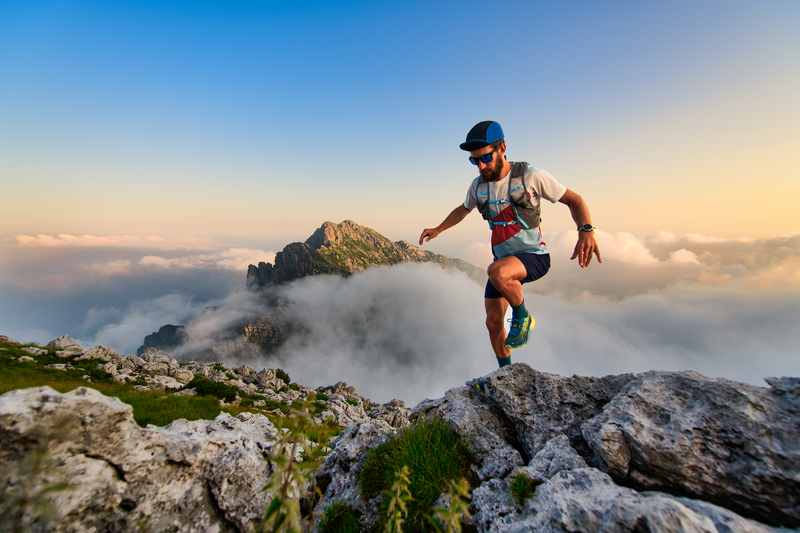
95% of researchers rate our articles as excellent or good
Learn more about the work of our research integrity team to safeguard the quality of each article we publish.
Find out more
ORIGINAL RESEARCH article
Front. Pharmacol. , 01 April 2025
Sec. Inflammation Pharmacology
Volume 16 - 2025 | https://doi.org/10.3389/fphar.2025.1562535
This article is part of the Research Topic Targeting Pulmonary Endothelium in Acute Lung Injury and Acute Respiratory Distress Syndrome View all 7 articles
Lung ischemia-reperfusion (I/R) injury represents an inevitable complication in lung transplantation, characterized by the excessive production of oxygen free radicals and toxic substances. Dexmedetomidine (DEX), a widely used anesthetic agent, has been shown to significantly elevate glutathione (GSH) levels, thereby conferring protection against copper influx. This study investigates the protective mechanisms of DEX in lung I/R injury, with a particular focus on cuproptosis. Utilizing a rat I/R model established by clamping the left hilum of lung for 90 min followed by 120 min of reperfusion, we examined the effects of DEX on lung injury scores, GSH content, and the expression of key proteins involved in cuproptosis. In conclusion, cuproptosis is implicated in pulmonary I/R injury, and the protective effect of DEX against lung I/R injury is partly mediated by inhibition of cuproptosis.
Lung ischemia-reperfusion (I/R) injury represents a critical complication in thoracic surgeries, including lung transplantation and sleeve pulmonary lobectomy. This pathophysiological process is characterized by excessive generation of oxygen free radicals and cytotoxic metabolites (Ward, 1994), frequently resulting in postoperative pulmonary dysfunction and graft failure. Despite its clinical significance, the precise molecular mechanisms underlying lung I/R injury remain incompletely elucidated. Current research priorities focus on mechanistic exploration and therapeutic development to improve surgical outcomes.
Copper, an essential trace element, serves as a catalytic cofactor in redox reactions and regulates multiple enzymatic systems (Steffens et al., 1987; Harris, 1992; Smith-Mungo and Kagan, 1998; Ash et al., 1984). Beyond its established roles in hemoglobin synthesis, bone formation (Rondanelli et al., 2021), and neuroimmune homeostasis (Gromadzka et al., 2020), emerging evidence implicates copper dysregulation in cellular pathology. Mechanistically, impaired copper metabolism induces proteotoxic stress through ferredoxin 1 (FDX1)-mediated lipoylated protein aggregation, mitochondrial respiratory dysfunction, and depletion of iron-sulfur cluster proteins - a copper-dependent cell death pathway termed cuproptosis (Tsvetkov et al., 2022). This novel regulated cell death modality has been implicated in both chronic conditions (e.g., Wilson’s disease) and acute pathologies, including traumatic spinal cord injury (Li et al., 2023).
Dexmedetomidine (DEX), a selective α2-adrenergic receptor agonist with clinical applications in perioperative sedation, has demonstrated protective effects against pulmonary injury. Preclinical studies revealed its capacity to attenuate oxidative damage through ROS suppression and lipid peroxidation reduction (Fu et al., 2017). Specifically, Zhou et al. (Zhou et al., 2018)documented DEX-mediated mitigation of pulmonary I/R injury in rodent models via modulation of oxidative stress markers (MDA reduction and SOD elevation). While these antioxidant properties are well-documented, the molecular mechanisms underlying DEX’s pulmonary protection remain incompletely characterized.
This study investigates the hypothesis that DEX alleviates lung I/R injury through cuproptosis regulation. Utilizing a rat I/R model, we systematically analyze expression profiles of cuproptosis-related proteins and glutathione-mediated antioxidant pathways. Our findings provide novel insights into cuproptosis pathophysiology in pulmonary I/R injury and establish a theoretical framework for developing targeted therapeutic strategies.
Male Sprague-Dawley rats (7–8 weeks old; Pengyue, Jinan, China) were housed in a controlled environment with a 12-h light/dark cycle and ad libitum access to food and water. All animals underwent a 3-day acclimatization period prior to experimentation. Animal procedures were conducted in accordance with the ARRIVE (Animal Research: Reporting of In Vivo Experiments) guidelines and approved by the Institutional Animal Care and Use Committee of the Affiliated Hospital of Qingdao University [AHQU-MAL20230607LH].
Body temperature was maintained at 36.5°C–39.0°C using a heating blanket throughout the procedure. Meloxicam (5 mg/kg) was administered subcutaneously for preemptive analgesia 1 h prior to anesthesia induction. Rats were anesthetized via intraperitoneal injection of sodium pentobarbital (60 mg/kg). Following tracheal intubation through a midline cervical incision, mechanical ventilation (initial tidal volume: 10 mL/kg) was initiated using a rodent ventilator. A femoral venous catheter was placed for drug administration.
After thoracotomy, heparin (300 IU/kg) was intravenously injected. Ten minutes post-heparinization, the left pulmonary hilum was occluded at end-expiration using a non-traumatic microvascular clamp. Lidocaine (20 mg) was topically applied to the surgical site for analgesia and phrenic nerve blockade. During ischemia, tidal volume was reduced to 6 mL/kg. Following 90 min of ischemia, clamps were removed and tidal volume restored to 10 mL/kg for 120-min reperfusion. Post-reperfusion, arterial blood was collected for gas analysis, and euthanasia was performed via exsanguination under deep anesthesia. Left lung tissues (Figure 1) and blood samples were harvested for subsequent analyses.
Figure 1. Photographs of rat lungs. Lungs were obtained immediately on ice blocks at the end of the experiment. (A) lungs in the control group; (B) lungs in the I/R group; (C) lungs in the DEX group. It was visualized that DEX reduced pulmonary congestion after lung ischemia-reperfusion.
Rats were randomly assigned to three groups (n = 6/group): Sham group: Thoracotomy without hilar clamping; I/R group: Full I/R protocol; DEX group: Dexmedetomidine (DEX; Sinopharm Chemical Reagent, 10 μg/kg) administered via femoral vein infusion over 20 min pre-thoracotomy, followed by I/R protocol.
Left lung lobes were fixed in 4% paraformaldehyde for 24 h, paraffin-embedded, and sectioned at 6 μm thickness for hematoxylin-eosin (H&E) staining. Histopathological evaluation was performed by a blinded pathologist using light microscopy. Lung injury scores (LIS) were calculated based on four parameters: (1) neutrophil infiltration, (2) interstitial edema, (3) hyaline membrane formation, and (4) hemorrhage. Each parameter was graded 0–4 (0 = normal, 1 = minimal, 2 = mild, 3 = moderate, 4 = severe).
Surface moisture was carefully blotted from fresh lung tissues using filter paper. Wet weights were recorded before desiccation in a 75°C oven for 72 h. Dry weights were measured to calculate the W/D ratio.
Blood samples were clotted at room temperature for 2 h, then centrifuged (3,000 × g, 15 min, 4°C) to collect serum. TNF-α, IL-1β, and IL-6 levels were quantified using commercial ELISA kits (Invitrogen) following manufacturer protocols.
Lung tissues were homogenized in cold PBS and centrifuged (12,000 × g, 15 min, 4°C). Supernatants were analyzed using GSH assay kits (Elabscience, Wuhan, China). Absorbance at 450 nm was measured for both tissue and serum samples.
Lung tissues were lysed in RIPA buffer (Elabscience, Wuhan, China) supplemented with protease inhibitors, then sonicated (Sonic Dismembrator, United States). For lipoylated protein analysis, 10 μM TCEP was added prior to 95°C denaturation. Protein concentrations were determined via BCA assay (Elabscience), with equal amounts loaded onto 4%–20% gradient SDS-PAGE gels. Proteins were transferred to PVDF membranes and blocked with 5% non-fat milk. Membranes were incubated overnight at 4°C with primary antibodies against: lipoic acid (Abcam, 1:1,000), FDX1 (Abcam, 1:1,000), LIAS (Proteintech, 1:1,000), SDHB (Proteintech, 1:1,000), DLAT (Proteintech, 1:1,000), DLD (Proteintech, 1:1,000), SLC31A1 (MCE, 1:1,000), DLST (MCE, 1:1,000), and β-actin (Sevier, 1:5,000). After PBST washes, membranes were incubated with HRP-conjugated secondary antibodies (Proteintech, 1:10,000) for 1 h at room temperature. Protein bands were visualized using ECL reagent (Elabscience) and quantified with ImageJ software.
Protein samples were prepared with non-reducing loading buffer (Beyotime, China) and minimally heated (70°C, 5 min). Electrophoresis and subsequent procedures followed standard Western blot protocols.
Deparaffinized sections underwent antigen retrieval in citrate buffer (pH 6.0). Endogenous peroxidase was quenched with 3% H2O2, followed by blocking with 10% goat serum. Sections were incubated with anti-lipoic acid antibody (Abcam, 1:100) overnight at 4°C, then with HRP-conjugated secondary antibody (37°C, 1 h). Diaminobenzidine (DAB) was used for chromogenic detection. Hematoxylin staining was used to visualize the nuclei.
After antigen retrieval and blocking, sections were incubated with anti-FDX1 (Abcam, 1:200) and anti-DLAT (Proteintech, 1:500) antibodies overnight at 4°C. Alexa Fluor-conjugated secondary antibodies (Invitrogen, 1:500) were applied for 1 h at room temperature. Nuclei were counterstained with DAPI (5 μg/mL). Images were acquired using an Olympus BX53 fluorescence microscope.
Data are presented as mean ± SD. Normality was assessed using Shapiro-Wilk test. One-way ANOVA with Tukey’s post hoc test was performed using Prism 10.1.2 (GraphPad). Statistical significance was set at p < 0.05.
Histopathological analysis revealed distinct morphological changes among groups (Figures 2A–C). Compared to the sham control, the I/R group demonstrated significantly exacerbated neutrophilic infiltration, interstitial edema, and alveolar hemorrhage. DEX pretreatment markedly improved these pathological alterations. Semi-quantitative assessment of lung injury scores (Figure 2D) confirmed progressive deterioration from control (minimal injury) to I/R groups (severe injury), with DEX intervention showing intermediate protection. The W/D ratio analysis (Figure 2E) paralleled these findings, showing significant elevation in I/R group compared to control, which was effectively reduced by DEX administration (p = 0.0059 vs. I/R).
Figure 2. Comparison of lung tissue injury. (A–C) microscopic images stained with HE. (D) Lung injury score (n = 6). (E) lung tissue dry-wet weight ratio (n = 6). (F) rats’ blood gas parameters (n = 6). ## p < 0.05 vs. Control group; ** p < 0.05 vs. I/R group.
Arterial blood gas analysis (Figure 2F) indicated that lung I/R injury significantly compromised oxygen exchange capacity, as evidenced by decreased PaO2 levels. DEX treatment partially restored oxygenation parameters, showing statistically significant improvement compared to I/R group (p = 0.004).
The I/R procedure induced significant elevation of serum pro-inflammatory cytokines (Figures 3A–C). TNF-α, IL-1β, and IL-6 levels were markedly increased in I/R group versus control (p < 0.05 for all comparisons). DEX pretreatment substantially mitigated this inflammatory cascade, demonstrating significant reduction in all measured cytokines compared to I/R group (p < 0.05).
Figure 3. Inflammatory factors and GSH. (A) concentrations of TNF-α(n = 6). (B) concentrations of IL-1β(n = 6). (C) concentrations of IL-6 (n = 6). (D) concentrations of GSH in serum. (E) the ratio of GSH content in lung tissue. ## p < 0.05 vs. control group; ** p < 0.05 vs. I/R group.
As shown in Figures 3D, E, I/R injury caused significant depletion of GSH content in both lung tissue and serum compared to control group (p < 0.05). DEX administration effectively reversed this trend, restoring GSH levels to values significantly higher than those observed in I/R group (p < 0.05).
Western blot analysis demonstrated I/R-induced upregulation of key cuproptosis regulators including FDX1, SLC31A1, LIAS, DLST, DLD, and SDHB (Figure 4). DEX treatment significantly attenuated these protein level alterations compared to I/R group (p < 0.05). Immunofluorescence quantification confirmed increased FDX1 expression in I/R lungs, which was reduced by DEX intervention (Figure 5).
Figure 4. WB results of cuproptosis-related protein expression. (A) Western blots for FDX 1, SLC 31 A1, LIAS, DLST, DLD, and SDHB in rat lung tissue. (B–G) relative levels of FDX 1, SLC 31 A1, LIAS, DLST, DLD, and SDHB in rat lung tissue (n = 3). ## p < 0.05 vs. control group; ** p < 0.05 vs. I/R group.
Figure 5. Immunofluorescence results of FDX1. Representative images of fluorescence probe for FDX1 and statistical comparison (n = 6). ## p < 0.05 vs. control group; ** p < 0.05 vs. I/R group.
Immunoblot and immunohistochemical analyses revealed significant I/R-induced accumulation of lipoylated DLAT and DLST (Figure 6). DEX administration significantly reduced lipoylated protein levels compared to I/R group (p < 0.05). Non-reducing Western blot demonstrated prominent DLAT oligomerization in I/R group, which was effectively suppressed by DEX treatment (Figure 7). Immunofluorescence analysis corroborated these findings, showing significant reduction of DLAT aggregates with DEX intervention (Figure 8).
Figure 6. Immunohistochemical and WB results of lipoacylated proteins. (A–C) representative images of lipoacylated protein immunohistochemical staining in rat lung tissue. (D) Western blots for lip-DLAT and lip-DLST. (E, F) relative levels of lip-DLAT and lip-DLST in rats lung tissue (n = 3). ## p < 0.05 vs. control group; ** p < 0.05 vs. I/R group.
Figure 7. Immunofluorescence results of DLAT. Representative images of fluorescence probe for DLAT and statistical comparison (n = 6). ## p < 0.05 vs. control group; ** p < 0.05 vs. I/R group.
Figure 8. Oligomerization of the DLAT protein in WB experiment. DLAT protein oligomerization was observed in the I/R group, but not in the other two groups.
Our study demonstrates that dexmedetomidine (DEX) administration effectively attenuates lung ischemia-reperfusion (I/R) injury, and this process is accompanied by inhibition of the cuproptosis. The therapeutic effects manifested as improved oxygenation parameters, reduced histopathological damage, and suppression of pro-inflammatory cytokine release.
Copper homeostasis plays pivotal roles in physiological processes, requiring precise regulation through an integrated network of copper-dependent proteins including transporters, chaperones, and enzymes (Zhong et al., 2022; Cobine and Brady, 2022; Bharathi Devi et al., 2016). Central to this regulatory system is solute carrier family 31 member 1 (SLC31A1), the principal copper importer governing cellular copper influx (Qi et al., 2023; Das et al., 2022; Xie et al., 2023). Our findings reveal that DEX significantly downregulates SLC31A1 expression, suggesting direct interference with copper uptake mechanisms. This observation aligns with computational modeling data indicating potential molecular interactions between DEX and SLC31A1, though experimental validation of this binding specificity requires further investigation.
The antioxidant glutathione (GSH) emerged as another critical mediator in DEX’s protective mechanism. We observed that DEX administration counteracts I/R-induced GSH depletion in both pulmonary tissue and systemic circulation. Given GSH’s established role in copper chelation and detoxification (Saporito-Magriñá et al., 2018), this restoration likely disrupts copper-mediated oxidative cascades. Notably, the coordinated downregulation of SLC31A1 and upregulation of GSH suggests synergistic modulation of copper homeostasis, potentially through adrenergic receptor-dependent signaling pathways warranting further exploration.
Ferredoxin 1 (FDX1), a key reductase facilitating copper redox cycling and protein lipoylation (Tsvetkov et al., 2022), displayed marked overexpression in I/R lungs that was attenuated by DEX treatment. The FDX1 suppression correlated with reduced lipoylated protein accumulation (DLAT/DLST) and diminished DLAT oligomerization - hallmark features of cuproptosis. FDX one results in oxidative stress inside the cell. Oxygen free radicals have been demonstrated to significantly contribute to cell death in organ I/R (Sadrkhanloo et al., 2022). Mechanistically, FDX1 inhibition may preserve cellular redox balance by maintaining GSH/GSSG ratios critical for free radical scavenging (Chen et al., 2022; Poprac et al., 2017). This dual regulation of copper metabolism and oxidative stress pathways positions FDX1 as a crucial nexus in DEX-mediated protection against I/R injury. DEX significantly inhibited FDX1/SLC31A1 expression, reduced copper influx, and restored TCA cycle function. This process may regulate protein post-translational modification through the α2-adrenoceptor mediated PI3K/Akt/mTOR signaling pathway (Zhao et al., 2020; Huang et al., 2024).
Our study has some limitations. First, the absence of cellular models limits mechanistic depth. Genetic manipulation (FDX1 knockout/overexpression) in vitro could clarify its regulatory hierarchy and enable mitochondrial functional analyses. Second, the lack of established cuproptosis inhibitors as positive controls prevents direct comparison of DEX’s therapeutic efficacy. Future studies should incorporate pharmacological (e.g., tetrathiomolybdate) and genetic interventions to validate target specificity.
There is a cuproptosis mechanism in the process of lung ischemia-reperfusion. The protective effect of DEX against lung I/R injury is partly mediated by inhibition of cuproptosis.
The original contributions presented in the study are included in the article/Supplementary Material, further inquiries can be directed to the corresponding author.
The animal study was approved by Ethics Committee of Laboratory Animal Welfare, Affiliated Hospital of Qingdao University. The study was conducted in accordance with the local legislation and institutional requirements.
HL: Conceptualization, Data curation, Formal Analysis, Investigation, Methodology, Project administration, Resources, Software, Supervision, Validation, Visualization, Writing – original draft, Writing – review and editing. ZW: Data curation, Formal Analysis, Writing – review and editing. TQ: Funding acquisition, Investigation, Methodology, Project administration, Resources, Writing – review and editing. WD: Data curation, Methodology, Project administration, Resources, Supervision, Validation, Writing – review and editing. ZW: Data curation, Methodology, Supervision, Validation, Visualization, Writing – review and editing. SC: Methodology, Project administration, Validation, Writing – review and editing. WJ: Conceptualization, Funding acquisition, Investigation, Methodology, Project administration, Resources, Software, Validation, Visualization, Writing – review and editing.
The author(s) declare that financial support was received for the research and/or publication of this article. This study received financial support from National Natural Science Foundation of China, Natural Science Foundation of Shandong Province and Postdoctoral Innovation Project of Shandong Province.
The authors declare that the research was conducted in the absence of any commercial or financial relationships that could be construed as a potential conflict of interest.
The author(s) declare that no Generative AI was used in the creation of this manuscript.
All claims expressed in this article are solely those of the authors and do not necessarily represent those of their affiliated organizations, or those of the publisher, the editors and the reviewers. Any product that may be evaluated in this article, or claim that may be made by its manufacturer, is not guaranteed or endorsed by the publisher.
The Supplementary Material for this article can be found online at: https://www.frontiersin.org/articles/10.3389/fphar.2025.1562535/full#supplementary-material
Ash, D. E., Papadopoulos, N. J., Colombo, G., and Villafranca, J. J. (1984). Kinetic and spectroscopic studies of the interaction of copper with dopamine beta-hydroxylase. J. Biol. Chem. 259, 3395–3398. doi:10.1016/s0021-9258(17)43105-x
Bharathi Devi, S. R., Dhivya, M. A., and Sulochana, K. N. (2016). Copper transporters and chaperones: their function on angiogenesis and cellular signalling. J. Biosci. 41, 487–496. doi:10.1007/s12038-016-9629-6
Chen, G. H., Song, C. C., Pantopoulos, K., Wei, X. L., Zheng, H., and Luo, Z. (2022). Mitochondrial oxidative stress mediated Fe-induced ferroptosis via the NRF2-ARE pathway. Free Radic. Biol. and Med. 180, 95–107. doi:10.1016/j.freeradbiomed.2022.01.012
Cobine, P. A., and Brady, D. C. (2022). Cuproptosis: cellular and molecular mechanisms underlying copper-induced cell death. Mol. cell 82, 1786–1787. doi:10.1016/j.molcel.2022.05.001
Das, A., Ash, D., Fouda, A. Y., Sudhahar, V., Kim, Y. M., Hou, Y., et al. (2022). Cysteine oxidation of copper transporter CTR1 drives VEGFR2 signalling and angiogenesis. Nat. cell Biol. 24, 35–50. doi:10.1038/s41556-021-00822-7
Fu, C., Dai, X., Yang, Y., Lin, M., Cai, Y., and Cai, S. (2017). Dexmedetomidine attenuates lipopolysaccharide-induced acute lung injury by inhibiting oxidative stress, mitochondrial dysfunction and apoptosis in rats. Mol. Med. Rep. 15, 131–138. doi:10.3892/mmr.2016.6012
Gromadzka, G., Tarnacka, B., Flaga, A., and Adamczyk, A. (2020). Copper dyshomeostasis in neurodegenerative diseases-therapeutic implications. Int. J. Mol. Sci. 21, 9259. doi:10.3390/ijms21239259
Harris, E. D. (1992). Copper as a cofactor and regulator of copper,zinc superoxide dismutase. J. Nutr. 122, 636–640. doi:10.1093/jn/122.suppl_3.636
Huang, T., Zhang, Y., Wu, Y., Han, X., Li, L., Guo, Z., et al. (2024). CEBPB dampens the cuproptosis sensitivity of colorectal cancer cells by facilitating the PI3K/AKT/mTOR signaling pathway. Saudi J. gastroenterology official J. Saudi Gastroenterology Assoc. 30, 381–388. doi:10.4103/sjg.sjg_169_24
Li, C., Wu, C., Ji, C., Xu, G., Chen, J., Zhang, J., et al. (2023). The pathogenesis of DLD-mediated cuproptosis induced spinal cord injury and its regulation on immune microenvironment. Front. Cell. Neurosci. 17, 1132015. doi:10.3389/fncel.2023.1132015
Poprac, P., Jomova, K., Simunkova, M., Kollar, V., Rhodes, C. J., and Valko, M. (2017). Targeting free radicals in oxidative stress-related human diseases. Trends Pharmacol. Sci. 38, 592–607. doi:10.1016/j.tips.2017.04.005
Qi, Y., Yao, Q., Li, X., Li, X., Zhang, W., and Qu, P. (2023). Cuproptosis-related gene SLC31A1: prognosis values and potential biological functions in cancer. Sci. Rep. 13, 17790. doi:10.1038/s41598-023-44681-8
Rondanelli, M., Faliva, M. A., Infantino, V., Gasparri, C., Iannello, G., Perna, S., et al. (2021). Copper as dietary supplement for bone metabolism: a review. Nutrients 13, 2246. doi:10.3390/nu13072246
Sadrkhanloo, M., Entezari, M., Orouei, S., Zabolian, A., Mirzaie, A., Maghsoudloo, A., et al. (2022). Targeting Nrf2 in ischemia-reperfusion alleviation: from signaling networks to therapeutic targeting. Life Sci. 300, 120561. doi:10.1016/j.lfs.2022.120561
Saporito-Magriñá, C. M., Musacco-Sebio, R. N., Andrieux, G., Kook, L., Orrego, M. T., Tuttolomondo, M. V., et al. (2018). Copper-induced cell death and the protective role of glutathione: the implication of impaired protein folding rather than oxidative stress. Metallomics 10, 1743–1754. doi:10.1039/c8mt00182k
Smith-Mungo, L. I., and Kagan, H. M. (1998). Lysyl oxidase: properties, regulation and multiple functions in biology. J. Int. Soc. Matrix Biol. 16, 387–398. doi:10.1016/s0945-053x(98)90012-9
Steffens, G. C., Biewald, R., and Buse, G. (1987). Cytochrome c oxidase is a three-copper, two-heme-A protein. Eur. J. Biochem. 164, 295–300. doi:10.1111/j.1432-1033.1987.tb11057.x
Tsvetkov, P., Coy, S., Petrova, B., Dreishpoon, M., Verma, A., Abdusamad, M., et al. (2022). Copper induces cell death by targeting lipoylated TCA cycle proteins. Science 375, 1254–1261. doi:10.1126/science.abf0529
Ward, P. A. (1994). Oxygen radicals, cytokines, adhesion molecules, and lung injury. Environ. health Perspect. 102 (Suppl. 10), 13–16. doi:10.1289/ehp.94102s1013
Xie, J., Yang, Y., Gao, Y., and He, J. (2023). Cuproptosis: mechanisms and links with cancers. Mol. cancer 22, 46. doi:10.1186/s12943-023-01732-y
Zhao, Y., Feng, X., Li, B., Sha, J., Wang, C., Yang, T., et al. (2020). Dexmedetomidine protects against lipopolysaccharide-induced acute kidney injury by enhancing autophagy through inhibition of the PI3K/AKT/mTOR pathway. Front. Pharmacol. 11, 128. doi:10.3389/fphar.2020.00128
Zhong, C. C., Zhao, T., Hogstrand, C., Chen, F., Song, C. C., and Luo, Z. (2022). Copper (Cu) induced changes of lipid metabolism through oxidative stress-mediated autophagy and Nrf2/PPARγ pathways. J. Nutr. Biochem. 100, 108883. doi:10.1016/j.jnutbio.2021.108883
Keywords: lung ischemia-reperfusion injury, dexmedetomidine, cuproptosis, rats, in vitro experiments
Citation: Liu H, Wang Z, Qiu T, Du W, Wu Z, Chen S and Jiao W (2025) Dexmedetomidine alleviates lung ischemia-reperfusion injury by inhibiting cuproptosis: an in vivo study. Front. Pharmacol. 16:1562535. doi: 10.3389/fphar.2025.1562535
Received: 17 January 2025; Accepted: 24 March 2025;
Published: 01 April 2025.
Edited by:
You Shang, Huazhong University of Science and Technology, ChinaReviewed by:
Shihua Lv, The Second Affiliated Hospital of Harbin Medical University, ChinaCopyright © 2025 Liu, Wang, Qiu, Du, Wu, Chen and Jiao. This is an open-access article distributed under the terms of the Creative Commons Attribution License (CC BY). The use, distribution or reproduction in other forums is permitted, provided the original author(s) and the copyright owner(s) are credited and that the original publication in this journal is cited, in accordance with accepted academic practice. No use, distribution or reproduction is permitted which does not comply with these terms.
*Correspondence: Wenjie Jiao, amlhb3dqQHFkdWhvc3BpdGFsLmNu
Disclaimer: All claims expressed in this article are solely those of the authors and do not necessarily represent those of their affiliated organizations, or those of the publisher, the editors and the reviewers. Any product that may be evaluated in this article or claim that may be made by its manufacturer is not guaranteed or endorsed by the publisher.
Research integrity at Frontiers
Learn more about the work of our research integrity team to safeguard the quality of each article we publish.