/mg dw for the ent-16-epi-16-F1t-PhytoP.
The only AdA metabolite measured in this analysis was ent-7 (RS)-7-F2t-dihomo-IsoP. Its amount increased following the increasing irradiances between the dark and 100 PAR conditions as well as between the 500 PAR and 1000 PAR conditions, decreasing between the 100 PAR and 500 PAR conditions. The maximum amount was observed under 100 PAR with 1.5 ± 0.3 pg/mg dw, which was significantly higher than the values observed under the dark, 250, 500, and 750 PAR conditions (ANOVA, p < 0.05). The amounts measured under the 1000 PAR condition were also significantly higher than those found under 500 PAR (ANOVA, p < 0.05).
All the oxylipin amounts that varied significantly under different light conditions were correlated with the level of irradiance, except for ent-7 (RS)-7-F2t-dihomo-IsoP (Table 1). The PhytoPs derived from ALA were negatively correlated with the increase of photon flux; however, the oxylipin amounts derived from EPA and AA were positively correlated with the light increase. The strongest correlation was found for 5-epi-5-F3t-IsoP, which is also the most abundant compound measured.
Table 1. Significant correlation between irradiance levels and amount in oxylipins measured in the biofilm.
The microphytobenthic biofilm encompasses a wide diversity of microorganism taxa, with diatoms overwhelmingly dominant. The isoprostanoids measured in this work depend only on the ROS reaction with the PUFAs and their release as free oxidized lipids by the phospholipases A2 in the cells (Ibrahim et al., 2011; Mallick and Mohn, 2000; Morrow et al., 1992; Roy et al., 2017). The ALA-derived oxylipins were in higher proportion in the dark, LL, and ML conditions than under HL; inversely, the AA- and EPA-derived oxylipins were measured in higher proportions under HL than the other light conditions. These variations in amount and composition under the different irradiances are evidence that light levels affect ROS production in the microphythobentic biofilm, triggering peroxidation on specific PUFAs.
Since FAs are widely recognized as biomarkers (Kelly and Scheibling, 2012; Parrish, 2013), associations with specific biological compartments within the biofilm can be established through the PUFA precursors of light-varying oxylipins. The IsoPs emerged as the predominant varying oxylipins, with EPA, a well-known diatom marker, identified as their precursor (Kelly and Scheibling, 2012; Parrish, 2013). The total of varying EPA-derived oxylipins significantly increased from 750 PAR to 1000 PAR (Figure 3). Their amounts were significantly correlated with increasing irradiance. One of them, 5(R)-5-F3t-IsoP was the most abundant, with values between 100 and 200 pg/mg dw. In the diatom Chaetoceros gracilis, the most abundant isoprostanoids identified were also derived from this PUFA, and 5 (RS)-5-F3t-IsoP accounted for approximately 42% (1.1 μg/g) of the total oxylipins (Vigor et al., 2020). We also observed 8-F3t-IsoP or 8-epi-8-F3t-IsoP to significantly increase in Phaeodactylum tricornutum after 48 h under 0.75 mM of H2O2 (Lupette et al., 2018), but the inverse was found after 24 h under 1 mM of H2O2 for P. tricornutum and C. gracilis (Vigor et al., 2020).
The second group of oxylipins that exhibited significant variation under different irradiances was PhytoPs. These are produced from ALA, which is known to be present in higher concentrations in green algae than in diatoms (D’Souza and Loneragan, 1999; Kelly and Scheibling, 2012). PhytoPs were, however, identified as the primary non-enzymatic oxylipins produced by the P. tricornutum, being measured in the same concentration range as the non-oxidized ALA (around 400 pmol per 1 million cells) (Lupette et al., 2018; Vigor et al., 2020). Therefore, the presence of PhytoPs in the biofilm could also be linked to diatom response. In diatoms, only certain glycerolipids (phosphatidylcholine and diacylglyceryl-hydroxymethyl- N,N,N-trimethyl-β-alanine) present in intracellular endomembranes such as the endoplasmic reticulum contain sufficient amounts of ALA to justify a role for the production of PhytoPs (Leblond et al., 2013; Lupette et al., 2018; Zulu et al., 2018). ALA peroxidation was observed through the variation of F1t-PhytoPs. However, B1t-PhytoP and PhytoFs, also derived from this PUFA, did not vary. In Lupette et al. (2018), the F1t-PhytoPs were also the most abundant series which varied under H2O2 oxidative stress in P. tricornutum, while the ent-16-B1t-PhytoP and 16 (RS)-16 A1-PhytoPs measured in low levels did not vary. Among the specific PUFA peroxidations, the ROS produced under different light conditions may also exhibit preferences for specific reaction sites and pathways.
Interestingly, the increase of oxidation induced by light-triggered ROS production did not appear to affect all PUFA precursors. Specifically, in the case of DHA, the peroxidation products, known as NeuroPs, remained constant across varying irradiances. In related studies, observations of C. gracilis (Vigor et al., 2020) and P. tricornutum indicated that NeuroPs did not vary under H2O2 oxidative stress. Moreover, these compounds were found to be produced in the same concentration range as their non-oxidized precursors (Lupette et al., 2018). Indeed, ROS are constantly produced as byproducts of metabolisms, such as photosynthesis or photorespiration, which continuously lead to lipid peroxidation by healthy organisms (Knieper et al., 2023; Mueller, 2004). This could partly explain this basal presence of the NeuroPs and the other non-varying oxylipins measured in the microphytobenthic biofilm and under non-stressing light levels. This oxidation could also have originated from laboratory conditions, which may have generated additional stress on the biofilm organisms, particularly due to the lack of a photoperiod. However, it is unlikely for MPB that these conditions induced major stress, as the diel migration was not perturbed over a week in the same conditions (Doose and Hubas, 2024).
In the MPB biofilm, the primary metabolism susceptible to generating rapid and substantial variations in ROS production under irradiation changes is photosynthesis, the chloroplast of these microorganisms being a major site of ROS generation (Pitzschke et al., 2006). During photosynthesis, H2O2 is notably generated through the water–water cycle (WWC) (Asada, 1999). In microalgae and cyanobacteria, the WWC is a significant pathway for dissipating excitation energy, accounting for up to 49% of total electron flux in diatoms (Curien et al., 2016; Waring et al., 2010). ROS production under high light can exceed the rate of the WWC reactions, leading to H2O2 increase in the chloroplast. It is challenging to determine whether the concentrations of H2O2 examined in the aforementioned studies are comparable to what photosynthesis might induce under HL conditions in microphytobenthic organisms. This difficulty arises due to uncertainties about the extent of H2O2 entry into the cell through aquaporins (Knieper et al., 2023; Vogelsang and Dietz, 2022) and the possibility that exposure via the culture medium could induce peroxidation at other membrane sites than those associated with H2O2 production in the chloroplast under HL. However, significant accumulation of H2O2 in the diatom N. epithemioides was observed in similar light exposure (30 and 40 min at 1,000 µmol photons m−2 s−1), reaching values of 1–1.5 μmol/μg Chla (Waring et al., 2010). This suggests that the observed increase in AA- and EPA-derived oxylipins in the biofilm under 1000 PAR might be partly attributed to the heightened production of H2O2 through photosynthesis in MPB.
The increase in PhytoPs was observed under non-stressing light, where photosynthesis was presumed to be efficient, considering the Ek and Eopt values in Doose and Hubas (2024) and that ROS scavengers are likely not overwhelmed. The production of these oxylipins triggered under an efficient state of photosynthesis might also suggest that the peroxidation could be attributed to ROS originating from non-photosynthetic organisms. ROS are ubiquitous in marine environments (Diaz et al., 2016; Paul Hansard et al., 2010; Roe et al., 2016; Rose et al., 2008), and bacteria present in natural water are known to contribute to the H2O2 source (Dixon et al., 2013; Marsico et al., 2015; Vermilyea et al., 2010; Zhang et al., 2016) and probably to the IO2⋅− source as well (Diaz et al., 2013; Hansel et al., 2019; Learman et al., 2011; Sutherland et al., 2019; Zhang et al., 2016). However, their concentrations in marine environments range from picomolars to hundreds of nanomolars, which might not be sufficient to trigger all the intracellular peroxidation in diatoms providing the oxylipins amounts measured in the present study (Diaz et al., 2016; Paul Hansard et al., 2010; Roe et al., 2016; Rose et al., 2008; Rusak et al., 2011). The observed prevalence of PhytoPs under non-stressful conditions may suggest a controlled and regulated response by some microphytobenthic organisms, notably diatoms, as discussed above. This could result from peroxidation triggered by a specific ROS signature of photosynthesis in a well-maintained state.
Interestingly, the total amount of oxylipins measured did not vary between all the light conditions except for the 1000 PAR condition. This suggests that the peroxidation rate was the same under these first mentioned irradiances and that the amount of ROS could have been maintained at a steady state by the antioxidant systems of the cells. Indeed, the cell’s capacity to detoxify, scavenge, or buffer ROS is suspected to control the quantity and spatial accumulation of ROS, which can be specific to an intracellular site such as a membrane patch or organelle (Knieper et al., 2023; Mittler et al., 2011). Moreover, the antioxidant controls the termination of the peroxidation reaction (Montuschi et al., 2004), and photoprotectants such as xanthophylls are known to have an effect on oxylipins present (Demmig-adams et al., 2012). Furthermore, a persisting or intensifying light stress triggers an increase of the generated ROS diversity (H2O2, 1O2, 1O2⋅−, etc.) (Foyer, 2018; Waring et al., 2010). The 1O2, characterized by high reactivity, can initiate signals but not transport them. In contrast, the lower reactivity of H2O2 allows it to interact with various biological sites to function as a mobile messenger and to be excreted in the extracellular space (Mullineaux et al., 2018; Schneider et al., 2016). This spatial specificity of ROS and antioxidant presence could thus also explain the differences in the distinct oxylipin responses under the different light levels. Moreover, regarding the localization of the PUFAs precursors in the diatom cell, lipid peroxidation might predominantly occur in endomembranes under LL and ML conditions (Lupette et al., 2018), whereas under HL conditions it might occur in chloroplasts since EPA is known to be a major fatty acid in diatom thylakoïd membranes (Büchel et al., 2022). The increase in IsoPs and PGFs observed under 1000 PAR conditions could thus be triggered by a specific ROS signature produced under conditions of overwhelmed ROS scavengers.
Under 100 PAR, the total amount of oxylipins measured in the biofilm tended to increase and had the highest amount of ALA derivatives, as well as the AdA derivative ent-7 (RS)-7-F2t-dihomo-IsoP. However, regarding the oxylipin proportion presented in Figure 2, the higher and lower percentages for ALA- and EPA-derived oxylipins, respectively, were observed under 250 PAR. Interestingly, the theoretical optimal light level for MPB measured and published by Doose and Hubas (2024) is situated between 100 and 250 PAR (187 ± 22 PAR). It was suggested that diatoms adjust the irradiance they receive around this Ek value through their vertical migration in the sediment (Jesus et al., 2023). Moreover, a downward migration of MPB was induced from 250 PAR (visual observation), corresponding to the range of irradiance found in the literature to induce the downward movement of mudflat MPB (Ezequiel et al., 2015; Laviale et al., 2016; Perkins et al., 2010; Serôdio et al., 2008; 2006). Therefore, the PhytoP synthesis was concomitant with the presence of MPB at the sediment surface, and thus more exposed to oxidative conditions compare to the anoxic conditions in the sediment. However, as discussed previously, the water amounts of ROS are likely not sufficient to explain the entire presence of these isoprostanoids in the biofilm. Regarding the high values of those oxylipins compared to general ALA quantities in diatoms, PhytoPs are suspected to have a biological function (Lupette et al., 2018). Moreover, ALA serves as a primary precursor in plants for various signaling compounds generated through oxidative modification by ROS (Ahme et al., 2020; Schaller and Stintzi, 2009). It would thus be interesting to further investigate the possible implication of non-enzymatic oxylipins such as PhytoPs in the migration response of MPB.
The de-epoxydation rates of the biofilm samples were given by Doose and Hubas (2024). They also increased significantly between 250 and 500 PAR, indicating a high NPQ response. On one hand, NPQ is considered to be the most crucial short-term photoacclimative processes, realized through the xanthophyll cycle in diatoms (Lavaud, 2007; Lavaud et al., 2002). On the other hand, xanthophylls are also known to directly scavenge the triplet-state excitation of Chl a (Larkum, 2003; Müller et al., 2001) and to have strong anti-oxidant properties. This, in turn, prevents lipid peroxidation and thus inhibits additional oxylipin production (Andersson and Aro, 2006; Galinato et al., 2007; Havaux and Niyogi, 1999; Saniewski and Czapski, 1983; Wang and Zheng, 2005). Therefore, it would be interesting to explore whether the epoxidation or de-epoxidation state of the xanthophylls influences isoprostanoid synthesis such the ALA- and EPA-derived oxylipins.
This study is the first investigation of the production of non-enzymatic oxylipins in a microphytobenthic biofilm under different irradiances. It revealed that isoprostanoid levels in the microphytobenthic biofilm respond to varying light intensities. They were identified as originating from the diatoms, indicating a light-dependent influence on ROS production originating from photosynthetic activity. The PUFA precursors showed distinct peroxidation patterns under different light conditions, suggesting a link between light-induced ROS diversity, ROS scavenging efficiency by antioxidant systems, and PUFA oxidation pathways. Under 1000 PAR, the total amount of isoprotanoids increased, indicating an oxidative stress. The EPA and AA derivatives characterized the HL conditions and evidenced lipid peroxidation, probably due to the antioxidant system becoming gradually overwhelmed by the higher generation of ROS through photosynthesis. In contrast, the PhytoPs, ALA derivatives, characterized the LL and ML where the de-epoxidation state was low and ROS scavengers were probably not overwhelmed. This indicates that the lipid peroxidation probably did not occur in the chloroplast but in other cellular sites such as other endomembranes. The concomitant presence of the diatoms at the sediment surface and the PhytoP synthesis suggests that these oxygenic conditions could also partly influence this isoprostanoid production in a photosynthetic independent way. However, the PhytoPs more likely resulted from a regulated response of MPB organisms. This study was conducted in a laboratory, focusing solely on the effects of irradiance variations; the biofilm oxylipin responses might thus differ under more complex environmental conditions. However, these findings provide novel insights into oxylipin production in mudflat biofilms, highlighting interest in exploring their signaling roles related to photoprotective mechanisms and vertical migration.
The raw data supporting the conclusions of this article will be made available by the authors, without undue reservation.
CD: conceptualization, formal analysis, investigation, methodology, writing–original draft, and writing–review and editing. CO: conceptualization, data curation, investigation, methodology, supervision, writing–review and editing, and validation. LM-N: data curation, investigation, methodology, validation, and writing–review and editing. TD: validation and writing–review and editing. CH: conceptualization, funding acquisition, methodology, supervision, validation, and writing–review and editing.
The authors declare that financial support was received for the research, authorship, and/or publication of this article. This study was funded by the Regional Council of French Brittany, the General Council of Finistère.
The authors declare that the research was conducted in the absence of any commercial or financial relationships that could be construed as a potential conflict of interest.
All claims expressed in this article are solely those of the authors and do not necessarily represent those of their affiliated organizations, or those of the publisher, the editors, and the reviewers. Any product that may be evaluated in this article, or claim that may be made by its manufacturer, is not guaranteed or endorsed by the publisher.
The Supplementary Material for this article can be found online at: https://www.frontiersin.org/articles/10.3389/fphbi.2024.1441713/full#supplementary-material
Ahme, O. S., Galano, J. M., Pavlickova, T., Revol-Cavalier, J., Vigor, C., Lee, J. C. Y., et al. (2020). Moving forward with isoprostanes, neuroprostanes and phytoprostanes: where are we now? Essays Biochem. 64, 463–484. doi:10.1042/EBC20190096
Améras, E., Stolz, S., Vollenweider, S., Reymond, P., Mène-Saffrané, L., and Farmer, E. E. (2003). Reactive electrophile species activate defense gene expression in Arabidopsis. Plant J. 34, 205–216. doi:10.1046/J.1365-313X.2003.01718.X
Andersson, B., and Aro, E.-M. (2006). “Photodamage and D1 protein turnover in photosystem II,” in Regulation of photosynthesis (Dordrecht: Springer), 377–393. doi:10.1007/0-306-48148-0_22
Apel, K., and Hirt, H. (2004). Reactive oxygen species: metabolism, oxidative stress, and signal transduction. Annu. Rev. Plant Biol. 55, 373–399. doi:10.1146/annurev.arplant.55.031903.141701
Asada, K. (1999). The water-water cycle in chloroplasts: scavenging of active oxygens and dissipation of excess photons. Annu. Rev. Plant Biol. 50, 601–639. doi:10.1146/annurev.arplant.50.1.601
Barnett, A., Méléder, V., Dupuy, C., and Lavaud, J. (2020). The vertical migratory rhythm of intertidal microphytobenthos in sediment depends on the light photoperiod, intensity, and spectrum: evidence for a positive effect of blue wavelengths. Front. Mar. Sci. 7, 212. doi:10.3389/fmars.2020.00212
Beninger, P. G., and Paterson, D. M. (2018). “Mudflat ecology,” in Springer nature. Editor Ec Aquatic doi:10.1007/978-3-319-99194-8
Büchel, C., Goss, R., Bailleul, B., Campbell, D. A., Lavaud, J., and Lepetit, B. (2022). Photosynthetic light reactions in diatoms. I. The lipids and light-harvesting complexes of the thylakoid membrane. Mol. Life Diatoms, 397–422. doi:10.1007/978-3-030-92499-7_15
Cartaxana, P., Ruivo, M., Hubas, C., Davidson, I., Serôdio, J., and Jesus, B. (2011). Physiological versus behavioral photoprotection in intertidal epipelic and epipsammic benthic diatom communities. J. Exp. Mar. Bio. Ecol. 405, 120–127. doi:10.1016/J.JEMBE.2011.05.027
Consalvey, M., Paterson, D. M., and Underwood, G. J. C. (2004). The ups and downs of life in a benthic biofilm: migration of benthic diatoms. Diatom Res. 19, 181–202. doi:10.1080/0269249X.2004.9705870
Curien, G., Flori, S., Villanova, V., Magneschi, L., Giustini, C., Forti, G., et al. (2016). The water to water cycles in microalgae. Plant Cell. Physiol. 57, 1354–1363. doi:10.1093/PCP/PCW048
Dall’Osto, L., Cazzaniga, S., Havaux, M., and Bassi, R. (2010). Enhanced photoprotection by protein-bound vs free xanthophyll pools: a comparative analysis of chlorophyll b and xanthophyll biosynthesis mutants. Mol. Plant 3, 576–593. doi:10.1093/mp/ssp117
D’Autréaux, B., and Toledano, M. B. (2007). ROS as signalling molecules: mechanisms that generate specificity in ROS homeostasis. Nat. Rev. Mol. Cell. Biol. 8, 813–824. doi:10.1038/nrm2256
Demmig-adams, B., Cohu, C. M., Zadelhoff, G., Veldink, G. A., Muller, O., Iii, W. W. A., et al. (2012). Emerging trade-offs – impact of photoprotectants (PsbS, xanthophylls, and vitamin E) on oxylipins as regulators of development and defense. New Phytol. 197, 720–729. doi:10.1111/nph.12100
Diaz, J. M., Hansel, C. M., Apprill, A., Brighi, C., Zhang, T., Weber, L., et al. (2016). Species-specific control of external superoxide levels by the coral holobiont during a natural bleaching event. Nat. Commun. 7, 13801. doi:10.1038/ncomms13801
Diaz, J. M., Hansel, C. M., Voelker, B. M., Mendes, C. M., Andeer, P. F., and Zhang, T. (2013). Widespread production of extracellular superoxide by heterotrophic bacteria. Sci. (80-. ) 340, 1223–1226. doi:10.1126/science.1237331
Di Dato, V., Barbarinaldi, R., Amato, A., Di Costanzo, F., Fontanarosa, C., Perna, A., et al. (2020a). Variation in prostaglandin metabolism during growth of the diatom Thalassiosira rotula. Sci. Rep. 10, 5374. doi:10.1038/s41598-020-61967-3
Di Dato, V., Di Costanzo, F., Barbarinaldi, R., Perna, A., Ianora, A., and Romano, G. (2019). Unveiling the presence of biosynthetic pathways for bioactive compounds in the Thalassiosira rotula transcriptome. Sci. Rep. 9, 9893. doi:10.1038/s41598-019-46276-8
Di Dato, V., Ianora, A., and Romano, G. (2020b). Identification of prostaglandin pathway in dinoflagellates by transcriptome data mining. Mar. Drugs 18, 109. doi:10.3390/MD18020109
Dietz, K. J. (2008). Redox signal integration: from stimulus to networks and genes. Physiol. Plant. 133, 459–468. doi:10.1111/j.1399-3054.2008.01120.x
Dixon, T. C., Vermilyea, A. W., Scott, D. T., and Voelker, B. M. (2013). Hydrogen peroxide dynamics in an agricultural headwater stream: evidence for significant nonphotochemical production. Limnol. Oceanogr. 58, 2133–2144. doi:10.4319/LO.2013.58.6.2133
Doose, C., and Hubas, C. (2024). The metabolites of light: untargeted metabolomic approaches bring new clues to understand light-driven acclimation of intertidal mudflat biofilm. Sci. Total Environ. 912, 168692. doi:10.1016/j.scitotenv.2023.168692
D’Souza, F. M. L., and Loneragan, N. R. (1999). Effects of monospecific and mixed-algae diets on survival, development and fatty acid composition of penaeid prawn (Penaeus spp.) larvae. Mar. Biol. 133, 621–633. doi:10.1007/s002270050502
Dumanović, J., Nepovimova, E., Natić, M., Kuča, K., and Jaćević, V. (2021). The significance of reactive oxygen species and antioxidant defense system in plants: a concise overview. Front. Plant Sci. 11, 1–13. doi:10.3389/fpls.2020.552969
Ezequiel, J., Laviale, M., Frankenbach, S., Cartaxana, P., and Serôdio, J. (2015). Photoacclimation state determines the photobehaviour of motile microalgae: the case of a benthic diatom. J. Exp. Mar. Bio. Ecol. 468, 11–20. doi:10.1016/j.jembe.2015.03.004
Ezequiel, J., Nitschke, M. R., Laviale, M., Serôdio, J., and Frommlet, J. C. (2023). Concurrent bioimaging of microalgal photophysiology and oxidative stress. Photosynth. Res. 155, 177–190. doi:10.1007/s11120-022-00989-6
Foyer, C. H. (2018). Reactive oxygen species, oxidative signaling and the regulation of photosynthesis. Environ. Exp. Bot. 154, 134–142. doi:10.1016/J.ENVEXPBOT.2018.05.003
Foyer, C. H., Ruban, A. V., and Noctor, G. (2017). Viewing oxidative stress through the lens of oxidative signalling rather than damage. Biochem. J. 474, 877–883. doi:10.1042/BCJ20160814
Galano, J. M., Lee, Y. Y., Oger, C., Vigor, C., Vercauteren, J., Durand, T., et al. (2017). Isoprostanes, neuroprostanes and phytoprostanes: an overview of 25 years of research in chemistry and biology. Prog. Lipid Res. 68, 83–108. doi:10.1016/j.plipres.2017.09.004
Galinato, M. G. I., Niedzwiedzki, D., Deal, C., Birge, R. R., and Frank, H. A. (2007). Cation radicals of xanthophylls. Photosynth. Res. 94, 67–78. doi:10.1007/s11120-007-9218-5
Gerwick, W. H., Moghaddam, M., and Hamberg, M. (1991). Oxylipin metabolism in the red alga Gracilariopsis lemaneiformis: mechanism of formation of vicinal dihydroxy fatty acids. Arch. Biochem. Biophys. 290, 436–444. doi:10.1016/0003-9861(91)90563-X
Guy, A., Oger, C., Heppekausen, J., Signorini, C., Defelice, C., Fürstner, A., et al. (2014). Oxygenated Metabolites of n-3 polyunsaturated fatty acids as potential oxidative stress biomarkers: total synthesis of 8-F3t-IsoP, 10-F 4t-NeuroP and [D4]-10-F4t-NeuroP. Chem. - A Eur. J. 20, 6374–6380. doi:10.1002/chem.201400380
Hansel, C. M., Diaz, J. M., and Plummer, S. (2019). Tight regulation of extracellular superoxide points to its vital role in the physiology of the globally relevant Roseobacter clade. MBio 10, 026688-e2718. doi:10.1128/mBio.02668-18
Haubois, A. G., Sylvestre, F., Guarini, J. M., Richard, P., and Blanchard, G. F. (2005). Spatio-temporal structure of the epipelic diatom assemblage from an intertidal mudflat in Marennes-Oléron Bay, France. Fr. Estuar. Coast. Shelf Sci. 64, 385–394. doi:10.1016/j.ecss.2005.03.004
Havaux, M., and Niyogi, K. K. (1999). The violaxanthin cycle protects plants from photooxidative damage by more than one mechanism. Proc. Natl. Acad. Sci. U. S. A. 96, 8762–8767. doi:10.1073/PNAS.96.15.8762
Hope, J. A., Paterson, D. M., Thrush, S. F., Julie Hope, C. A., Editor, H., and Van Alstyne, K. (2020). The role of microphytobenthos in soft-sediment ecological networks and their contribution to the delivery of multiple ecosystem services. J. Ecol. 108, 815–830. doi:10.1111/1365-2745.13322
Ibrahim, A., Mbodji, K., Hassan, A., Aziz, M., Boukhettala, N., Coëffier, M., et al. (2011). Anti-inflammatory and anti-angiogenic effect of long chain n-3 polyunsaturated fatty acids in intestinal microvascular endothelium. Clin. Nutr. 30, 678–687. doi:10.1016/J.CLNU.2011.05.002
Jahn, U., Galano, J. M., and Durand, T. (2008). Beyond prostaglandins - chemistry and biology of cyclic oxygenated metabolites formed by free-radical pathways from polyunsaturated fatty acids. Angew. Chem. Int. Ed. 47, 5894–5955. doi:10.1002/anie.200705122
Jesus, B., Jauffrais, T., Trampe, E., Méléder, V., Ribeiro, L., Bernhard, J. M., et al. (2023). Microscale imaging sheds light on species-specific strategies for photo-regulation and photo-acclimation of microphytobenthic diatoms. Environ. Microbiol. 16499, 3087–3103. doi:10.1111/1462-2920.16499
Jesus, B., Perkins, R. G., Consalvey, M., Brotas, V., and Paterson, D. M. (2006). Effects of vertical migrations by benthic microalgae on fluorescence measurements of photophysiology. Mar. Ecol. Prog. Ser. 315, 55–66. doi:10.3354/meps315055
Kelly, J. R., and Scheibling, R. E. (2012). Fatty acids as dietary tracers in benthic food webs. Mar. Ecol. Prog. Ser. 446, 1–22. doi:10.3354/meps09559
Knieper, M., Viehhauser, A., and Dietz, K.-J. (2023). Oxylipins and reactive carbonyls as regulators of the plant redox and reactive oxygen species network under stress. Antioxidants 12, 814–829. doi:10.3390/antiox12040814
Krieger-Liszkay, A. (2005). Singlet oxygen production in photosynthesis. J. Exp. Bot. 56, 337–346. doi:10.1093/JXB/ERH237
Krieger-Liszkay, A., Christian, A. E., Ae, F., and Trebst, A. (2008). Singlet oxygen production in photosystem II and related protection mechanism. Photosynth. Res. 98, 551–564. doi:10.1007/s11120-008-9349-3
Lamari, N., Ruggiero, M. V., D’Ippolito, G., Kooistra, W. H. C. F., Fontana, A., and Montresor, M. (2013). Specificity of lipoxygenase pathways supports species delineation in the marine diatom genus Pseudo-nitzschia. PLoS One 8, 732811–e73310. doi:10.1371/JOURNAL.PONE.0073281
Larkum, A. W. D. (2003). “Light-harvesting systems in algae,” in Photosynthesis in algae. Editors W. Larkum, S. Douglas, and J. Raven (Kluwer Academic Publishers), 277–304. doi:10.1007/978-94-007-1038-2_13
Lavaud, J. (2007). Fast regulation of photosynthesis in diatoms: mechanisms, evolution and ecophysiology.
Lavaud, J., and Goss, R. (2014). “The peculiar features of non-photochemical fluorescence quenching in diatoms and brown algae,” in Non-photochemical quenching and energy dissipation in plants, algae and cyanobacteria. Editors B. Demmig-Adams, G. Garab, and W. Adams III (Springer Science + Business Media Dordrecht), 421–443. doi:10.1007/978-94-017-9032-1_20
Lavaud, J., Rousseau, B., Van Gorkom, H. J., and Etienne, A. L. (2002). Influence of the diadinoxanthin pool size on photoprotection in the marine planktonic diatom Phaeodactylum tricornutum. Plant Physiol. 129, 1398–1406. doi:10.1104/PP.002014
Laviale, M., Frankenbach, S., and Serôdio, J. (2016). The importance of being fast: comparative kinetics of vertical migration and non-photochemical quenching of benthic diatoms under light stress. Mar. Biol. 163, 10–12. doi:10.1007/s00227-015-2793-7
Learman, D. R., Voelker, B. M., Vazquez-Rodriguez, A. I., and Hansel, C. M. (2011). Formation of manganese oxides by bacterially generated superoxide. Nat. Geosci. 4, 95–98. doi:10.1038/NGEO1055
Leblond, J. D., Dahmen, A. S., Dodson, V. J., and Dahmen, J. L. (2013). Characterization of the betaine lipids, diacylglyceryl-N,N,N-trimethylhomoserine (DGTS) and diaclyglycerylhydroxymethyl-N,N,N-trimethyl-β-alanine (DGTA), in brown- and green-pigmented raphidophytes. Arch. Hydrobiol. Suppl. Algol. Stud. 142, 17–27. doi:10.1127/1864-1318/2013/0130
Lebreton, B., Rivaud, A., Picot, L., Prévost, B., Barillé, L., Sauzeau, T., et al. (2019). From ecological relevance of the ecosystem services concept to its socio-political use. The case study of intertidal bare mudflats in the Marennes-Oléron Bay, France. Ocean. Coast. Manag. 172, 41–54. doi:10.1016/J.OCECOAMAN.2019.01.024
Lepetit, B., Sturm, S., Rogato, A., Gruber, A., Sachse, M., Falciatore, A., et al. (2013). High light acclimation in the secondary plastids containing diatom Phaeodactylum tricornutum is triggered by the redox state of the plastoquinone pool. Plant Physiol. 161, 853–865. doi:10.1104/PP.112.207811
Linares-Maurizi, A., Reversat, G., Awad, R., Bultel-Poncé, V., Oger, C., Galano, J. M., et al. (2023). Bioactive oxylipins profile in marine microalgae. Mar. Drugs 21, 136. doi:10.3390/md21030136
Longini, M., Belvisi, E., Proietti, F., Bazzini, F., Buonocore, G., and Perrone, S. (2017). Oxidative stress biomarkers: establishment of reference values for isoprostanes, AOPP, and NPBI in cord blood. Mediat. Inflamm. 2017, 1758432. doi:10.1155/2017/1758432
Lupette, J., Jaussaud, A., Vigor, C., Oger, C., Galano, J. M., Réversat, G., et al. (2018). Non-enzymatic synthesis of bioactive isoprostanoids in the diatom Phaeodactylum following oxidative stress. Plant Physiol. 178, 1344–1357. doi:10.1104/PP.18.00925
Macintyre, H. L., Geider, R. J., and Miller, D. C. (1996). Microphytobenthos: the ecological role of the secret garden of unvegetated, shallow-water marine habitats. II. Role in sediment stability and shallow-water food webs. Estuaries 19, 202–212. doi:10.2307/1352225
Mallick, N., and Mohn, F. H. (2000). Reactive oxygen species: response of algal cells. J. Plant Physiol. 157, 183–193. doi:10.1016/S0176-1617(00)80189-3
Marques da Silva, J., Cruz, S., and Cartaxana, P. (2017). Inorganic carbon availability in benthic diatom communities: photosynthesis and migration. Philos. Trans. R. Soc. B Biol. Sci. 372, 20160398. doi:10.1098/RSTB.2016.0398
Marsico, R. M., Schneider, R. J., Voelker, B. M., Zhang, T., Diaz, J. M., Hansel, C. M., et al. (2015). Spatial and temporal variability of widespread dark production and decay of hydrogen peroxide in freshwater. Aquat. Sci. 77, 523–533. doi:10.1007/s00027-015-0399-2
Méléder, V., Rincé, Y., Barillé, L., Gaudin, P., and Rosa, P. (2007). Spatiotemporal changes in microphytobenthos assemblages in a macrotidal flat (Bourgneuf Bay, France). J. Phycol. 43, 1177–1190. doi:10.1111/j.1529-8817.2007.00423.x
Meyer, A. J. (2008). The integration of glutathione homeostasis and redox signaling. J. Plant Physiol. 165, 1390–1403. doi:10.1016/j.jplph.2007.10.015
Meyer, N., Rettner, J., Werner, M., Werz, O., and Pohnert, G. (2018). Algal oxylipins mediate the resistance of diatoms against algicidal bacteria. Mar. Drugs 16, 486. doi:10.3390/MD16120486
Mittler, R., Vanderauwera, S., Suzuki, N., Miller, G., Tognetti, V. B., Vandepoele, K., et al. (2011). ROS signaling: the new wave? Trends Plant Sci. 16, 300–309. doi:10.1016/j.tplants.2011.03.007
Montuschi, P., Barnes, P. J., and Jackson Roberts Ii, L. (2004). Isoprostanes: markers and mediators of oxidative stress. FASEB J. 18, 1791–1800. doi:10.1096/FJ.04-2330REV
Morrow, J. D., Awad, J. A., Boss, H. J., Blair, I. A., and Roberts, L. J. (1992). Non-cyclooxygenase-derived prostanoids (F2-isoprostanes) are formed in situ on phospholipids. Proc. Natl. Acad. Sci. U. S. A. 89, 10721–10725. doi:10.1073/PNAS.89.22.10721
Morrow, J. D., Hill, K. E., Burk, R. F., Nammour, T. M., Badr, K. F., and Roberts, L. J. (1990). A series of prostaglandin F2-like compounds are produced in vivo in humans by a non-cyclooxygenase, free radical-catalyzed mechanism. Proc. Natl. Acad. Sci. U. S. A. 87, 9383–9387. doi:10.1073/PNAS.87.23.9383
Mueller, M. J. (2004). Archetype signals in plants: the phytoprostanes. Curr. Opin. Plant Biol. 7, 441–448. doi:10.1016/j.pbi.2004.04.001
Müller, P., Li, X. P., and Niyogi, K. K. (2001). Non-photochemical quenching. A response to excess light energy. Plant Physiol. 125, 1558–1566. doi:10.1104/PP.125.4.1558
Mullineaux, P. M., Exposito-Rodriguez, M., Laissue, P. P., and Smirnoff, N. (2018). ROS-dependent signalling pathways in plants and algae exposed to high light: comparisons with other eukaryotes. Free Radic. Biol. Med. 122, 52–64. doi:10.1016/j.freeradbiomed.2018.01.033
Nishiyama, Y., Allakhverdiev, S. I., and Murata, N. (2006). A new paradigm for the action of reactive oxygen species in the photoinhibition of photosystem II. Biochim. Biophys. Acta - Bioenerg. 1757, 742–749. doi:10.1016/j.bbabio.2006.05.013
Noctor, G., and Foyer, C. H. (2016). Intracellular redox compartmentation and ROS-related communication in regulation and signaling. Plant Physiol. 171, 1581–1592. doi:10.1104/PP.16.00346
Nourooz-Zadeh, J., Liu, E. H. C., Änggård, E. E., and Halliwell, B. (1998). F4-isoprostanes: a novel class of prostanoids formed during peroxidation of docosahexaenoic acid (DHA). Biochem. Biophys. Res. Commun. 242, 338–344. doi:10.1006/bbrc.1997.7883
Nymark, M., Valle, K. C., Brembu, T., Hancke, K., Winge, P., Andresen, K., et al. (2009). An integrated analysis of molecular acclimation to high light in the marine diatom Phaeodactylum tricornutum. PLoS One 4, 7743. doi:10.1371/journal.pone.0007743
Orefice, I., Di Dato, V., Sardo, A., Lauritano, C., and Romano, G. (2022). Lipid mediators in marine diatoms. Aquat. Ecol. 56, 377–397. doi:10.1007/s10452-021-09932-8
Parchmann, S., and Mueller, M. J. (1998). Evidence for the formation of dinor isoprostanes E1 from alpha-linolenic acid in plants. J. Biol. Chem. 273, 32650–32655. doi:10.1074/JBC.273.49.32650
Parrish, C. C. (2013). Lipids in marine ecosystems. ISRN Oceanogr. 2013, 1–16. doi:10.5402/2013/604045
Paul Hansard, S., Vermilyea, A. W., and Voelker, B. M. (2010). Measurements of superoxide radical concentration and decay kinetics in the Gulf of Alaska. Deep. Res. Part I Oceanogr. Res. Pap. 57, 1111–1119. doi:10.1016/j.dsr.2010.05.007
Perkins, R. G., Lavaud, J., Serôdio, J., Mouget, J. L., Cartaxana, P., Rosa, P., et al. (2010). Vertical cell movement is a primary response of intertidal benthic biofilms to increasing light dose. Mar. Ecol. Prog. Ser. 416, 93–103. doi:10.3354/meps08787
Pitzschke, A., Forzani, C., and Hirt, H. (2006). Reactive oxygen species signaling in plants. Antioxid. Redox Signal. 8, 1757–1764. doi:10.1089/ARS.2006.8.1757
Prelle, L. R., and Karsten, U. (2022). Photosynthesis, respiration, and growth of five benthic diatom strains as a function of intermixing processes of coastal peatlands with the baltic sea. Microorganisms 10, 749. doi:10.3390/microorganisms10040749
Prins, A., Deleris, P., Hubas, C., and Jesus, B. (2020). Effect of light intensity and light quality on diatom behavioral and physiological photoprotection. Front. Mar. Sci. 7, 203. doi:10.3389/fmars.2020.00203
Ramel, F., Birtic, S., Ginies, C., Soubigou-Taconnat, L., Triantaphylidès, C., and Havaux, M. (2012). Carotenoid oxidation products are stress signals that mediate gene responses to singlet oxygen in plants. Proc. Natl. Acad. Sci. U. S. A. 109, 5535–5540. doi:10.1073/pnas.1115982109
Ribeiro, L., Brotas, V., Rincé, Y., and Jesus, B. (2013). Structure and diversity of intertidal benthic diatom assemblages in contrasting shores: a case study from the Tagus estuary. J. Phycol. 49, 258–270. doi:10.1111/jpy.12031
Roberts, L. J., Montine, T. J., Markesbery, W. R., Tapper, A. R., Hardy, P., Chemtob, S., et al. (1998). Formation of isoprostane-like compounds (neuroprostanes) in vivo from docosahexaenoic acid. J. Biol. Chem. 273, 13605–13612. doi:10.1074/JBC.273.22.13605
Roe, K. L., Schneider, R. J., Hansel, C. M., and Voelker, B. M. (2016). Measurement of dark, particle-generated superoxide and hydrogen peroxide production and decay in the subtropical and temperate North Pacific Ocean. Deep Sea Res. Part I Oceanogr. Res. Pap. 107, 59–69. doi:10.1016/J.DSR.2015.10.012
Rose, A. L., Webb, E. A., Waite, T. D., and Moffett, J. W. (2008). Measurement and implications of nonphotochemically generated superoxide in the equatorial pacific ocean. Environ. Sci. Technol. 42, 2387–2393. doi:10.1021/es7024609
Roy, J., Galano, J. M., Durand, T., Le Guennec, J. Y., and Lee, J. C. Y. (2017). Physiological role of reactive oxygen species as promoters of natural defenses. FASEB J. 31, 3729–3745. doi:10.1096/FJ.201700170R
Ruocco, N., Albarano, L., Esposito, R., Zupo, V., Costantini, M., and Ianora, A. (2020). Multiple roles of diatom-derived oxylipins within marine environments and their potential biotechnological applications. Mar. Drugs 18, 342. doi:10.3390/md18070342
Rusak, S. A., Peake, B. M., Richard, L. E., Nodder, S. D., and Cooper, W. J. (2011). Distributions of hydrogen peroxide and superoxide in seawater east of New Zealand. Mar. Chem. 127, 155–169. doi:10.1016/j.marchem.2011.08.005
Russo, E., d’Ippolito, G., Fontana, A., Sarno, D., D’Alelio, D., Busseni, G., et al. (2020). Density-dependent oxylipin production in natural diatom communities: possible implications for plankton dynamics. ISME J. 14, 164–177. doi:10.1038/S41396-019-0518-5
Saniewski, M., and Czapski, J. (1983). The effect of methyl jasmonate on lycopene and β-carotene accumulation in ripening red tomatoes. Experientia 39, 1373–1374. doi:10.1007/bf01990110
Schaller, A., and Stintzi, A. (2009). Enzymes in jasmonate biosynthesis - structure, function, regulation. Phytochemistry 70, 1532–1538. doi:10.1016/J.PHYTOCHEM.2009.07.032
Schneider, R. J., Roe, K. L., Hansel, C. M., and Voelker, B. M. (2016). Species-level variability in extracellular production rates of reactive oxygen species by diatoms. Front. Chem. 4, 5. doi:10.3389/fchem.2016.00005
Serôdio, J., Coelho, H., Vieira, S., and Cruz, S. (2006). Microphytobenthos vertical migratory photoresponse as characterised by light-response curves of surface biomass. Estuar. Coast. Shelf Sci. 68, 547–556. doi:10.1016/j.ecss.2006.03.005
Serôdio, J., Ezequiel, J., Barnett, A., Mouget, J. L., Meĺéder, V., Laviale, M., et al. (2012). Efficiency of photoprotection in microphytobenthos: role of vertical migration and the xanthophyll cycle against photoinhibition. Aquat. Microb. Ecol. 67, 161–175. doi:10.3354/AME01591
Serôdio, J., Vieira, S., and Cruz, S. (2008). Photosynthetic activity, photoprotection and photoinhibition in intertidal microphytobenthos as studied in situ using variable chlorophyll fluorescence. Cont. Shelf Res. 28, 1363–1375. doi:10.1016/j.csr.2008.03.019
Sutherland, K. M., Coe, A., Gast, R. J., Plummer, S., Suffridge, C. P., Diaz, J. M., et al. (2019). Extracellular superoxide production by key microbes in the global ocean. Limnol. Oceanogr. 64, 2679–2693. doi:10.1002/lno.11247
Triantaphylidès, C., and Havaux, M. (2009). Singlet oxygen in plants: production, detoxification and signaling. Trends Plant Sci. 14, 219–228. doi:10.1016/J.TPLANTS.2009.01.008
Triantaphylidès, C., Krischke, M., Hoeberichts, F. A., Ksas, B., Gresser, G., Havaux, M., et al. (2008). Singlet oxygen is the major reactive oxygen species involved in photooxidative damage to plants. Plant Physiol. 148, 960–968. doi:10.1104/PP.108.125690
Underwood, G. J. C., and Kromkamp, J. (1999). Primary production by phytoplankton and microphytobenthos in estuaries. Adv. Ecol. Res. 29, 93–153. doi:10.1016/S0065-2504(08)60192-0
Vermilyea, A. W., Hansard, S. P., and Voelker, B. M. (2010). Dark production of hydrogen peroxide in the Gulf of Alaska. Limnol. Oceanogr. 55, 580–588. doi:10.4319/lo.2009.55.2.0580
Vigor, C., Oger, C., Reversat, G., Rocher, A., Zhou, B., Linares-Maurizi, A., et al. (2020). Isoprostanoid profiling of marine microalgae. Biomolecules 10, 1073. doi:10.3390/BIOM10071073
Vigor, C., Reversat, G., Rocher, A., Oger, C., Vercauteren, J., Durand, T., et al. (2018). Isoprostanoids quantitative profiling of marine red and brown macroalgae. Food Chem. 268, 452–462. doi:10.1016/j.foodchem.2018.06.111
Vogelsang, L., and Dietz, K. J. (2022). Plant thiol peroxidases as redox sensors and signal transducers in abiotic stress acclimation. Free Radic. Biol. Med. 193, 764–778. doi:10.1016/J.FREERADBIOMED.2022.11.019
Wang, S. Y., and Zheng, W. (2005). Preharvest application of methyl jasmonate increases fruit quality and antioxidant capacity in raspberries. Int. J. Food Sci. Technol. 40, 187–195. doi:10.1111/J.1365-2621.2004.00930.X
Waring, J., Klenell, M., Bechtold, U., Underwood, G. J. C., and Baker, N. R. (2010). Light-induced responses of oxygen photoreduction, reactive oxygen species production and scavenging in two diatom species. J. Phycol. 46, 1206–1217. doi:10.1111/j.1529-8817.2010.00919.x
Werner, U., Billerbeck, M., Polerecky, L., Franke, U., Huettel, M., Van Beusekom, J. E. E., et al. (2006). Spatial and temporal patterns of mineralization rates and oxygen distribution in a permeable intertidal sand flat (Sylt, Germany). Limnol. Oceanogr. 51, 2549–2563. doi:10.4319/lo.2006.51.6.2549
Woelfel, J., Schoknecht, A., Schaub, I., Enke, N., Schumann, R., and Karsten, U. (2014). Growth and photosynthesis characteristics of three benthic diatoms from the brackish southern Baltic Sea in relation to varying environmental conditions. Phycologia 53, 639–651. doi:10.2216/14-019.1
Zhang, T., Diaz, J. M., Brighi, C., Parsons, R. J., McNally, S., Apprill, A., et al. (2016). Dark production of extracellular superoxide by the coral Porites astreoides and representative symbionts. Front. Mar. Sci. 3, 232. doi:10.3389/fmars.2016.00232
Keywords: microphytobenthos, oxylipins, isprostanoids, light acclimations, mudflat biofilm
Citation: Doose C, Oger C, Mas-Normand L, Durand T and Hubas C (2024) Non-enzymatic oxylipin production in a mudflat microphytobenthic biofilm: evidence of a diatom response to light. Front. Photobiol. 2:1441713. doi: 10.3389/fphbi.2024.1441713
Received: 31 May 2024; Accepted: 16 August 2024;
Published: 09 September 2024.
Edited by:
Herwig Stibor, Ludwig Maximilian University of Munich, GermanyReviewed by:
Paulo Cartaxana, University of Aveiro, PortugalCopyright © 2024 Doose, Oger, Mas-Normand, Durand and Hubas. This is an open-access article distributed under the terms of the Creative Commons Attribution License (CC BY). The use, distribution or reproduction in other forums is permitted, provided the original author(s) and the copyright owner(s) are credited and that the original publication in this journal is cited, in accordance with accepted academic practice. No use, distribution or reproduction is permitted which does not comply with these terms.
*Correspondence: Caroline Doose, Y2Fyb2xpbmUuZG9vc2VAdW5pdi1icmVzdC5mcg==
Disclaimer: All claims expressed in this article are solely those of the authors and do not necessarily represent those of their affiliated organizations, or those of the publisher, the editors and the reviewers. Any product that may be evaluated in this article or claim that may be made by its manufacturer is not guaranteed or endorsed by the publisher.
Research integrity at Frontiers
Learn more about the work of our research integrity team to safeguard the quality of each article we publish.