- 1Department of Dermatology, Miguel Servet University Hospital, Zaragoza, Spain
- 2Department of Veterinary Medicine, Faculty of Biomedical and Health Sciences, Universidad Europea de Madrid, Villaviciosa de Odón, Spain
- 3Department of Dermatology, University of Zaragoza, Zaragoza, Spain
Introduction: Light-based antimicrobial photodynamic therapy (aPDT) shows promise against a wide range of microorganisms, including drug-resistant strains, with low resistance development likelihood. However, its limitations include the need for light exposure, suitability for local infections, and limited industry interest. Relevant studies on aPDT for various infections are discussed.
Methods: We conducted an extensive literature review on aPDT encompassing articles published from the year 2000 to the current date. To compile comprehensive data, multiple databases (PubMed, Web of Science, Scopus, and Cochrane Library) were systematically searched for relevant studies in both English and Spanish languages. The search strategy involved a combination of Medical Subject Headings (MeSH) terms and free-text terms. The terms included variations and combinations of “Antimicrobial Photodynamic Therapy,” “aPDT,” “photodynamic inactivation,” “microbial photoinactivation,” among others. To ensure the reliability and quality of the information gathered, articles were screened based on relevance to aPDT in antimicrobial therapy, methodological rigor, and alignment with the objectives of this review.
Results: aPDT shows promise in treating various cutaneous infections. It effectively targets fungal infections, including caused by dermatophytes and Malassezia spp., with notable results against dermatophytosis and tinea capitis. New photosensitizers, like nanoencapsulated hypericin, hold potential for treating these infections. In onychomycosis, aminolevulinic acid-aPDT or methylene blue-aPDT offers an alternative to conventional therapies, showing cure rates as high as 90%. For bacterial infections, it presents a solution against antibiotic-resistant strains and infected ulcers, with potential benefits for wound healing. Regarding viral infections, it indirectly targets viruses by impacting host cells’ metabolism, showing efficacy against human papillomavirus and herpes viruses. Lastly, aPDT demonstrates success in treating parasitic infections, particularly leishmaniasis, with cure rates of up to 100%.
Discussion: aPDT is increasingly used in dermatological infections, showing efficacy against various microorganisms and stimulating wound healing. It can combat resistant strains and mixed infections, especially in chronic wounds. Combining photodynamic therapy with antimicrobials is a promising strategy to address antimicrobial resistance, but there are practical limitations. Further research is needed for home-based protocols, portable devices, and adapting photosensitizers, as aPDT holds potential as an alternative treatment for recalcitrant infections and immunosuppressed patients, though challenges remain in application.
1 Introduction
The alarming surge in microbial drug resistance has become a global health crisis, exacerbated by the extensive use of antibiotics during the COVID-19 pandemic to combat bacterial coinfections and secondary infections (Sellera et al., 2022; Sabino et al., 2023). Bacteria’s rapid acquisition of resistance genes across generations and strains poses a dire threat. The potential postantibiotic era could cripple modern medicine, rendering common infections and critical medical procedures, like transplants and oncology treatments, untreatable. While antibiotic resistance predominantly affects bacteria, emerging threats like drug-resistant fungi, exemplified by Candida auris, demand equal vigilance (Eix and Nett, 2022).
Light-based technologies like antimicrobial photodynamic therapy (aPDT) have a historical precedent, from Niels Finsen’s Nobel Prize-winning work against cutaneous tuberculosis to contemporary applications (Dai et al., 2009; Sellera et al., 2022; Sabino et al., 2023). aPDT, leveraging light and photosensitizing drugs (PS), induces cell death through oxidative stress and demonstrates efficacy against a wide spectrum of microorganisms, including bacteria, fungi, parasites, and viruses, exhibiting efficacy against representative members of each group and including drug-resistant strains (Wainwright et al., 2017; Pérez-Laguna et al., 2018a). A crucial aspect of the oxidative and nitrosative stress generated by this process for antimicrobial applications lies in the fact that the various cellular targets of these radicals minimize the likelihood of resistant strain selection. Radicals produced through aPDT possess exceedingly brief half-lives and exclusively react at their sites of origin, mitigating toxicity to adjacent normal tissues (Baltazar et al., 2015). Unlike traditional antibiotics, aPDT’s diverse cellular targets make resistance development less likely (Dai et al., 2009). Encouragingly, aPDT even diminishes bacterial virulence and enhances antibiotic sensitivity. This makes aPDT a promising clinical option, prompting the question of whether it's time for aPDT to transition from the lab to clinical practice, particularly as the world confronts simultaneous health crises (Pérez-Laguna et al., 2019; Sellera et al., 2022).
The antimicrobial efficacy of aPDT has been extensively explored through numerous in vitro studies since the 1990s. Many of these investigations showcased promising outcomes, frequently achieving inactivation rates exceeding 5 log10 colony-forming units (CFU) (Cieplik et al., 2018; Pérez-Laguna et al., 2021). However, it’s noteworthy that a significant portion of these studies have been conducted on planktonic cultures, namely free-living bacterial cells. Yet, to comprehensively assess antimicrobial efficacy, examining its impact on biofilms is imperative. Biofilms are known to exhibit vastly distinct properties, including a tolerance up to 1000 times greater to antimicrobial therapies. Additionally, the biofilm matrix (extracellular polymeric substance) can shield bacteria against aPDT-induced demise by impeding PS penetration throughout the biofilm and hampering light propagation in deeper layers (Marsh et al., 2011; Pérez-Laguna et al., 2018b; Pérez-Laguna et al., 2021).
aPDT has been used against all type of microorganisms in vitro, however, considering the ease use of the aPDT lamps on the skin and the application of the PS, mucocutaneous infections have been where it has been used the most (Dai et al., 2009). In addition, the beneficial effects of aPDT on host tissues, such as growth factor stimulation and immune response enhancement, potentially fostering improved wound healing, support its use for chronic ulcers (Cieplik et al., 2018; Pérez et al., 2021).
Nevertheless, there are some drawbacks that make that aPDT is not still a routine treatment used in the clinics: 1) its effect lasts only during the light exposures, therefore those germs that survive can regrowth and maintain the infection; 2) considering the need of light to active the PS, the treatment is only useful for local infections; 3) treatment is done by doctors and nurses at hospital, therefore it is time consuming for them; and 4) even though there is a lot of basic research on aPDT, there is not significant industry interest (Dai et al., 2009; Pérez-Laguna et al., 2019; Sellera et al., 2022).
2 Components and mechanism of aPDT
aPDT relies on the utilization of various molecules known as PS with varying chemical complexity. When combined with visible light or near-infrared light and molecular oxygen (O2), these PS can eliminate target microorganisms. The simplified mechanism by which the three essential components of aPDT (light, PS, and O2) interact is as follows: A light source is required to activate the PS. This involves matching the emission wavelength (λ) of the light with the absorption λ of the PS, causing the PS to transition from its ground state to a temporary singlet state (1PS*), ultimately reaching an excited triplet state (3PS*). When the PS returns to its ground state, it releases energy, which then reacts with O2 to generate reactive oxygen species (ROS). These ROS are responsible for causing damage to the target microorganism. If the ROS produced includes superoxide anion, hydrogen peroxide, or hydroxyl radical, the process is referred to as type I. If singlet oxygen is generated, it is termed type II aPDT (Wainwright et al., 2017; Pérez-Laguna et al., 2018a; Polat and Kang, 2021).
2.1 Photosensitizers
PS, which are molecules capable of triggering a photodynamic reaction when exposed to light of specific λ, can be classified into porphyrinoids and non-porphyrinoids. Table 1 includes the most prominent examples detailing their origin and absorption spectrum (Pérez-Laguna et al., 2018a).
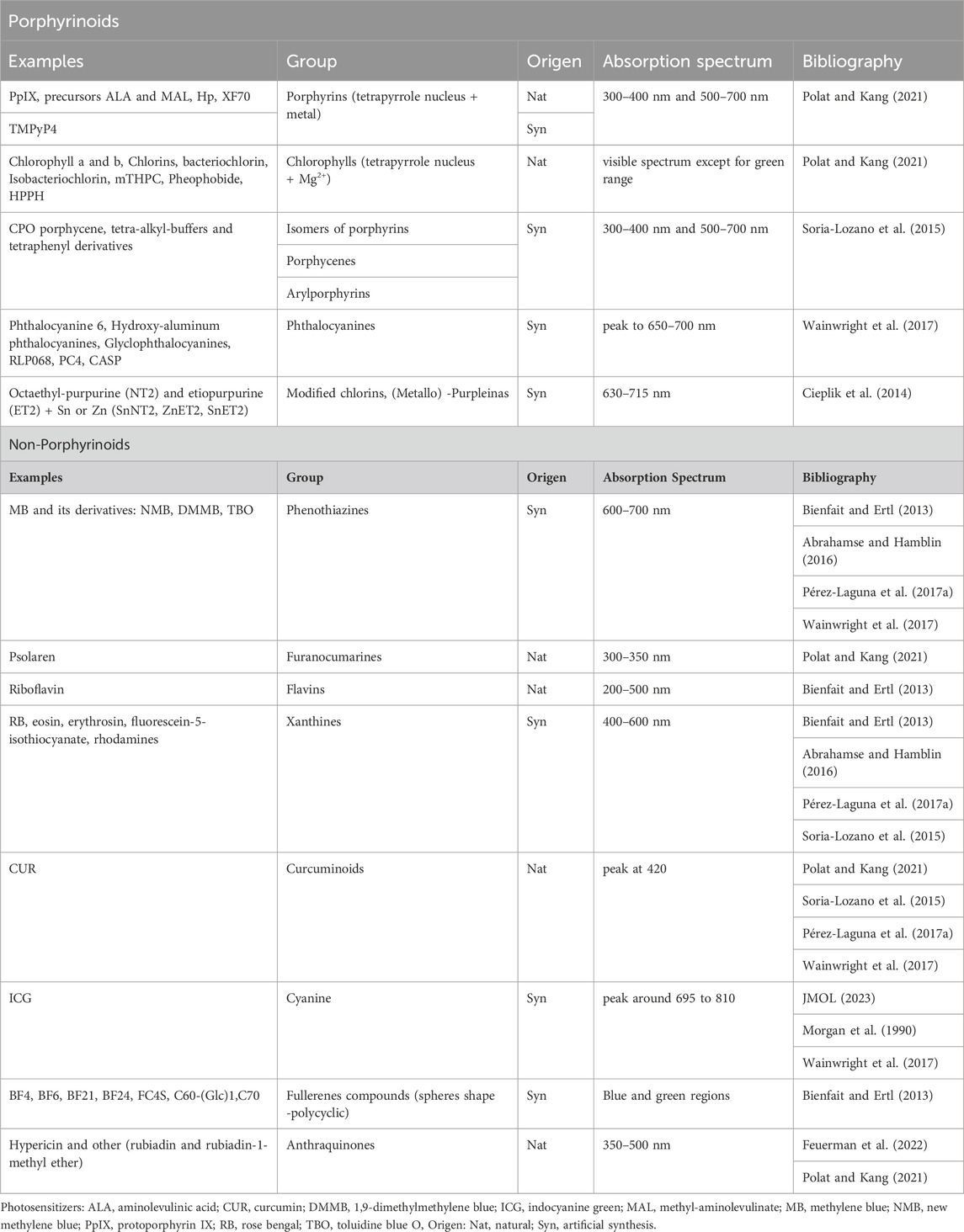
Table 1. Classification of the main PS for aPDT (Adapted from Pérez-Laguna et al., 2018a).
The most common PS employed in aPDT include the phenothiazines toluidine blue O (TBO) and methylene blue (MB), as well as porphyrins, with a notable emphasis on aminolevulinic acid (ALA) and its derivative, methyl-aminolevulinate (MAL). Both ALA and MAL serve as precursors for the production of protoporphyrin IX (PpIX) (Wainwright et al., 2017; Pérez-Laguna et al., 2018a; Pérez-Laguna et al., 2019). On the other hand, indocyanine green (ICG) has received clinical approval, but solely in dentistry as an adjuvant approach (Parker, 2013; Wainwright et al., 2017). Other well-known examples are psolaren, riboflavin, rose Bengal, chlorophyll a, curcumin and hypericin. Figure 1 shows the 2D chemical structure of these molecules.
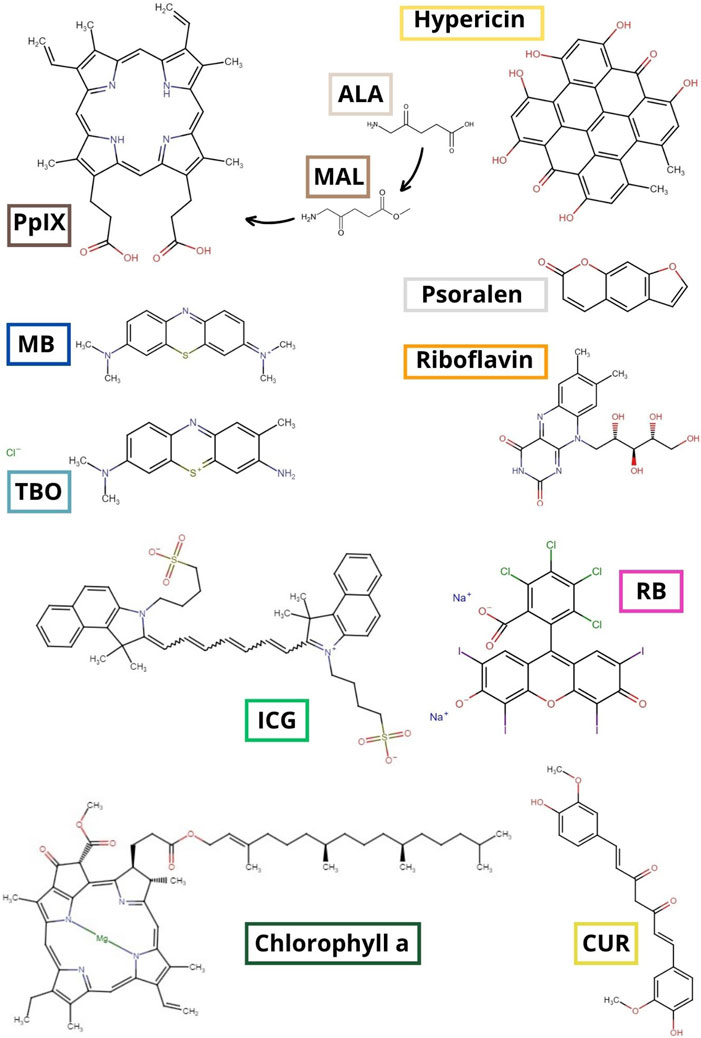
Figure 1. 2D chemical structure of the most commonly used PS. ALA: aminolevulinic acid; CUR: curcumin; ICG: indocyanine green; MAL: methyl-aminolevulinate; MB: methylene blue; PpIX: protoporphyrin IX; RB: rose Bengal; TBO: toluidine blue O (Bienfait and Ertl, 2013).
2.2 Light
Regarding the use of light in an aPDT procedure, as a clinician, you must make decisions regarding the λ, light source and dosage. The fundamental consideration is that the λ needs to match or, at least, fall within the absorption spectrum of the PS molecule to effectively initiate the photodynamic reaction (Pérez-Laguna et al., 2018a).
Light, in terms of electromagnetic radiation, is composed of massless elementary particles known as photons and energy-carrying waves that traverse through space with a blend of electric and magnetic fields. The dual nature of waves and particles contributes to their distinctive physical properties. To characterizing the waves, the distance between two successive wave crests is measured, and this parameter is referred to as λ. The less distance (short λ), the more energetic the wave is. At the top of Figure 2 we can see an illustration of the different wavelengths depending on the color. For example, red light has a longer λ than blue light, that is, in red light there is more distance between the consecutive crests of its wave. Therefore, the energy of red light is less than that of blue light (Pérez-Laguna et al., 2018a; Polat and Kang, 2021).
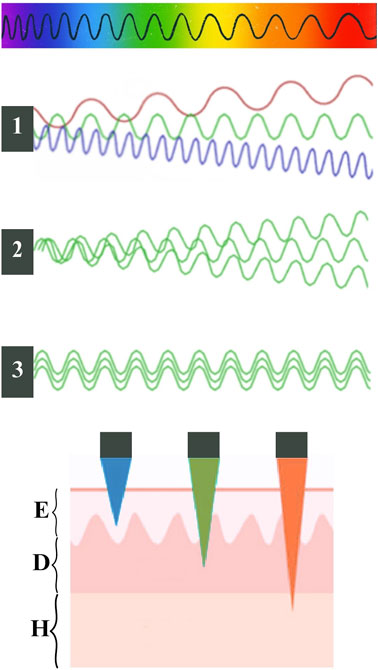
Figure 2. At the top, schematic illustration of the different wavelengths (λ) depending on the color of the light. In the middle of the schema, the black squares represent light sources, number one is a non-coherent polychromatic source (emits in a broad spectrum: green, red and blue); number two is a non-coherent monochromatic source (green), number three is a coherent source (same green λ and the same orientation). At the bottom, schematic representation adapted from Vicentini et al., 2017 of the penetration of light through the tissue depending on its color; E:epidermis, D:dermis, H: hypodermis.
The excitation of a PS occurs when it goes from its basal state to its excited state (PS*) by absorption of a photon (hv) when it is irradiated with a suitable λ light: PS + hѵ →PS* (Wainwright et al., 2017; Pérez-Laguna et al., 2018a).
The λ is determined by the light source. These can be classified into two types: non-coherent or coherent light: when the waves of the electric and magnetic fields are not synchronized or not maintain a constant phase relationship over time, it is called non-coherent light and can be monochromatic or polychromatic (Brancaleon and Moseley, 2002; Pérez-Laguna et al., 2018a; Polat and Kang, 2021). On the other hand, when all the emitted waves have the same λ and the same orientation (In phase), it is called coherent light (Figure 2) (Pérez-Laguna et al., 2018a).
Examples of different sources and some characteristics are presented in Table 2.
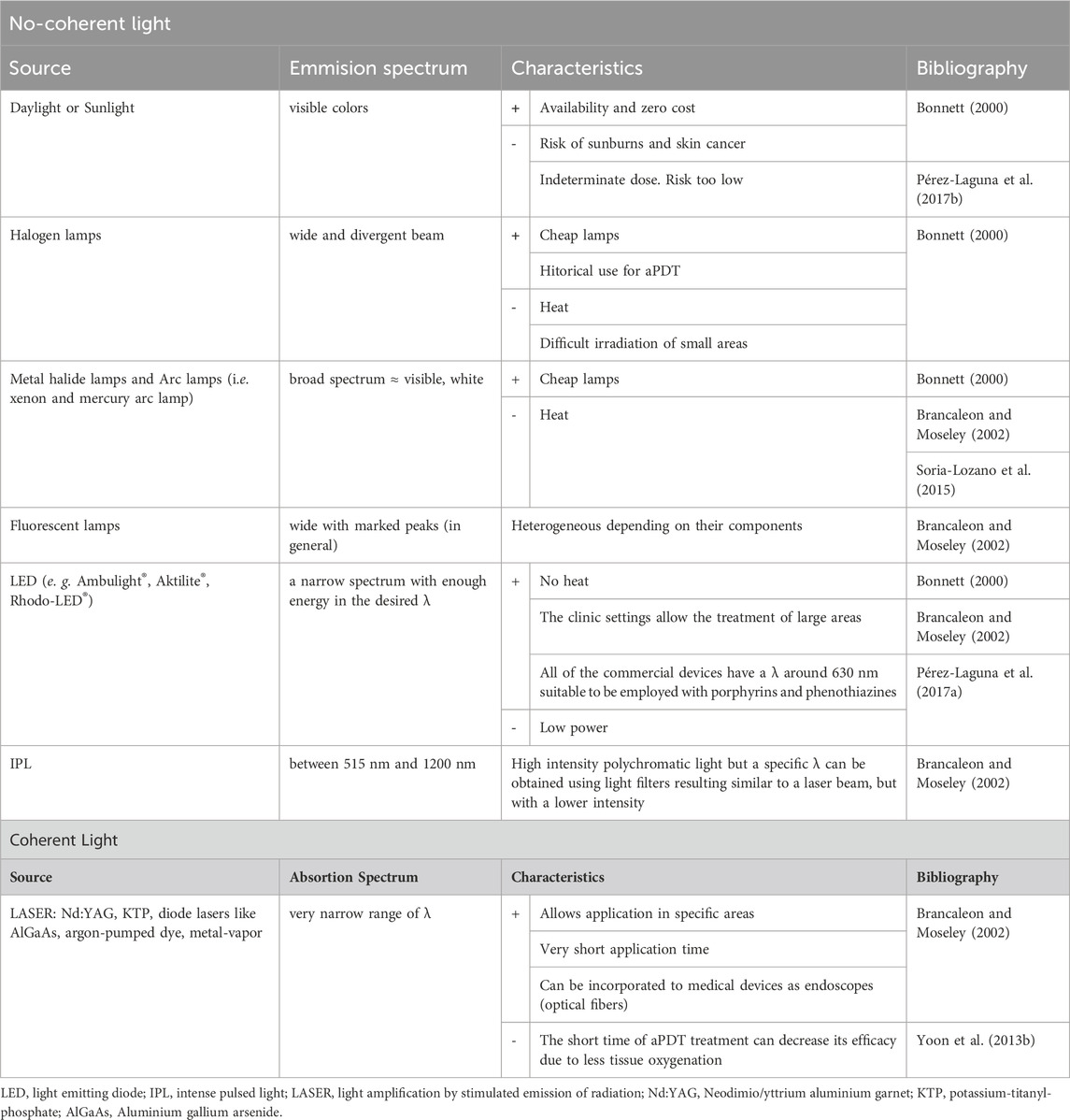
Table 2. Examples of sources of light for aPDT (Adapted from Pérez-Laguna et al., 2018a).
Regarding dosimetry of the light for an aPDT procedure depends on their power, measured in watts (W), the irradiance or power density, expressed in W/cm2, and the fluence, also called density or dose of energy, measured in J/cm2. The choice of the values of these magnitudes will depend on the pathology to be treated, the treatment area’s location and size, the source used, and other variables such as the characteristics of the PS. In addition, it is necessary to consider that with too low doses of light, there is sublethal damage, while at too high, there is an oxygen depletion that decreases the therapeutic efficacy (Morton et al., 2002; Pérez-Laguna et al., 2018a; Pérez-Laguna et al., 2019).
In addition, a key aspect to consider is the penetration of light into tissues. It is influenced by optical scattering and absorption due to endogenous tissue chromophores, such as hemoglobin (absorbing λ below 600 nm) and other molecules like NADH, collagen, and lipopigments. Besides melanin that has a main role. It absorbs across the visible spectrum (range between 400–750 nm) and is able to neutralize ROS. Therefore, understanding an individual’s phototype based on melanin quantity is essential for estimating light penetration into their skin. On the other hand, water absorption in tissues increases with λ above 1.300 nm. In sum, the optical window of the tissue is the range between 600 and 1.200 nm. Regarding the phototherapeutic window for aPDT, λ exceeding 850 nm are insufficient for an effective photodynamic reaction, making an optimal phototherapeutic window fall roughly between 650–850 nm. In this optimal range, a ideal PS should absorb between the red and the near-infrared to minimize light scattering and maximize tissue penetration. While not always a priority, this consideration enhances the overall effectiveness of the treatment. PS absorbing in the blue range offers higher energy efficiency, but lower tissue penetration compared to those absorbing in the red range (Morton et al., 2002; Yoon et al., 2013a; Pérez-Laguna et al., 2018a). Considering all the above, the penetration is estimated to be around 1–3 mm with a 630 nm λ and less with shorter λ (see Figure 2) (Vicentini et al., 2017).
3 aPDT in the treatment of dermatological infections
We conducted an exhaustive review of the literature on aPDT from 2000 to the present. Databases were searched in English and Spanish: PubMed, Web of Science, Scopus and Cochrane library. In the following sections, the most relevant studies about the use of aPDT to treat cutaneous infections caused by viruses, bacteria, fungi and parasites are presented.
3.1 aPDT target: cutaneous infections caused by virus
PDT has a direct effect on the treatment of bacteria and fungi, whereas in the case of viruses, its therapeutic mechanism is indirect (Hamblin and Hasan, 2004; Costa et al., 2012). In the case of using ALA as PS, iruses themselves lack the ability to produce PpIX in the presence of a PS and light energy. PDT takes advantage of the virus’s dependency on the infected host cell to exert its action (Wainwright, 2004). Two hypotheses have been proposed to explain this therapeutic action:
• Infected cells have an accelerated metabolism and capture the PS to a greater extent than healthy cells. This leads to a selective accumulation of PpIX, which, upon receiving light energy, causes cell death using mechanisms mentioned in previous sections. The death of the host cell prevents the virus from continuing to replicate within it, halting the infection chain (Costa et al., 2012).
• In the case of the herpes simplex virus, the PS has been described to bind to surface glycoproteins of the virus. This binding prevents virions from escaping the cell, as they are unable to fuse with the cell membrane for exocytosis (Wainwright, 2004; Costa et al., 2012).
The genetic variability of viruses and their mutations contribute to the development of resistances to targeted treatments. The indirect action of aPDT in inactivating viral replication is an advantage of this treatment. PDT offers many benefits in treating cutaneous viral infections; however, reactivation of herpes viruses and primary adenovirus infections have been described as adverse events of conventional aPDT (Hamblin and Hasan, 2004; Costa et al., 2012).
At times, viruses induce the appearance of precancerous lesions, such as in the case of human papillomavirus (HPV). The use of aPDT for the prevention and treatment of these lesions has been investigated with positive results (Wainwright, 2004; Costa et al., 2012).
Traditionally, ALA-aPDT has been used to treat localized viral infections. However, in recent years, the systemic effect of aPDT on the body’s immune response has been reported. Therefore, aPDT has been proposed as a photobiomodulator with antiviral effects against systemic viral infections such as COVID-19 (Fekrazad, 2020). Moreover, ALA-aPDT with MB is used in some countries like Canada for nasal decolonization and has also been tested for SARS-CoV-2 decolonization (Wainwright, 2003).
3.1.1 aPDT for human papillomavirus
HPV exhibits a wide range of subtypes, some of which lead to precancerous lesions. The main cutaneous HPV lesions are viral warts and condylomas. While these lesions occur in immunocompetent individuals, their severity and difficulty in eradication are more pronounced in immunocompromised individuals (Xie et al., 2019). aPDT can provide assistance in such cases. Certain studies report clearance rates of lesions surpassing conventional treatments like keratolytics (Rossi et al., 2009).
There isn't a single protocol for treating HPV lesions. Literature reports studies using both MAL-aPDT and ALA-aPDT for treating viral warts. Their results are better than placebo in wart clearance at 1 year. Improved results are observed when hyperkeratosis is curetted before PS application (Lavogiez et al., 2016). Figure 3 shows a viral wart treated with MAL-aPDT achieving good outcomes.
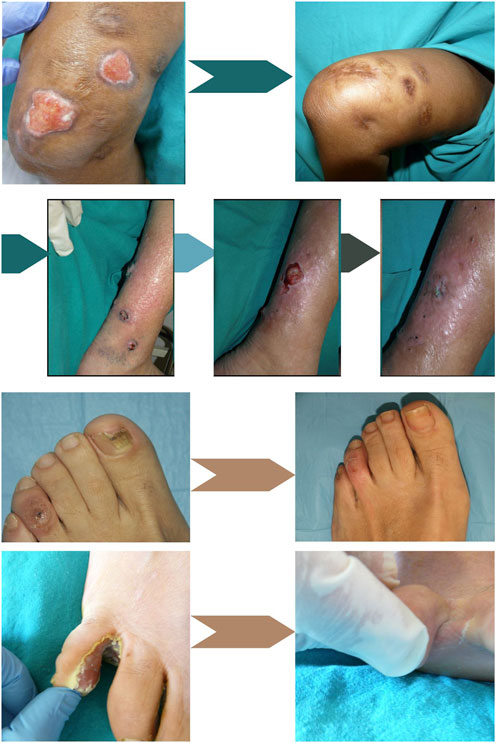
Figure 3. Sporotrichosis skin infection in a patient with chronic hepatitis C treated with low and intermittent doses of itraconazole and intralesional 1% MB solution aPDT. Upper left: Before treatment. Upper right: After treatment; Below, Alternaria spp. Skin infection treated sequentially with 11 sessions of MB-aPDT, 10 sessions of MAL-aPDT and 400 mg/day of voriconazole for 45 days. Left: At the beginning of MB-aPDT. Middle: single persistent lesion after aPDT using MB and MAL. Right: Cure after adding 45 days of treatment with voriconazole (6 months after the first treatment); Below, Onychomycosis due to T. rubrum and viral wart. Left: Before treatment. Right: Results after 3 sessions of MAL-aPDT. At the bottom, Interdigital infection by P. areuginosa resistant to treatment with oral ciprofloxacin. Left: Before treatment. Right: Results after 3 sessions of MAL-aPDT with 3 h of incubation separated by 1 week and oral ciprofloxacin adjuvant. Adapted from Gilaberte et al. (Almenara-Blasco et al., 2022) (Original photographs by the authors).
ALA has high affinity for HPV-infected keratinocytes; in the case of genital warts, fluorescence after its application is higher than in perilesional skin (Lavogiez et al., 2016). In pilot studies with 6-session ALA-aPDT protocols using white or red light, cure rates ranged from 50% to 100% at 4 months, maintaining good cosmetic results (Stender et al., 2000). The most effective wavelength for ALA-aPDT wart treatment has been studied, and white light was significantly more effective than red or blue light (Stender et al., 1999). ALA-aPDT therapy has also proven effective in treating periungual and subungual warts with cure rates up to 90% (Schroeter et al., 2007).
Common side effects of wart treatment with ALA or MAL-aPDT include pain and hyperpigmentation, with pain rated as intense or unbearable in 20% of cases. For these patients, prior local anesthesia before illumination and prescribing home analgesia is recommended (Smucler and Jatsová, 2005).
Other treatment modalities for warts include aPDT with 10% MB, which has yielded positive results. This treatment can be conducted using daylight, considerably reducing pain and costs (Fathy et al., 2017).
Condyloma acuminatum, another type of HPV-induced skin lesion, appears in the genital area and can coexist with precancerous or cancerous lesions due to HPV. ALA-aPDT has been studied for treating this condition with very satisfactory outcomes. Multiple clinical trials have shown that ALA-aPDT is equally effective as CO2 laser treatment or surgical excision for vulvar, vaginal, and male genital condylomas, with the added advantage of being more antimicrobial (Fehr et al., 2002). Additionally, PDT showed shorter healing times, excellent cosmetic results, and minimal tissue destruction. Treatment guidelines consisted of two sessions of 20% ALA-aPDT separated by 1 week. Recurrences were less than 30% in the first year, lower than CO2 laser treatment and with fewer adverse effects (Chen et al., 2007).
A recent systematic review and meta-analysis about the efficacy of aPDT of warts induced by HPV infection analyzed 19 randomized controlled clinical trials found the following results depending on the type of infection: 1) hand and foot warts, aPDT showed a statistically significant improvement in the wart clearance rate compared with placebo (p = 0.02), other lasers (p < 0.0001), and cryotherapy (p < 0.009); 2) plane wart group, aPDT demonstrated its superiority over placebo (p = 0.003) and cryotherapy (p = 0.007) in terms of the cure rate; and 3) condyloma acuminatum group was not superior in terms of the wart clearance rate, with a value lower than that of the carbon dioxide (CO2) laser (p = 0.003) and electrosurgical generator (p < 0.00001). However, all studies mentioned a significant decrease in the recurrence rate after aPDT. In conclusion, they recommend aPDT as a first-line therapy for warts (Shen et al., 2022).
3.1.2 aPDT for herpes virus infections
The treatment of herpes viruses with aPDT experienced its peak in the 1970s. Red light-neutral/proflavine was initially used as a PS with favorable results, but significant contact dermatitis emerged as an adverse event. Due to this, along with the extensive tissue necrosis that occurred, researchers started investigating other PS. MB demonstrated greater effectiveness at the same concentration in inactivating herpes viruses, adenoviruses, polyomaviruses coxsackieviruses and coronavirus (Wainwright, 2003; Praena et al., 2022).
Currently, MB is preferred as the PS for treating herpes viruses. However, aPDT treatment for herpes viruses is not standardized, and the risk-to-benefit ratio should be carefully assessed before proceeding with the treatment. Significant local inflammatory reactions have been described as adverse events (Wainwright, 2003). A systematic review that evaluates the efficacy of aPDT on the treatment of herpes labialis revealed that MB the most frequent PS, with only 1 study used 5-ALA; most studies used laser and one used a red light from halogen lamp; all articles reported good outcomes with resolution of disease and no recurrences after a maximum follow-up of 12 months; however due to the very few case reports and heterogeneity among protocols the quality of the evidence is weak (Lotufo et al., 2020).
Recently, two randomized controlled clinical trials carried out in children with herpetic gingivostomatitis and also in herpes labials have shown that the combination of aPDT with topical anti-viral therapy reported improvement in pain score, HSV-1 quantification and levels of proinflammatory cytokines statistically significant in comparison to both treatments separately (Ajmal, 2021; Vellappally et al., 2022).
3.2 aPDT target: cutaneous infections caused by bacteria
The most common therapy for infected ulcers and wounds is antibiotics; however, there is extensive antibiotic resistance among hospital pathogens. PS can be selectively taken up by bacteria, accumulating within bacterial cells and cytoplasmic membranes, or in their vicinity, where subsequent photodynamic reactions occur (Mahmoudi et al., 2018).
Gram-negative bacteria often exhibit innate resistance to multiple antibiotics, especially Pseudomonas aeruginosa, which complicates the search for new drugs and alternative therapies. This resistance is attributed to the fact that Gram-negative bacteria possess a double lipid bilayer, with peptidoglycan layer sandwiched between an outer layer of lipopolysaccharides, resulting in low permeability to small, lipophilic molecules (Sperandio et al., 2013; Mahmoudi et al., 2018). Therefore, for effective antimicrobial aPDT, the employed PS must penetrate the bacterial cell walls and reach the plasma membrane or cytoplasm. However, the bacterial cell membrane barriers limit the simple diffusion of the PS into the bacterial cytosol. This makes it more challenging to find highly potent PS for mediating aPDT in Gram-negative bacteria, as their membrane barrier prevents the absorption of anionic and neutral PSs (Pérez-Laguna et al., 2018a). Hence, efforts are made to optimize the chemical structure of the PS, such as creating positively charged (cationic) PS or coupling/combining them with positively charged entities like poly-L-lysine, polyethyleneimine, and polymyxin B (Sperandio et al., 2013; Del Valle et al., 2020).
Gram-positive bacteria and yeast are affected by neutral or anionic metal-free porphyrins, whereas Gram-negative bacteria are not. This resistance of Gram-negative bacteria to anionic porphyrin-mediated photosensitization has been widely reported. aPDT of Escherichia coli and P. aeruginosa with high concentrations of hematoporphyrin derivative or deuteroporphyrin combined with high light intensities did not result in bacterial inactivation (Tegos et al., 2006).
A straightforward approach to sensitize Gram-negative bacteria to aPDT is pretreating them with ethylenediaminetetraacetic acid (EDTA). It is known that brief treatment of Gram-negative cells with EDTA causes them to lose up to 50% of their lipopolysaccharide into the medium and become highly sensitive to hydrophobic agents. Studies pre-treated E. coli and Klebsiella pneumoniae with EDTA and conducted aPDT with hematoporphyrin, finding that cells maintained their resistance to hematoporphyrin and light exactly as before EDTA exposure. However, the use of non-porphyrin sensitizers like phthalocyanines and pre-treatment with tris-EDTA that altered the bacterial outer membrane could yield a good response for E. coli treatment (Bertoloni et al., 1984).
Acinetobacter baumannii is a clinically significant pathogen responsible for various infections in hospital settings. A. baumannii’s resistance to disinfection, oxidative stress, and its ability to form biofilms contribute to its persistence and role in healthcare-associated infections. The bacteria’s increasing resistance to antibiotics, particularly carbapenems, classifies it as a critical global threat. To address this, aPDT is explored as a potential alternative treatment. Cationic PS, such as chitosan + TBO, riboflavin-based compounds, and other porphyrin-based compounds, have shown promise in reducing A. baumannii viability (Bustamante and Palavecino, 2023). Hamblin MR et al. demonstrated that the addition of a solution containing a range of different inorganic salts potentiates the photodynamic action of many different PS especially against Gram-negative and Gram-positive bacteria. Phenothiazinium dyes, fullarenes and titanium dioxide are some of the PSs that benefit the most from this combination. Potassium iodide, potassium bromide potassium thiocyanate, potassium selenocyanate and sodium nitrite are non-toxic salts that may be clinically applicable (Hamblin and Abrahamse, 2018). aPDT has also been shown to be potentiated in a mouse model of oral candidiasis by addition of potassium iodine (Kashef and Hamblin, 2022).
In Figure 3 photos of an interdigital infection by P. areuginosa resistant to treatment with oral ciprofloxacin before treatment and after 3 sessions of MAL-aPDT with 3 h of incubation separated by 1 week and oral ciprofloxacin adjuvant are shown (Almenara-Blasco et al., 2022).
3.2.1 aPDT in the treatment of infected ulcers and wounds
Bacterial infections have a particularly detrimental effect on wound healing, especially when wounds already exhibit delayed healing (Sun et al., 2020). Bacterial infections slow down wound healing by prolonging the inflammatory phase and producing virulence factors such as enterotoxins, hemolysins, matrix metalloproteinases, and hyaluronidase. These factors overwhelm the host’s defenses, promote bacterial proliferation, and worsen local tissue destruction (Sun et al., 2020). aPDT reduces bacterial counts in the wound and stimulates wound healing (Nesi-Reis et al., 2018; Sun et al., 2020; Pérez et al., 2021). Therefore, aPDT could be considered as an alternative to antibiotic therapy for the treatment of infected leg ulcers (Kawczyk-Krupka et al., 2018; Pérez-Laguna et al., 2021)
In the first randomized controlled clinical trial of aPDT in chronic wounds (chronic leg ulcers and diabetic foot ulcers), a significant and broad-spectrum elimination of bacterial cells was demonstrated, along with a trend toward accelerated wound healing using the phenothiazinium derivative PPA904 as the PS (Lei et al., 2015). Other phthaloycanines derivatives have also demonstrated in clinical trials its efficacy for infected foot ulcers in patients with diabetes (Mannucci et al., 2014) Additionally, the effects of ALA-mediated aPDT for the treatment of chronic skin ulcers on the lower extremities infected with P. aeruginosa have been investigated (compared to red light treatment alone), with significantly improved healing outcomes in the ALA-aPDT group in terms of reduction in mean ulcer area. The use of aPDT has even been suggested as an option for diabetic foot ulcers for preventing amputations (Lei et al., 2015).
Recently, a systematic review and meta-analysis from randomized clinical trials about aPDT for treating infected skin wound have been published (de Oliveira et al., 2022). After analyzing 573 articles, only 4 were selected; regarding the mechanism of action they propose that aPDT reduces healing time and improved the healing process and wound oxygenation; all the studies used red LED light; patients treated with aPDT showed 15%–17% (p = 0.0003/I2 = 0%) lower microbial cell viability in the wound and a significantly smaller wound size (0.72 cm2/p = 0.0187/I2 = 0%) than patients treated with placebo or red-light exposure; therefore, they concluded that aPDT can be an excellent alterantive treatment for infected wounds, though larger trials are needed (de Oliveira et al., 2022). Finally, in our clinical experience, MB has has been particularly useful in the management of chronically infected ulcers. Two cases involving Fusarium spp and P. aeruginosa have been reported, both treated with conventional lamps and daylight aPDT, combined with conventional antimicrobials between the aPDT sessions, both with positive responses (Aspiroz et al., 2017). The advantages of MB as a PS are its short incubation period (20 min) and non-painful irradiation. However, this PS strongly stains tissues and perilesional skin with intense blue color (Figure 4) (Almenara-Blasco et al., 2022)
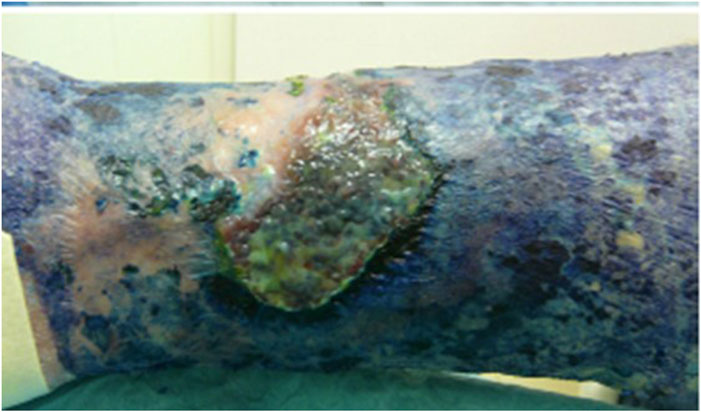
Figure 4. Intense bluish staining of tissues after treatment with MB. Source: Gilaberte et al. (Almenara-Blasco et al., 2022) (Original photograph by the authors).
Recently in Italy, the PS RLP068/CI has become available, designed alongside a 630 nm lamp for the treatment of diabetic foot ulcers. When used twice a week for 4 weeks to 5 months, it achieved a reduction of at least 40% in ulcer size. Furthermore, this treatment can be combined with other therapies and has demonstrated its effectiveness (Lorenzoni et al., 2021).
3.3 aPDT target: cutaneous infections caused by fungi
Fungi are eukaryotic organisms, and their resemblances to mammalian cells have posed significant challenges in the development of new drugs for treating fungal infections, often presenting a therapeutic hurdle due to their chronic and recurrent nature. aPDT offers an innovative therapeutic alternative in this domain, showcasing its utility across various studies (Baltazar et al., 2015).
The primary cutaneous fungal diseases are caused by dermatophytes, Malassezia spp., and Candida spp. (El-Gohary et al., 2014). In addition, some opportunistic fungi produce deep fungal cutaneous infections, especially in immunosuppressed patients (Chandorkar and Simkins, 2020).
Dermatophytosis stands as the most prevalent fungal infection worldwide. In its treatment, aPDT employs a distinct mechanism of action compared to antifungal drugs that mainly target ergosterol production. This therapy has effectively been demonstrated to inactivate T. rubrum, the most common causative agent of dermatophytosis (Nenoff et al., 2014). Clinical cases of Majocchi’s granulomas refractory to conventional treatments have responded favorably to ALA-aPDT (Shi et al., 2021).
aPDT has been successfully used also to treat tinea capitis. ALA and MAL, either with LED lamps or daylight, has been used to treat either inflammatory and non-inflammatory forms. The combination with ketonazole shampooing is necessary in order to reduce of the spores from the hair to avoid reinfections (Aspiroz et al., 2021; Zhang et al., 2022).
New PSs seem to be promising to treat tinea capitis and tinea corporis such as nanoencapsulated hypericin in P-123; this PS has shown in solve cutaneous infections caused by M. canis in animal after only 3 sessions (Galinari et al., 2021).
Malassezia spp. Can give rise to a variety of diseases, including pityriasis versicolor, which exhibits particular recurrence and relapse after conventional treatments. A case reveals that after red light-aPDT treatment (70–100 J/cm2), no hyphae or spores were evident in the affected areas 10 days post-treatment (Kim and Kim, 2007). Another condition attributed to this genus, albeit less frequent, is malassezia folliculitis, for which instances of aPDT effectiveness have been documented after three sessions of MAL-aPDT (Lee et al., 2010).
aPDT has great potential in treatment of sporotrichosis, as its fungicidal effect both in vitro and in vivo has clearly been demonstrated. aPDT could be used in conjunction with classic antifungal agents, such as itraconazole, reducing its dose the therefore its toxicity and side effects (Gilaberte et al., 2014). Intralesional MB seems to be the most effective PS and either LED lamps or daylight can be use (García-Malinis et al., 2018). It was treated through intralesional MB-assisted aPDT in combination with low-dose oral itraconazole (Gilaberte et al., 2014).
Figure 3 shows a photo of a Sporotrichosis skin infection in a patient with chronic hepatitis C treated with low and intermittent doses of itraconazole and intralesional aPDT with 1% MB (Almenara-Blasco et al., 2022).
Additionally, a case of chromoblastomycosis caused by Alternaria alternata has been reported, treated initially with itraconazole and subsequently with aPDT using 5-ALA as the PS, yielding a positive therapeutic response (Liu and Xia, 2014). Figure 3 depicts a cutaneous alternaria infection in an organ transplant recipient patient managed through a sequential approach of ALA and MB-PDT and only 1 month of voriconazole therapy.
3.3.1 aPDT for onychomycosis
aPDT for the treatment of onychomycosis has undergone extensive investigation. In a clinical trial involving 30 patients with onychomycosis caused by T. rubrum, unresponsive to any topical antifungal agents, the clinical and microbiological cure rate after 12 months of ALA-aPDT with 20% ALA and 40 J/cm2 red light was 43%, which declined to 36% at 18 months (Morton et al., 2020). Figueiredo Souza et al. conducted two studies with 80 patients, comparing ALA-aPDT with 2% MB administered at 15-day intervals and ablation of hyperkeratotic areas against oral fluconazole over 24 weeks. A healing rate of 90% was observed in the ALA-PDT group with MB, compared to 45% in the oral fluconazole group (Arca et al., 2002; Scher and Baran, 2003; Westerberg and Voyack, 2013; Robres et al., 2015).
On the other hand, a systematic review involving 214 patients summarized all the PSs and protocols tested up to date, concluding that ALA-aPDT appears to be effective in treating onychomycosis caused by different fungal species such as T. rubrum, T. mentagrophytes, T. interdigitale, Epidermophyton floccosum, Candida albicans, Acremonium spp, Fusarium oxysporum, and Aspergillus terreus. The primary challenge lies in the penetration of the PS, which might be overcome through pre-treatment with 40% urea or mechanical ablation, proving more effective than lasers (Bhatta et al., 2016). Some authors propose to encapsulate a PS, such as rose Bengal, in chitosan nanoparticles to enhance its penetration into the nail plate (Bekmukhametova et al., 2023).
Figure 3 shows photos of an onychomycosis due to T. rubrum and viral wart before treatment and after 3 sessions of MAL-aPDT (Almenara-Blasco et al., 2022)
ALA-PDT has also demonstrated efficacy in treating two patients with onychomycosis caused by F. oxysporum or A. terreus. In both cases, 40% urea ointment was applied under occlusion for 12 h for 7 days prior, followed by ALA-aPDT using 16% MAL cream and 630 nm LED illumination (dose of 37 J/cm2). A single treatment led to clinical improvement in nail appearance, and cultures turned negative. Two additional treatments were administered, with both patients remaining disease-free during follow-up (Gilaberte et al., 2011).
Considering that in most of the toenails onychomicosis are caused by dermatophytes that usually also infect the foot, it is necessary to treat the nail and the feet at the same time. For that reason, our protocol is to combine 3 sessions of MAL-aPDT with 1 month of oral terbinafine 250 mg/daily or topical if there is contraindication; using this protocol we obtained a complete clinical response in 80% of the cases and microbiological cure in 60% (Navarro-Bielsa et al., 2022).
3.3.2 aPDT for Candida spp. associated stomatitis
A study compared ALA-aPDT with topical nystatin for treating denture-related stomatitis caused by C. spp. In the first group, patients received topical nystatin treatment four times a day for 15 days. For each ALA-aPDT group, 500 mg/L of Photogem® was applied to their dentures and palates. After a 30-min incubation period, the Photogem-coated surfaces were illuminated with LED light (455 nm, doses of 37.5 and 122 J/cm2, respectively) three times a week for 15 days. Mycological cultures were taken from the dentures at the beginning (day 0), the end of treatment (day 15), and at follow-up intervals (days 30, 60, and 90). Both treatments significantly reduced fungal burden at the end of treatment and on day 30 of the follow-up period. However, no significant differences were observed between the two treatment modalities (53% vs. 45% for the nystatin and ALA-aPDT group, respectively). The study highlighted that fewer ALA-aPDT sessions were needed to achieve the same outcome, although the nystatin approach did not require in-person patient consultations (Mima et al., 2012).
3.4 aPDT target: cutaneous infections caused by parasites
Leishmaniasis is a disease transmitted by protozoa of the genus Leishmania spp., typically introduced into the body by infected sandflies during blood feeding (Enk et al., 2003). The treatment of leishmaniasis is complex due to resistance issues and the extended duration of treatments. One of the drugs used is pentavalent antimony; however, increasing reports of clinical isolates of L. spp. Resistant to this compound have limited its use (Sohl et al., 2007).
The strength of the recommendation to use aPDT for cutaneous leishmaniasis is B with the maximal quality of the evidence (I) (Morton et al., 2020). Among the described protocols is a weekly therapy of the lesion for 4 weeks with 10% ALA-aPDT, resulting in cure rates ranging from 90% to 100%. Other protocols vary the number of lesions treated per week and the occlusion times, which are typically 4 h for ALA-aPDT and 3 h for MAL-aPDT (Enk et al., 2003).
In 2015, Enk CD et al. conducted a clinical trial with daylight-aPDT for the treatment of L. major and L. tropical. The treatment protocol involved applying 16% MAL to the skin lesion, occluding it for 30 min, and then sequentially exposing it to sunlight for 2.5 h. Treatment was repeated weekly until clinical and microbiological cure was achieved. Cure was typically achieved about 5–7 weeks of treatment. The effectiveness of daylight MAL-aPDT was 89%, with clinical and microbiological resolution observed in 89% of cases. This aPDT modality is particularly valuable for children due to its lack of pain and the ability for parents to administer it at home (Song et al., 2011; Sbeghen et al., 2015).
The use of MB as a PS has been studied in vitro and in vivo to treat various Leishmania species. The results have been positive, although there isn't a clear guideline for its use. The norm involves a 1-h incubation followed by red light illumination, but fluences and session frequencies vary. MB presents advantages compared to MAL and ALA. The main benefit is that it doesn't rely on the host cell’s metabolism for parasite destruction. Cost is also crucial, as this disease is more prevalent in resource-limited countries (Pinto et al., 2017).
ALA-aPDT has also proven useful in treating mucocutaneous leishmaniasis in immunosuppressed patients undergoing anti-TNFα treatment, reducing or even replacing systemic treatment with liposomal amphotericin B (Cerro et al., 2020).
The aesthetic results are consistently good without causing disfigurement, and no recurrences have been observed in any case within the first 6 months (Enk et al., 2003).
3.4.1 aPDT for mite infections
Demodex folliculorum is a widespread ectoparasite that inhabits the pilosebaceous unit and is part of the human skin microbiome. It is particularly found on the face, where sebaceous glands are abundant. Demodex has been linked to various clinical conditions (rosacea, perioral dermatitis, blepharitis, follicular pityriasis, papulonodular demodicosis, etc.). aPDT has been used to treat some of these conditions, both for its eradication effect and its modulating effect on the pilosebaceous unit (Fan et al., 2018). There are no established protocols, and studies conducted are often isolated cases. The most used regimen has been 16% MAL in occlusion for 2–3 h followed by red light illumination at a dose of 37 J/cm2, with some studies repeating this weekly for up to 4 sessions (Gilaberte et al., 2009; Feuerman et al., 2022).
4 Discussion
The use of aPDT to treat dermatological infections is increasing in the last years. The present review summarizes the clinical trials and clinical cases that show the efficacy of aPDT against infections and cutaneous diseases caused by bacteria, fungi, viruses, parasites and even mites. In addition to the direct effect of aPDT killing the microorganisms, aPDT stimulates wound healing and also exerts some effect on the host immune system which can help to destroy secreted virulence factors enhancing its clinical effect.
aPDT is also effective against resistant strains and biofilm-forming bacteria, a notable challenge in clinical practice, and could be an excellent alternative of treatment for infections caused by a mixed of microorganism, something very common in chronic wounds.
Combination approaches, coupling aPDT with antibiotics, or antifungals or anti-virals, emerge as a promising strategy for tackling skin and mucocutaneous infections. Such combinations capitalize on synergistic or additive antimicrobial effects, potentially circumventing treatment failures by increasing microorganisms’ susceptibility to aPDT, or viceversa, and preventing regrowth during irradiation intervals. The concept of using combination treatments of aPDT and antimicrobials has far-reaching implications. It could potentially counteract the formidable challenge posed by high-level resistance microorganisms. Given its multi-target nature, aPDT could impede the selection of resistant strains, adding a layer of complexity to the evolution of antimicrobial resistance. This approach holds promise in dermatological infections like onychomycoses, deep fungal infections, and chronic wounds, where additive or synergistic effects have been observed.
However, there are some limitations for the widely use of aPDT in the clinical setting: photoinactivation of microbes is and exclusive localized process and lasts during the light is on, allowing the remaining microorganisms to regrow after; aPDT should be performed in the clinic being more time consuming for doctors and nurses than just prescribing a pill. This is true, but without any doubt, aPDT could be the best alternative of treatment, alone or in combination with conventional antimicrobials, to treat recalcitrant infections and fragile patients, especially immunosuppressed ones.
Homebase protocols, portable light devices and the use of daylight, besides more research in developing or adapting the PS to the different type of infections are needed. While hurdles remain in terms of application duration, administration, and industry interest, combination treatments that leverage aPDT’s strengths could be a promising solution to address the challenges posed by antimicrobial resistance. Continued research and development are essential to harness the full potential of this approach for clinical benefit.
Author contributions
MA-B: Writing–original draft, Writing–review and editing. VP-L: Writing–original draft, Writing–review and editing. AN-B: Writing–review and editing. TG-C: Writing–review and editing, Conceptualization, Supervision. YG: Conceptualization, Methodology, Supervision, Writing–original draft, Writing–review and editing.
Funding
The author(s) declare that no financial support was received for the research, authorship, and/or publication of this article.
Acknowledgments
The authors would like to express their gratitude for the collaboration in enhancing the manuscript to Francisco Galindo, Full Professor of Chemistry at the Universitat Jaume I (UJI) in Castellón, Spain. They also want to express their gratitude to the members of the research group recognized by the Government of Aragon, B59-23D in Dermatology and Photobiology.
Conflict of interest
The authors declare that the research was conducted in the absence of any commercial or financial relationships that could be construed as a potential conflict of interest.
The author(s) declared that they were an editorial board member of Frontiers, at the time of submission. This had no impact on the peer review process and the final decision.
Publisher’s note
All claims expressed in this article are solely those of the authors and do not necessarily represent those of their affiliated organizations, or those of the publisher, the editors and the reviewers. Any product that may be evaluated in this article, or claim that may be made by its manufacturer, is not guaranteed or endorsed by the publisher.
References
Abrahamse, H., and Hamblin, M. R. (2016). New photosensitizers for photodynamic therapy. Biochem. J. 473 (4), 347–364. doi:10.1042/BJ20150942
Ajmal, M. (2021). Effectiveness of photodynamic therapy as an adjunct to topical antiviral therapy in the treatment of herpes labialis: a randomized controlled clinical trial. Photodiagnosis Photodyn. Ther. 34, 102302. doi:10.1016/j.pdpdt.2021.102302
Almenara-Blasco, M., García-Gil, M., and Gilaberte, Y. (2022). “Terapia fotodinámica antimicrobiana,” in Manual de Terapia Fotodinámica para Dermatología. 1a. Editors T Gracia-Cazaña, and Y Gilaberte (Madrid: Aulamédica), 181.
Arca, E., Taştan, H. B., Akar, A., Kurumlu, Z., and Gür, A. R. (2002). An open, randomized, comparative study of oral fluconazole, itraconazole and terbinafine therapy in onychomycosis. J. Dermatol. Treat. 13 (1), 3–9. doi:10.1080/09546630252775171
Aspiroz, C., Melcon, B., Cerro, P. A., and Gilaberte, Y. (2021). Tinea capitis caused by Microsporum canis treated with methyl-aminolevulinate daylight photodynamic therapy and ketoconazole shampooing. Photodermatol. Photoimmunol. Photomed. 37, 567–568. doi:10.1111/phpp.12706
Aspiroz, C., Sevil, M., Toyas, C., and Gilaberte, Y. (2017). Photodynamic therapy with methylene blue for skin ulcers infected with Pseudomonas aeruginosa and Fusarium spp. Actas Dermosifiliogr. 108 (6), e45–e48. doi:10.1016/j.ad.2016.11.020
Baltazar, L. M., Ray, A., Santos, D. A., Cisalpino, P. S., Friedman, A. J., and Nosanchuk, J. D. (2015). Antimicrobial photodynamic therapy: an effective alternative approach to control fungal infections. Front. Microbiol. 6 (MAR), 202–211. doi:10.3389/fmicb.2015.00202
Bekmukhametova, A., Antony, A., Halliday, C., Chen, S., Ho, C. H., Uddin, M. M. N., et al. (2023). Rose bengal-encapsulated chitosan nanoparticles for the photodynamic treatment of Trichophyton species. Photochem Photobiol. 100, 115–128. doi:10.1111/php.13839
Bertoloni, G., Salvato, B., Dall’Acqua, M., Vazzoler, M., and Jori, G. (1984). Hematoporphyrin-sensitized photoinactivation of Streptococcus faecalis. Photochem Photobiol. 39 (6), 811–816. doi:10.1111/j.1751-1097.1984.tb08864.x
Bhatta, A. K., Keyal, U., and Wang, X. L. (2016). Photodynamic therapy for onychomycosis: a systematic review. Photodiagnosis Photodyn. Ther. 15, 228–235. doi:10.1016/j.pdpdt.2016.07.010
Bienfait, B., and Ertl, P. (2013). JSME: a free molecule editor in JavaScript. J. Cheminform 5, 24. doi:10.1186/1758-2946-5-24
Brancaleon, L., and Moseley, H. (2002). Laser and non-laser light sources for photodynamic therapy. Lasers Med. Sci. 17 (3), 173–186. doi:10.1007/s101030200027
Bustamante, V., and Palavecino, C. E. (2023). Effect of photodynamic therapy on multidrug-resistant Acinetobacter baumannii: a scoping review. Photodiagnosis Photodyn. Ther. 43, 103709. doi:10.1016/j.pdpdt.2023.103709
Cerro, P. A., Sánchez-Hernández, M., Morales-Callaghan, A. M., Estrada-Mallarino, N., and Gilaberte, Y. (2020). Successful treatment with photodynamic therapy in a patient with nasal mucocutaneous leishmaniasis undergoing treatment with TNFα inhibitor. Eur. J. Dermatol 30 (6), 750–751. doi:10.1684/ejd.2020.3921
Chandorkar, A., and Simkins, J. (2020). Emerging fungal cutaneous infections in immunocompromised patients. Curr. Fungal Infect. Rep. 14 (3), 217–224. doi:10.1007/s12281-020-00395-2
Chen, K., Chang, B. Z., Ju, M., Zhang, X. H., and Gu, H. (2007). Comparative study of photodynamic therapy vs CO2 laser vaporization in treatment of condylomata acuminata: a randomized clinical trial. Br. J. Dermatol 156 (3), 516–520. doi:10.1111/j.1365-2133.2006.07648.x
Cieplik, F., Deng, D., Crielaard, W., Buchalla, W., Hellwig, E., Al-Ahmad, A., et al. (2018). Antimicrobial photodynamic therapy - what we know and what we don’t. Crit. Rev. Microbiol. 44 (5), 571–589. doi:10.1080/1040841X.2018.1467876
Cieplik, F., Tabenski, L., Buchalla, W., and Maisch, T. (2014). Antimicrobial photodynamic therapy for inactivation of biofilms formed by oral key pathogens. Front. Microbiol. 5, 405. doi:10.3389/fmicb.2014.00405
Costa, L., Faustino, M. A. F., Neves, MGPMS, Cunha, A., and Almeida, A. (2012). Photodynamic inactivation of mammalian viruses and bacteriophages. Viruses 4 (7), 1034–1074. doi:10.3390/v4071034
Dai, T., Huang, Y. Y., and Hamblin, M. R. (2009). Photodynamic therapy for localized infections--state of the art. Photodiagnosis Photodyn. Ther. 6 (3–4), 170–188. doi:10.1016/j.pdpdt.2009.10.008
Del Valle, C. A., Pérez-Laguna, V., Resta, I. M., Gavara, R., Carles, F.-L., Miravet, J. F., et al. (2020). A cost-effective combination of Rose Bengal and off-the-shelf cationic polystyrene for the photodynamic inactivation of Pseudomonas aeruginosa. Mater Sci. Eng. C Mater Biol. Appl. 117 (111302), 111302. doi:10.1016/j.msec.2020.111302
de Oliveira, A. B., Ferrisse, T. M., Fontana, C. R., Basso, F. G., and Brighenti, F. L. (2022). Photodynamic therapy for treating infected skin wounds: a systematic review and meta-analysis from randomized clinical trials. Photodiagnosis Photodyn. Ther. 40, 103118. doi:10.1016/j.pdpdt.2022.103118
Eix, E. F., and Nett, J. E. (2022). Modeling Candida auris skin colonization: mice, swine, and humans. PLoS Pathog. 18 (9), 1010730. doi:10.1371/journal.ppat.1010730
El-Gohary, M., van Zuuren, E. J., Fedorowicz, Z., Burgess, H., Doney, L., Stuart, B., et al. (2014). Topical antifungal treatments for tinea cruris and tinea corporis. Cochrane Database Syst. Rev. (8), 2014. CD009992. doi:10.1002/14651858.CD009992.pub2
Enk, C. D., Fritsch, C., Jonas, F., Nasereddin, A., Ingber, A., Jaffe, C. L., et al. (2003). Treatment of cutaneous leishmaniasis with photodynamic therapy. Arch. Dermatol 139 (4), 432–434. doi:10.1001/archderm.139.4.432
Fan, L., Yin, R., Lan, T., and Hamblin, M. R. (2018). Photodynamic therapy for rosacea in Chinese patients. Photodiagnosis Photodyn. Ther. 24, 82–87. doi:10.1016/j.pdpdt.2018.08.005
Fathy, G., Asaad, M. K., and Rasheed, H. M. (2017). Daylight photodynamic therapy with methylene blue in plane warts: a randomized double-blind placebo-controlled study. Photodermatol. Photoimmunol. Photomed. 33 (4), 185–192. doi:10.1111/phpp.12291
Fehr, M. K., Hornung, R., Degen, A., Schwarz, V. A., Fink, D., Haller, U., et al. (2002). Photodynamic therapy of vulvar and vaginal condyloma and intraepithelial neoplasia using topically applied 5-aminolevulinic acid. Lasers Surg. Med. 30 (4), 273–279. doi:10.1002/lsm.10048
Fekrazad, R. (2020). Photobiomodulation and antiviral photodynamic therapy as a possible novel approach in COVID-19 management. Photobiomodulation, Photomed. laser Surg. 38, 255–257. doi:10.1089/photob.2020.4868
Feuerman, H., Atzmony, L., Glick, M., Sherman, S., Snast, I., Hodak, E., et al. (2022). Pigmented demodicidosis - an under-recognized cause of facial hyperpigmentation. Int. J. Dermatol 61 (5), 564–569. doi:10.1111/ijd.15992
Galinari, C. B., Conrado, P. C. V., Arita, G. S., Mosca, V. A. B., Melo, R. C., Bianchi, T. de P., et al. (2021). Nanoencapsulated hypericin in P-123 associated with photodynamic therapy for the treatment of dermatophytosis. J. Photochem Photobiol. B 215, 112103. doi:10.1016/j.jphotobiol.2020.112103
García-Malinis, A. J., Milagro Beamonte, A., Torres Sopena, L., García-Callen, O., Puertolas-Villacampa, P., and Gilaberte, Y. (2018). Cutaneous sporotrichosis treated with methylene blue-daylight photodynamic therapy. J. Eur. Acad. Dermatology Venereol. 32, e90–e91. doi:10.1111/jdv.14545
Garin, C., Alejo, T., Perez-Laguna, V., Prieto, M., Mendoza, G., Arruebo, M., et al. (2021). Chalcogenide nanoparticles and organic photosensitizers for synergetic antimicrobial photodynamic therapy. J. Mater Chem. B 9 (31), 6246–6259. doi:10.1039/d1tb00972a
Gilaberte, Y., Aspiroz, C., Alejandre, M. C., Andres-Ciriano, E., Fortuño, B., Charlez, L., et al. (2014). Cutaneous sporotrichosis treated with photodynamic therapy: an in vitro and in vivo study. Photomed. Laser Surg. 32 (1), 54–57. doi:10.1089/pho.2013.3590
Gilaberte, Y., Aspiroz, C., Martes, M. P., Alcalde, V., Espinel-Ingroff, A., and Rezusta, A. (2011). Treatment of refractory fingernail onychomycosis caused by nondermatophyte molds with methylaminolevulinate photodynamic therapy. J. Am. Acad. Dermatology 65, 669–671. doi:10.1016/j.jaad.2010.06.008
Gilaberte, Y., Frias, M. P., Rezusta, A., and Vera-Alvarez, J. (2009). Photodynamic therapy with methyl aminolevulinate for resistant scalp folliculitis secondary to Demodex infestation. J. Eur. Acad. Dermatology Venereol. 23, 718–719. doi:10.1111/j.1468-3083.2009.03171.x
Hamblin, M. R., and Abrahamse, H. (2018). Inorganic salts and antimicrobial photodynamic therapy: mechanistic conundrums? Molecules 23 (12), 3190. doi:10.3390/molecules23123190
Hamblin, M. R., and Hasan, T. (2004). Photodynamic therapy: a new antimicrobial approach to infectious disease? Photochem Photobiol. Sci. 3 (5), 436–450. doi:10.1039/b311900a
JMOL (2023). JMOL: an open-source Java viewer for chemical structures in 3D. Available at: https://jmol.sourceforge.net/.
Kashef, N., and Hamblin, M. R. (2022). In vivo potentiation of antimicrobial photodynamic therapy in a mouse model of fungal infection by addition of potassium iodide. Methods Mol. Biol. 2451, 621–630. doi:10.1007/978-1-0716-2099-1_33
Kawczyk-Krupka, A., Pucelik, B., Międzybrodzka, A., Sieroń, A. R., and Dąbrowski, J. M. (2018). Photodynamic therapy as an alternative to antibiotic therapy for the treatment of infected leg ulcers. Photodiagnosis Photodyn. Ther. 23, 132–143. doi:10.1016/j.pdpdt.2018.05.001
Kim, Y. J., and Kim, Y. C. (2007). Successful treatment of pityriasis versicolor with 5-aminolevulinic acid photodynamic therapy. Archives dermatology 143, 1218–1220. doi:10.1001/archderm.143.9.1218
Lavogiez, C., Mortier, L., and Mordon, S. (2016). Antimicrobial photodynamic therapy in dermatology. Part II: viral and parasitic infections; perspectives. Ann. Dermatol Venereol. 143 (1), 62–69. doi:10.1016/j.annder.2015.02.019
Lee, J. W., Kim, B. J., and Kim, M. N. (2010). Photodynamic therapy: new treatment for recalcitrant Malassezia folliculitis. Lasers Surg. Med. 42 (2), 192–196. doi:10.1002/lsm.20857
Lei, X., Liu, B., Huang, Z., and Wu, J. (2015). A clinical study of photodynamic therapy for chronic skin ulcers in lower limbs infected with Pseudomonas aeruginosa. Arch. Dermatol Res. 307 (1), 49–55. doi:10.1007/s00403-014-1520-4
Liu, Z. H., and Xia, X. J. (2014). Successful sequential treatment with itraconazole and ALA-PDT for chromoblastomycosis because of Alternaria alternata. Dermatol Ther. 27 (6), 357–360. doi:10.1111/dth.12149
Lorenzoni, V., Chiavetta, A., Curci, V., Pepa, G. D., Licciardello, C., Pantò, F., et al. (2021). New perspective to improve care of patients with infected diabetic foot ulcer: early economic impact of the use of photodynamic therapy with RLP068 (based) system. Clin. Outcomes Res. 13, 135–144. doi:10.2147/CEOR.S274897
Lotufo, M. A., Tempestini Horliana, A. C. R., Santana, T., de Queiroz, A. C., Gomes, A. O., Motta, L. J., et al. (2020). Efficacy of photodynamic therapy on the treatment of herpes labialis: a systematic review. Photodiagnosis Photodyn. Ther. 29, 101536. doi:10.1016/j.pdpdt.2019.08.018
Mahmoudi, H., Bahador, A., Pourhajibagher, M., and Alikhani, M. Y. (2018). Antimicrobial photodynamic therapy: an effective alternative approach to control bacterial infections. J. Lasers Med. Sci. 9 (3), 154–160. doi:10.15171/jlms.2018.29
Mannucci, E., Genovese, S., Monami, M., Navalesi, G., Dotta, F., Anichini, R., et al. (2014). Photodynamic topical antimicrobial therapy for infected foot ulcers in patients with diabetes: a randomized, double-blind, placebo-controlled study--the D.A.N.T.E (Diabetic ulcer Antimicrobial New Topical treatment Evaluation) study. Acta Diabetol. 51 (3), 435–440. doi:10.1007/s00592-013-0533-3
Marioni, J., Bresolí-Obach, R., Agut, M., Comini, L. R., Cabrera, J. L., Paraje, M. G., et al. (2017). On the mechanism of Candida tropicalis biofilm reduction by the combined action of naturally-occurring anthraquinones and blue light. PLoS One 12 (7), e0181517. doi:10.1371/journal.pone.0181517
Marsh, P. D., Moter, A., and Devine, D. A. (2011). Dental plaque biofilms: communities, conflict and control. Periodontol 55 (1), 16–35. doi:10.1111/j.1600-0757.2009.00339.x
Mima, E. G., Vergani, C. E., Machado, A. L., Massucato, E. M. S., Colombo, A. L., Bagnato, V. S., et al. (2012). Comparison of Photodynamic Therapy versus conventional antifungal therapy for the treatment of denture stomatitis: a randomized clinical trial. Clin. Microbiol. Infect. 18 (10), E380–E388. doi:10.1111/j.1469-0691.2012.03933.x
Morgan, A. R., Garbo, G. M., Keck, R. W., Eriksen, L. D., and Selman, S. H. (1990). Metallopurpurins and light: effect on transplantable rat bladder tumors and murine skin. Photochem Photobiol. 51 (5), 589–592. doi:10.1111/j.1751-1097.1990.tb01970.x
Morton, C. A., Brown, S. B., Collins, S., Ibbotson, S., Jenkinson, H., Kurwa, H., et al. (2002). Guidelines for topical photodynamic therapy: report of a workshop of the British Photodermatology Group. Br. J. Dermatol 146 (4), 552–567. doi:10.1046/j.1365-2133.2002.04719.x
Morton, C. A., Szeimies, R. M., Basset-Séguin, N., Calzavara-Pinton, P. G., Gilaberte, Y., Haedersdal, M., et al. (2020). European Dermatology Forum guidelines on topical photodynamic therapy 2019 Part 2: emerging indications - field cancerization, photorejuvenation and inflammatory/infective dermatoses. J. Eur. Acad. Dermatol Venereol. 34 (1), 17–29. doi:10.1111/jdv.16044
Navarro-Bielsa, A., Gracia-Cazaña, T., Robres, P., Lopez, C., Calvo-Priego, M. D., Aspiroz, C., et al. (2022). Combination of photodynamic therapy and oral antifungals for the treatment of onychomycosis. Pharm. (Basel) 15 (6), 722. doi:10.3390/ph15060722
Nenoff, P., Grunewald, S., and Paasch, U. (2014). Laser therapy of onychomycosis. J. Dtsch. Dermatol Ges. 12 (1), 33–38. doi:10.1111/ddg.12251
Nesi-Reis, V., Lera-Nonose, DSSL, Oyama, J., Silva-Lalucci, M. P. P., Demarchi, I. G., Aristides, S. M. A., et al. (2018). Contribution of photodynamic therapy in wound healing: a systematic review. Photodiagnosis Photodyn. Ther. 21, 294–305. doi:10.1016/j.pdpdt.2017.12.015
Parker, S. (2013). The use of diffuse laser photonic energy and indocyanine green photosensitiser as an adjunct to periodontal therapy. Br. Dent. J. 215 (4), 167–171. doi:10.1038/sj.bdj.2013.790
Pérez, M., Robres, P., Moreno, B., Bolea, R., Verde, M. T., Pérez-Laguna, V., et al. (2021). Comparison of antibacterial activity and wound healing in a superficial abrasion mouse model of staphylococcus aureus skin infection using photodynamic therapy based on Methylene Blue or mupirocin or both. Front. Med. (Lausanne) 8, 673408. doi:10.3389/fmed.2021.673408
Pérez-Laguna, V., Garcia-Luque, I., Ballesta, S., Pérez-Artiaga, L., Lampaya-Pérez, V., Samper, S., et al. (2018b). Antimicrobial photodynamic activity of Rose Bengal, alone or in combination with Gentamicin, against planktonic and biofilm Staphylococcus aureus. Photodiagnosis Photodyn. Ther. 21, 211–216. doi:10.1016/j.pdpdt.2017.11.012
Pérez-Laguna, V., Garcia-Luque, I., Ballesta, S., Rezusta, A., and Gilaberte, Y. (2021). Photodynamic therapy combined with antibiotics or antifungals against microorganisms that cause skin and soft tissue infections: a planktonic and biofilm approach to overcome resistances. Pharm. (Basel) 14 (7), 603. doi:10.3390/ph14070603
Pérez-Laguna, V., Garcia-Malinis, A. J., Aspiroz, C., Rezusta, A., and Gilaberte, Y. (2018a). Antimicrobial effects of photodynamic therapy. G. Ital. Dermatol Venereol. 153 (6), 833–846. doi:10.23736/S0392-0488.18.06007-8
Pérez-Laguna, V., Gilaberte, Y., Millán-Lou, M. I., Agut, M., Nonell, S., Rezusta, A., et al. (2019). A combination of photodynamic therapy and antimicrobial compounds to treat skin and mucosal infections: a systematic review. Photochem Photobiol. Sci. 18 (5), 1020–1029. doi:10.1039/c8pp00534f
Pérez-Laguna, V., Pérez-Artiaga, L., Lampaya-Pérez, V., García-Luque, I., Ballesta, S., Nonell, S., et al. (2017a). Bactericidal effect of photodynamic therapy, alone or in combination with mupirocin or linezolid, on Staphylococcus aureus. Front. Microbiol. 8, 8. doi:10.3389/fmicb.2017.01002
Pérez-Laguna, V., Rezusta, A., Ramos, J. J., Ferrer, L. M., Gené, J., Revillo, M. J., et al. (2017b). Daylight photodynamic therapy using methylene blue to treat sheep with dermatophytosis caused by Arthroderma vanbreuseghemii. Small Ruminant Res. 150, 97–101. doi:10.1016/j.smallrumres.2017.03.011
Pinto, J. G., Martins, J. F. de S., Pereira, A. H. C., Mittmann, J., Raniero, L. J., and Ferreira-Strixino, J. (2017). Evaluation of methylene blue as photosensitizer in promastigotes of Leishmania major and Leishmania braziliensis. Photodiagnosis Photodyn. Ther. 18, 325–330. doi:10.1016/j.pdpdt.2017.04.009
Polat, E., and Kang, K. (2021). Natural photosensitizers in antimicrobial photodynamic therapy. Biomedicines 9 (6), 584. doi:10.3390/biomedicines9060584
Praena, B., Mascaraque, M., Andreu, S., Bello-Morales, R., Abarca-Lachen, E., Rapozzi, V., et al. (2022). Potent virucidal activity in vitro of photodynamic therapy with Hypericum extract as photosensitizer and white light against human coronavirus HCoV-229E. Pharmaceutics 14 (11), 2364. doi:10.3390/pharmaceutics14112364
Robres, P., Aspiroz, C., Rezusta, A., and Gilaberte, Y. (2015). Usefulness of photodynamic therapy in the management of onychomycosis. Actas Dermosifiliogr. 106 (10), 795–805. doi:10.1016/j.ad.2015.08.005
Rossi, R., Bruscino, N., Ricceri, F., Grazzini, M., Dindelli, M., and Lotti, T. (2009). Photodynamic treatment for viral infections of the skin. G. Ital. Dermatol Venereol. 144 (1), 79–83.
Sabino, C. P., Ribeiro, M. S., Wainwright, M., Dos Anjos, C., Sellera, F. P., Dropa, M., et al. (2023). The biochemical mechanisms of antimicrobial photodynamic therapy(†). Photochem Photobiol. 99 (2), 742–750. doi:10.1111/php.13685
Sbeghen, M. R., Voltarelli, E. M., Campois, T. G., Kimura, E., Aristides, S. M. A., Hernandes, L., et al. (2015). Topical and intradermal efficacy of photodynamic therapy with methylene blue and light-emitting Diode in the treatment of cutaneous leishmaniasis caused by Leishmania braziliensis. J. Lasers Med. Sci. 6 (3), 106–111. doi:10.15171/jlms.2015.03
Scher, R. K., and Baran, R. (2003). Onychomycosis in clinical practice: factors contributing to recurrence. Br. J. Dermatol 149, 5–9. doi:10.1046/j.1365-2133.149.s65.5.x
Schroeter, C. A., Kaas, L., Waterval, J. J., Bos, P. M., and Neumann, H. A. M. (2007). Successful treatment of periungual warts using photodynamic therapy: a pilot study. J. Eur. Acad. Dermatol Venereol. 21 (9), 1170–1174. doi:10.1111/j.1468-3083.2007.02081.x
Sellera, F. P., Sabino, C. P., Núñez, S. C., and Ribeiro, M. S. (2022). Clinical acceptance of antimicrobial photodynamic therapy in the age of WHO global priority pathogens: so what we need to move forward? Photodiagnosis Photodyn. Ther. 40, 103158. doi:10.1016/j.pdpdt.2022.103158
Shen, S., Feng, J., Song, X., and Xiang, W. (2022). Efficacy of photodynamic therapy for warts induced by human papilloma virus infection: a systematic review and meta-analysis. Photodiagnosis Photodyn. Ther. 39, 102913. doi:10.1016/j.pdpdt.2022.102913
Shi, L., Wu, Q., Yang, J., Tan, J., Yang, H., Hu, C., et al. (2021). ALA-PDT successfully treated Majocchi’s granuloma by directly killing Trichophyton tonsurans and recruiting T lymphocytes. Photodiagnosis Photodyn. Ther. 35, 102328. doi:10.1016/j.pdpdt.2021.102328
Smucler, R., and Jatsová, E. (2005). Comparative study of aminolevulic acid photodynamic therapy plus pulsed dye laser versus pulsed dye laser alone in treatment of viral warts. Photomed. Laser Surg. 23 (2), 202–205. doi:10.1089/pho.2005.23.202
Sohl, S., Kauer, F., Paasch, U., and Simon, J. C. (2007). Photodynamic treatment of cutaneous leishmaniasis. J. Dtsch. Dermatol Ges. 5 (2), 128–130. doi:10.1111/j.1610-0387.2007.06177.x
Song, D., Lindoso, J. A. L., Oyafuso, L. K., Kanashiro, E. H. Y., Cardoso, J. L., Uchoa, A. F., et al. (2011). Photodynamic therapy using methylene blue to treat cutaneous leishmaniasis. Photomed. Laser Surg. 29 (10), 711–715. doi:10.1089/pho.2010.2915
Soria-Lozano, P., Gilaberte, Y., Paz-Cristobal, M., Pérez-Artiaga, L., Lampaya-Pérez, V., Aporta, J., et al. (2015). In vitro effect photodynamic therapy with differents photosensitizers on cariogenic microorganisms. BMC Microbiol. 15 (1), 187. doi:10.1186/s12866-015-0524-3
Sperandio, F. F., Huang, Y. Y., and Hamblin, M. R. (2013). Antimicrobial photodynamic therapy to kill Gram-negative bacteria. Recent Pat. Antiinfect Drug Discov. 8 (2), 108–120. doi:10.2174/1574891x113089990012
Stender, I. M., Lock-Andersen, J., and Wulf, H. C. (1999). Recalcitrant hand and foot warts successfully treated with photodynamic therapy with topical 5-aminolaevulinic acid: a pilot study. Clin. Exp. Dermatol 24 (3), 154–159. doi:10.1046/j.1365-2230.1999.00441.x
Stender, I. M., Na, R., Fogh, H., Gluud, C., and Wulf, H. C. (2000). Photodynamic therapy with 5-aminolaevulinic acid or placebo for recalcitrant foot and hand warts: randomised double-blind trial. Lancet 355 (9208), 963–966. doi:10.1016/S0140-6736(00)90013-8
Sun, Y., Ogawa, R., Xiao, B. H., Feng, Y. X., Wu, Y., Chen, L. H., et al. (2020). Antimicrobial photodynamic therapy in skin wound healing: a systematic review of animal studies. Int. Wound J. 17 (2), 285–299. doi:10.1111/iwj.13269
Tegos, G. P., Anbe, M., Yang, C., Demidova, T. N., Satti, M., Mroz, P., et al. (2006). Protease-stable polycationic photosensitizer conjugates between polyethyleneimine and chlorin(e6) for broad-spectrum antimicrobial photoinactivation. Antimicrob. Agents Chemother. 50 (4), 1402–1410. doi:10.1128/AAC.50.4.1402-1410.2006
Vellappally, S., Mahmoud, M. H., Alaqeel, S. M., Alotaibi, R. N., Almansour, H., Alageel, O., et al. (2022). Efficacy of antimicrobial photodynamic therapy versus antiviral therapy in the treatment of herpetic gingivostomatitis among children: aa randomized controlled clinical trial. Photodiagnosis Photodyn. Ther. 39, 102895. doi:10.1016/j.pdpdt.2022.102895
Vicentini, C., Tylcz, J. B., Maire, C., Mordon, S., and Mortier, L. (2017). Terapia fotodinámica. EMC - Dermatol. 51 (3), 1–8. doi:10.1016/s1761-2896(17)85934-3
Wainwright, M. (2003). Local treatment of viral disease using photodynamic therapy. Int. J. Antimicrob. Agents 21 (6), 510–520. doi:10.1016/s0924-8579(03)00035-9
Wainwright, M. (2004). Photoinactivation of viruses. Photochem Photobiol. Sci. 3 (5), 406–411. doi:10.1039/b311903n
Wainwright, M., Maisch, T., Nonell, S., Plaetzer, K., Almeida, A., Tegos, G. P., et al. (2017). Photoantimicrobials-are we afraid of the light? Lancet Infect. Dis. 17 (2), e49–e55. doi:10.1016/S1473-3099(16)30268-7
Westerberg, D. P., and Voyack, M. J. (2013). Onychomycosis: current trends in diagnosis and treatment. Am. Fam. Physician 88 (11), 762–770.
Xie, J., Wang, S., Li, Z., Ao, C., Wang, J., Wang, L., et al. (2019). 5-aminolevulinic acid photodynamic therapy reduces HPV viral load via autophagy and apoptosis by modulating Ras/Raf/MEK/ERK and PI3K/AKT pathways in HeLa cells. J. Photochem Photobiol. B 194, 46–55. doi:10.1016/j.jphotobiol.2019.03.012
Yoon, I., Li, J. Z., and Shim, Y. K. (2013a). Advance in photosensitizers and light delivery for photodynamic therapy. Clin. Endosc. 46 (1), 7–23. doi:10.5946/ce.2013.46.1.7
Yoon, I., Li, J. Z., and Shim, Y. K. (2013b). Advance in photosensitizers and light delivery for photodynamic therapy. Clin. Endosc. 46 (1), 7–23. doi:10.5946/ce.2013.46.1.7
Keywords: photoinactivation, dermatological infections, antimicrobial photodynamic therapy, skin, drug resistance
Citation: Almenara-Blasco M, Pérez-Laguna V, Navarro-Bielsa A, Gracia-Cazaña T and Gilaberte Y (2024) Antimicrobial photodynamic therapy for dermatological infections: current insights and future prospects. Front. Photobiol. 2:1294511. doi: 10.3389/fphbi.2024.1294511
Received: 14 September 2023; Accepted: 04 March 2024;
Published: 05 April 2024.
Edited by:
Indermeet Kohli, Henry Ford Health System, United StatesReviewed by:
Maria Amparo Ferreira Faustino, University of Aveiro, PortugalNatallia E. Uzunbajakava, Netherlands Organisation for Applied Scientific Research, Netherlands
Copyright © 2024 Almenara-Blasco, Pérez-Laguna, Navarro-Bielsa, Gracia-Cazaña and Gilaberte. This is an open-access article distributed under the terms of the Creative Commons Attribution License (CC BY). The use, distribution or reproduction in other forums is permitted, provided the original author(s) and the copyright owner(s) are credited and that the original publication in this journal is cited, in accordance with accepted academic practice. No use, distribution or reproduction is permitted which does not comply with these terms.
*Correspondence: Y. Gilaberte, eWdpbGFiZXJ0ZUBzYWx1ZC5hcmFnb24uZXM=
†These authors have contributed equally to this work