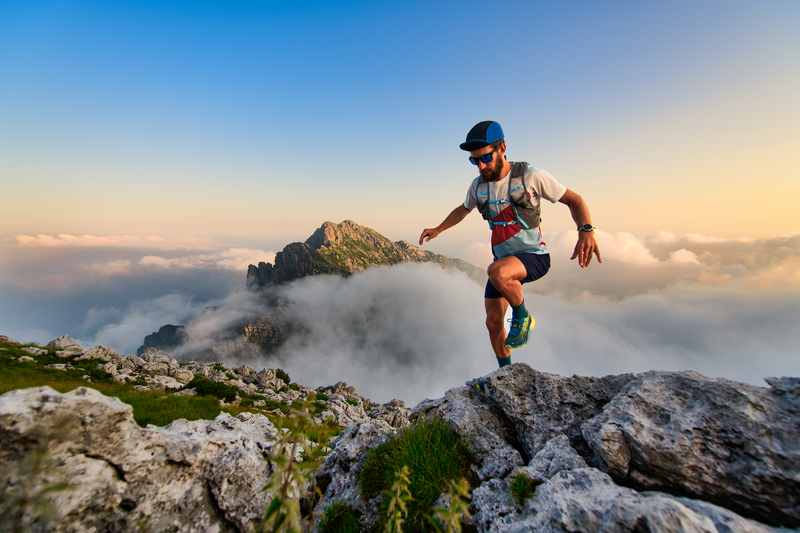
95% of researchers rate our articles as excellent or good
Learn more about the work of our research integrity team to safeguard the quality of each article we publish.
Find out more
REVIEW article
Front. Pharmacol. , 20 March 2025
Sec. Cardiovascular and Smooth Muscle Pharmacology
Volume 16 - 2025 | https://doi.org/10.3389/fphar.2025.1493662
Aging is an independent risk factor for many chronic diseases, including cancer and cardiovascular, pulmonary, and neurodegenerative diseases. In recent years, the mechanisms of aging-related cardiovascular diseases (CVDs) have been studied intensively. Takeda G protein-coupled receptor 5 (TGR5) is a membrane receptor for bile acids that has been found to play an important role in various disease processes, such as inflammation, oxidative stress, and metabolic disorders, all of which contribute to aging-related CVDs. In this review, we summarise the role of TGR5 in aging-related CVDs and propose TGR5 as an attractive therapeutic target based on its mechanism of involvement, which may contribute to future drug target design.
With the progressive aging of the global population, the incidence of aging-related CVDs has been on the rise (Sheikh and Salma, 2024). Despite much work has been done to ameliorate or prevent age-related physiological decline and to select appropriate therapeutic agents to maximize healthy lifespan, effective clinical interventions to mitigate the impact of aging on human health remain limited (Queen et al., 2020; Hodgson et al., 2020; Lacanna et al., 2019; Ali et al., 2020). Therefore, it is imperative that we explore therapeutic approaches to promote healthy aging.
One promising therapeutic target that has gained attention in the context of aging is Takeda G protein-coupled receptor 5 (TGR5). It plays a crucial role in the regulation of metabolic processes such as glucose and lipid metabolism, as well as modulating immune-inflammatory responses and maintaining energy homeostasis (Jin et al., 2024). According to previous researches, TGR5 has been reported to have a significant impact on the progression of tumors and the development of metabolic disorders such as diabetes and obesity (Jin et al., 2024; Lun et al., 2024; Zhao et al., 2022; Bhimanwar and Mittal, 2022). More recently, emerging evidence has suggested that TGR5 may also play a pivotal role in the aging process (Wang et al., 2023; Perino et al., 2021). In this review, we aim to summarise the role of TGR5 in aging-related cardiovascular diseases and to explore the therapeutic potential of TGR5 for targeting these diseases. By understanding the intricate interplay between TGR5 and the various pathways involved in cardiovascular aging, we hope to pave the way for the development of therapeutic strategies to combat aging-related CVDs.
TGR5, a member of the G protein-coupled receptor (GPCR) superfamily, is characterized by seven transmembrane domains that enable it to recognize and bind various bile acid molecules, including deoxycholic acid (DCA) and lithocholic acid (LCA) (Lun et al., 2024). Upon activation, TGR5 primarily increases intracellular cAMP levels through the stimulation of Gs protein (Tanaka et al., 2024). As a stimulatory G protein, Gs interacts with the activated TGR5, leading to the activation of adenylate cyclase (AC). This subsequently promotes the conversion of ATP into cAMP. This process constitutes a crucial step in the TGR5 signaling pathway, as cAMP functions as a second messenger to further activate downstream signaling molecules, including protein kinase A (PKA), thereby regulating a wide range of cellular physiological functions (Holter et al., 2020). These signaling events subsequently exert influences on the physiological functions of cells.
TGR5 is widely expressed across various tissues and organs, reflecting its diverse physiological roles. High levels of TGR5 expression have been observed in the liver, intestine, kidneys, and cardiovascular system (Gou et al., 2023). In the liver, TGR5 is expressed on the surface of hepatocytes and cholangiocytes, playing a pivotal role in bile acid homeostasis and liver protection (Bidault-Jourdainne et al., 2021). By sensing changes in bile acid levels, TGR5 regulates bile acid synthesis and transport, thereby preventing the accumulation of toxic bile acids and protecting the liver from injury (Chiang and Ferrell, 2020). In the intestine, TGR5 is expressed on the surface of enterocytes, where it promotes the absorption of bile acids and fatty acids and regulates intestinal motility (Chang et al., 2024). Through facilitating nutrient absorption and modulating gut function, TGR5 contributes to overall metabolic health. In the kidneys, TGR5 is expressed on the surface of renal tubular cells (Guo et al., 2024). By modulating renal function, TGR5 helps prevent the progression of kidney diseases, such as nephropathy (Kim et al., 2023). Finally, in the cardiovascular system, TGR5 is expressed on cardiomyocytes, endothelial cells, and smooth muscle cells, where it regulates cardiac function, vascular tone, and inflammation (Wang et al., 2021; Sauerbruch et al., 2021).
Atherosclerosis is a typical aging-related chronic CVD that poses a significant threat to human health and is one of the leading causes of death among the elderly (Kong et al., 2022). As individuals age, metabolic and immune functions decline, increasing the risk of atherosclerosis. Research has shown that TGR5 expression levels significantly decrease in aging mouse models, whereas TGR5 activation significantly improves aging-related cardiovascular dysfunction (Wang et al., 2017). In animal models, TGR5 activation has significantly reduced plasma total cholesterol and triglyceride levels, as well as atherosclerotic lesion areas in the aortic root, underscoring its crucial role in regulating lipid metabolism (Biagioli et al., 2023). Additionally, in macrophages, TGR5 activation downregulates the expression of scavenger receptor A (SR-A) and cluster of differentiation 36 (CD36), which are pivotal in the uptake of oxidized LDL (oxLDL) and foam cell formation (Xia et al., 2020). Consequently, TGR5 activation reduces the uptake of oxLDL by macrophages, inhibiting foam cell formation and mitigating the lipid deposition process in atherosclerosis. These suggest that TGR5 plays a pivotal role in regulating various physiological and pathophysiological processes, exhibiting remarkable protective effects against the development of atherosclerosis.
Given TGR5’s multifaceted protective roles in atherosclerosis, its potential as a therapeutic target is gaining increasing attention. Various TGR5 agonists have been developed and demonstrated promising anti-atherosclerotic effects in animal models. For instance, INT-777 significantly inhibits the development of atherosclerosis in mouse models, reducing macrophage content within plaques (Miyazaki-Anzai et al., 2018). Additionally, the application of nanotechnology has enhanced the bioavailability and targeting of TGR5 agonists, laying the groundwork for future clinical trials (Moretti et al., 2018). Figure 1 shows the role of TGR5 in atherosclerosis. However, therapeutic strategies targeting TGR5 still face significant challenges. For instance, systemic activation of TGR5 agonists may disrupt bile acid homeostasis, potentially inducing biliary dysfunction or gastrointestinal adverse effects (Fleishman and Kumar, 2024; Ye et al., 2024). Additionally, the extent of TGR5 signaling activation may vary due to individual genetic backgrounds (such as GPBAR1 polymorphisms) or differences in gut microbiota composition, leading to inconsistent therapeutic responses (Zhao et al., 2023; Pavlovic et al., 2017). The dose-dependent effects observed in preclinical studies also suggest that careful optimization of dosing regimens in human trials is necessary to avoid desensitization or receptor downregulation risks (Van Nierop et al., 2017).
Figure 1. TGR5 in atherosclerosis. After using TGR5 receptor agonists (such as INT-777) treated cells, macrophages reduce the production of circulating factors and uptake of oxidized LDL via TGR5-induced cAMP signaling pathway and subsequent NF-κB inhibition, which may contribute to its anti-atherosclerotic effect.
MI is predominantly observed in the elders. However, dysregulation of immune pathways, impaired suppression of post-MI inflammation, spatially restricted inflammatory responses, and excessive fibrosis can lead to adverse cardiac remodeling, significantly impairing cardiac function. In recent years, the role of bile acids and their receptors in CVDs has garnered increasing attention. A study investigating changes in plasma bile acid levels in patients with acute MI found significantly reduced levels of DCA (Wang et al., 2021). This observation led researchers to investigate the role of DCA and its receptor TGR5 in the context of MI. In a mouse model of MI induced by ligation of the left anterior descending coronary artery, subsequent studies demonstrated that DCA binding to TGR5 significantly improved cardiac function and reduced ischemic injury (Wang et al., 2021). These findings highlight the protective role of DCA in MI and suggest that TGR5 could be a promising therapeutic target. Additionally, DCA was found to suppress the expression and activation of IL-1β, thereby mitigating inflammation-induced myocardial damage (Forteza et al., 2023).
To further explore the role of TGR5 in MI, researchers have conducted investigations into its impact on the immune system. In TGR5−/− mice, the proportions of CD4+ T and CD8+ T cells in the heart and spleen remained elevated, with significantly increased CD4+ T cell infiltration in ischemic areas (Cheng et al., 2022). This suggests that TGR5 may regulate CD4+ T cell function post-MI. To test this hypothesis, researchers performed MI surgeries in CD4-specific TGR5-knockout mice. Compared to controls, these mice exhibited more severe ischemic injury, significantly reduced cardiac function, and larger infarct areas, further confirming TGR5’s critical role in post-MI cardiac repair and remodeling (Cheng et al., 2022).
At the cellular level, additional studies have shed light on TGR5’s mechanisms of action. In rat H9C2 cardiomyocytes and human cardiomyocytes subjected to ischemia/reperfusion injury, TGR5 expression was significantly upregulated (Li et al., 2020). This upregulation is likely an adaptive response to ischemic injury, aiming to mitigate damage through TGR5 activation. Further experiments revealed that TGR5 activation improved cell proliferation, reduced apoptosis, and decreased the mRNA and protein levels of inflammatory factors, suggesting that TGR5 confers cardioprotective effects by suppressing inflammation and enhancing cell survival (Li et al., 2020).
Notably, the pro-survival effects of TGR5 activation may entail metabolic trade-offs (Su et al., 2024; Guo et al., 2016). Enhanced cAMP signaling could exacerbate arrhythmogenic risks, particularly in aged hearts with preexisting calcium dysregulation (Grammatika Pavlidou et al., 2023; Lin et al., 2018). Interestingly, the protective effects of TGR5 were partially reversed when cells were treated with the AKT inhibitor MK-2206 (Li et al., 2020). This finding indicates that TGR5 may exert its protective functions through the AKT/GSK-3β signaling pathway. Given the pivotal role of the AKT/GSK-3β pathway in cell survival, proliferation, and apoptosis, TGR5 likely modulates this pathway to safeguard cardiomyocytes from ischemic injury. Additionally, the dual role of TGR5 in post-ischemic inflammation resolution and fibrotic repair remains incompletely understood, necessitating time-dependent modulation to avoid maladaptive remodeling (Wang et al., 2022; Kaya et al., 2019).
HF is a terminal stage of various CVDs, is characterized by diminished cardiac pump function. Recent studies have revealed a close relationship between TGR5 and aging-related HF. Changes in TGR5 expression and function in elderly HF patients may influence cardiac function and repair processes (Xu et al., 2024). MI is a major trigger for HF, as myocardial necrosis and scar formation after MI lead to structural and functional alterations in the heart, predisposing it to HF (Jiang et al., 2023). TGR5 likely plays a pivotal role in this transition by promoting myocardial repair and regeneration, reducing inflammation, and improving cardiac function, thereby preventing HF progression (Yan et al., 2024).
In the elderly population, cardiac hypertrophy is often pathological and closely related to cardiac dysfunction, significantly impacting their quality of life and overall health status (Tracy et al., 2020). Multiple studies have confirmed that the activation of TGR5 can inhibit the onset and progression of cardiac hypertrophy. In an H9C2 cardiac hypertrophy model induced by high glucose, LCA reduces the mRNA levels of hypertrophic markers such as calcineurin (CaN) and nuclear factor of activated T-cells (NFAT) in a dose-dependent manner (Cheng et al., 2019). Furthermore, upon activation of TGR5 by LCA, cAMP levels rise, subsequently activating PKA and increasing the phosphorylation of phospholamban (PLN) (Cheng et al., 2019). This process reverses the downregulation of SERCA2a expression, which is a crucial step in improving cardiac function. These findings suggest that TGR5 activation modulates intracellular calcium homeostasis and inhibits the CaN/NFAT signaling pathway, thereby reducing calcium ion accumulation in cardiomyocytes and promoting myocardial energy metabolism, ultimately inhibiting cardiomyocyte hypertrophy.
Additionally, in a myocardial hypertrophy model induced by endothelin-1 (ET-1), TGR5 activation significantly suppressed the increase in cardiomyocyte surface area and total protein, as well as the expression of hypertrophic markers such as atrial natriuretic peptide (ANF) and β-myosin heavy chain (β-MHC) (Chi et al., 2024; Jen et al., 2017; Wang et al., 2024). TGR5 activation may also protect cardiomyocytes from damage by inhibiting inflammation and oxidative stress, further inhibiting cardiac hypertrophy (Xu et al., 2024; Cheng et al., 2019).
In a DCM model, TGR5 plays a regulatory role in cardiac hypertrophy. Cardiac-specific TGR5−/− mice exhibit more severe cardiac dysfunction and myocardial hypertrophy, while TGR5 activation can prevent lipotoxicity and cardiac dysfunction, inhibiting cardiac hypertrophy (Fleishman and Kumar, 2024; Wang et al., 2024). This may be related to TGR5’s inhibition of fatty acid uptake and the localization of CD36 in the plasma membrane, as CD36 is the main mediator of long-chain fatty acid uptake. By inhibiting CD36-mediated fatty acid uptake and lipotoxicity, the energy metabolism and inflammatory responses of cardiac cells are improved, thereby slowing or reversing the development of cardiac hypertrophy (Shu et al., 2022).
During the aging process, the risk of cardiac fibrosis significantly increases (Izzo et al., 2021). Cardiac fibrosis serves as a common pathological basis for various CVDs, including HF and MI, and is also a critical factor contributing to the gradual decline of cardiac function (Jiang et al., 2021).
Emerging evidence suggests that the activation of TGR5 may inhibit the onset and progression of cardiac fibrosis through multiple pathways. With advancing age, the body’s energy metabolism and lipid metabolism functions gradually decline, resulting in abnormal lipid deposition in tissues such as the heart (Xie et al., 2023). However, the activation of TGR5 can promote energy expenditure and lipid metabolism, reducing lipid accumulation in cardiac tissue and thereby effectively inhibiting the occurrence of cardiac fibrosis (Wang et al., 2024). The activation of TGR5, on the other hand, can protect cardiac tissue from injury by inhibiting inflammatory responses and oxidative stress pathways (Doroszko et al., 2020). In animal models, it has been observed that TGR5 activation reduces the expression of inflammatory factors and the generation of oxidative stress products in myocardial tissue, thereby inhibiting the progression of cardiac fibrosis (Xu et al., 2024; Yin et al., 2023). The activation of TGR5 may regulate the expression of genes related to extracellular matrix (ECM) synthesis and degradation, suppressing the activation and proliferation of cardiac fibroblasts, and thus reducing collagen fiber deposition (Yin et al., 2023). For example, by inhibiting the expression of fibrosis factors such as transforming growth factor-β (TGF-β), TGR5 can decrease the activation of cardiac fibroblasts and the synthesis of ECM (Reilly-O'Donnell et al., 2024; Sweeney et al., 2020). In a mouse model of myocardial fibrosis induced by a combination of isoproterenol and ursodeoxycholic acid (UDCA), research has found that UDCA, through the activation of TGR5, significantly alleviated the degree of myocardial fibrosis and reduced the expression of type I and III collagen, as well as TGF-β1 protein (Reilly-O'Donnell et al., 2024; Li et al., 2017). This finding further supports the potential role of TGR5 in cardiac fibrosis.
Hypertension, as a chronic disease that is widespread globally, has a significantly higher prevalence with age, posing a serious threat to public health (Mills et al., 2020). During the aging process, the activation of TGR5 stimulates energy expenditure and lipid metabolism, reducing the accumulation of lipids in vital tissues such as the heart and blood vessels, thereby lowering the risk of hypertension (Wang et al., 2023). Furthermore, TGR5 can inhibit the expression of the epithelial sodium channel (ENaC) in the kidney, decreasing sodium reabsorption and subsequently lowering blood pressure (Xu et al., 2025). This mechanism has been validated in mouse models treated with deoxycorticosterone acetate (DOCA), highlighting the significant role of TGR5 in renal sodium handling (Xu et al., 2025).
Beyond its direct effects on the kidney, TGR5 also participates in blood pressure regulation by influencing water and electrolyte balance. Studies have shown that TGR5 activation can modulate the expression and trafficking of AQP2 (aquaporin-2) in renal medullary collecting duct cells via the cAMP pathway, thereby affecting water reabsorption and urine concentration, and indirectly regulating blood pressure (Guo et al., 2024). Additionally, TGR5 activation induces the expression of eNOS and the production of NO, while promoting the generation of hydrogen sulfide and inhibiting endothelin signaling (Ma et al., 2021; Fiorucci et al., 2017). These effects collectively promote vasodilation and reduce blood pressure. Nevertheless, TGR5-mediated regulation of renal ENaC and AQP2 may be attenuated by age-related tubular dysfunction, contributing to interindividual variability in antihypertensive efficacy (Tamma et al., 2015; Tiwari et al., 2009). Moreover, central TGR5 activation, which suppresses sympathetic activity, may unpredictably interact with conventional therapies (such as β-blockers), warranting pharmacokinetic studies to elucidate synergistic or antagonistic mechanisms.
Notably, the activation of TGR5 can significantly ameliorate hypertension symptoms, which is associated with decreased levels of norepinephrine, improved myocardial cell morphology, and reduced inflammation and oxidative stress in the paraventricular nucleus (PVN) (Li et al., 2024). This finding suggests that TGR5 may serve as a central regulator of blood pressure, modulating sympathetic nerve activity and cardiovascular function.
Moreover, the gut microbiota plays a pivotal role in blood pressure regulation, and changes in its composition are closely linked to hypertension (Ge et al., 2024). TGR5 is closely related to gut microbiota function, as evidenced by the significant remodeling of the gut microbiota observed in TGR5−/− mice (Zhan et al., 2024; Ma et al., 2020). This further confirms the role of TGR5 in gut microbiota regulation. Bile acids, as ligands of TGR5, not only activate TGR5 but also regulate the composition and function of the gut microbiota, forming a potential feedback loop for blood pressure regulation (Ishimwe et al., 2022). Table 1 briefly summarizes the role of TGR5 and aging-related CVDs.
In summary, this review highlights the emerging role of TGR5 as a potential therapeutic target for aging-related CVDs.By extensively examining the literature, we found that TGR5, a bile acid receptor, plays a crucial role in regulating these mechanisms. These effects are particularly relevant in the context of aging-related CVDs such as atherosclerosis, MI, HF, cardiac hypertrophy, myocardial fibrosis, and hypertension.
Although current evidence suggests that TGR5 activation may confer cardioprotective benefits, the underlying mechanisms remain to be fully elucidated. The current research on TGR5 is limited by several critical gaps. First, the lack of clinical data on safety and efficacy in humans represents a significant barrier to progress. Second, the complexity of TGR5 signaling networks, including its crosstalk with other bile acid receptors such as FXR and PXR, may obscure target-specific effects and complicate therapeutic outcomes. Third, the impact of aging-related microenvironmental changes, including epigenetic modifications and senescence-associated secretory phenotypes, on TGR5 functionality remains poorly understood. Future research should prioritize the development of tissue-selective TGR5 modulators to minimize off-target effects, employ advanced models such as organoids or humanized animals to dissect individual response heterogeneity, and explore potential synergies between TGR5 agonists and existing anti-aging therapies, such as Senolytics, to enhance therapeutic efficacy (Zhang et al., 2022). Additionally, longitudinal studies are needed to assess the long-term safety and tolerability of TGR5-targeted interventions, particularly in elderly populations with comorbidities. Overall, this review underscores the importance of TGR5 in the pathogenesis of aging-related CVDs and highlights its potential as an attractive therapeutic target for these increasingly prevalent conditions.
YH: Conceptualization, Formal Analysis, Writing–original draft, Writing–review and editing. SL: Funding acquisition, Investigation, Resources, Writing–review and editing. YaZ: . YuZ: Formal Analysis, Software, Writing–review and editing. KH: Conceptualization, Validation, Writing–review and editing. LD: Conceptualization, Methodology, Project administration, Writing–review and editing. BL: Funding acquisition, Supervision, Validation, Writing–review and editing. YiZ: Conceptualization, Project administration, Supervision, Writing–review and editing. JF: Conceptualization, Funding acquisition, Supervision, Validation, Writing–review and editing.
The author(s) declare that financial support was received for the research and/or publication of this article. This research was funded by grants from Sichuan Science and Technology Program (2022YFS0610), Luzhou Municipal People’s Government - Southwest Medical University Science and Technology Strategic Cooperation (2021LZXNYD-J33), Hejiang People’s Hospital - Southwest Medical University Science and Technology Strategic Cooperation Project (2021HJXNYD13), Xuyong People’s Hospital - Southwest Medical University Science and Technology Strategic Cooperation Project (2024XYXNYD18), Gulin County People’s Hospital - Affiliated Hospital of Southwest Medical University Science and Technology strategic Cooperation (2022GLXNYDFY13), 2022-N-01-33 project of China International Medical Foundation.
The authors declare that the research was conducted in the absence of any commercial or financial relationships that could be construed as a potential conflict of interest.
All claims expressed in this article are solely those of the authors and do not necessarily represent those of their affiliated organizations, or those of the publisher, the editors and the reviewers. Any product that may be evaluated in this article, or claim that may be made by its manufacturer, is not guaranteed or endorsed by the publisher.
Ali, S., Mansour, A. G., Huang, W., Queen, N. J., Mo, X., Anderson, J. M., et al. (2020). CSF1R inhibitor PLX5622 and environmental enrichment additively improve metabolic outcomes in middle-aged female mice. Aging (Albany NY) 12, 2101–2122. doi:10.18632/aging.102724
Bhimanwar, R. S., and Mittal, A. (2022). TGR5 agonists for diabetes treatment: a patent review and clinical advancements (2012-present). Expert Opin. Ther. Pat. 32, 191–209. doi:10.1080/13543776.2022.1994551
Biagioli, M., Marchiano, S., Di Giorgio, C., Bordoni, M., Urbani, G., Bellini, R., et al. (2023). Activation of GPBAR1 attenuates vascular inflammation and atherosclerosis in a mouse model of NAFLD-related cardiovascular disease. Biochem. Pharmacol. 218, 115900. doi:10.1016/j.bcp.2023.115900
Bidault-Jourdainne, V., Merlen, G., Glenisson, M., Doignon, I., Garcin, I., Pean, N., et al. (2021). TGR5 controls bile acid composition and gallbladder function to protect the liver from bile acid overload. JHEP Rep. 3, 100214. doi:10.1016/j.jhepr.2020.100214
Chang, D. S., Soni, K. G., and Preidis, G. A. (2024). Smooth muscle contractile responses to bile acids in mouse ileum require TGR5 but not ASBT. Front. Neurol. 15, 1334319. doi:10.3389/fneur.2024.1334319
Cheng, D., Fan, Y., Fang, S., Wang, M., and Liu, H. (2022). ResNet-AE for radar signal anomaly detection. Sensors (Basel) 22, 6249. doi:10.3390/s22166249
Cheng, K. C., Chang, W. T., Kuo, F. Y., Chen, Z. C., Li, Y., and Cheng, J. T. (2019). TGR5 activation ameliorates hyperglycemia-induced cardiac hypertrophy in H9c2 cells. Sci. Rep. 9, 3633. doi:10.1038/s41598-019-40002-0
Chi, B., Zhang, M., Sun, L., Liu, H., and Tian, Z. (2024). Study on the hypotensive effect and mechanism of hawthorn (Crataegus pinnatifida) fruits and hyperoside in spontaneously hypertensive rats. Food Funct. 15, 5627–5640. doi:10.1039/d3fo02641h
Chiang, J. Y. L., and Ferrell, J. M. (2020). Bile acid receptors FXR and TGR5 signaling in fatty liver diseases and therapy. Am. J. Physiol. Gastrointest. Liver Physiol. 318, G554-G573–G573. doi:10.1152/ajpgi.00223.2019
Doroszko, A., Radziwon-Balicka, A., and Skomro, R. (2020). Novel approaches for diagnosing and management of cardiovascular disorders mediated by oxidative stress. Oxid. Med. Cell Longev. 2020, 7096727. doi:10.1155/2020/7096727
Fiorucci, S., Zampella, A., Cirino, G., Bucci, M., and Distrutti, E. (2017). Decoding the vasoregulatory activities of bile acid-activated receptors in systemic and portal circulation: role of gaseous mediators. Am. J. Physiol. Heart Circ. Physiol. 312, H21-H32–H32. doi:10.1152/ajpheart.00577.2016
Fleishman, J. S., and Kumar, S. (2024). Bile acid metabolism and signaling in health and disease: molecular mechanisms and therapeutic targets. Signal Transduct. Target Ther. 9, 97. doi:10.1038/s41392-024-01811-6
Forteza, M. J., Berg, M., Edsfeldt, A., Sun, J., Baumgartner, R., Kareinen, I., et al. (2023). Pyruvate dehydrogenase kinase regulates vascular inflammation in atherosclerosis and increases cardiovascular risk. Cardiovasc Res. 119, 1524–1536. doi:10.1093/cvr/cvad038
Ge, Y., Wang, J., Wu, L., and Wu, J. (2024). Gut microbiota: a potential new regulator of hypertension. Front. Cardiovasc Med. 11, 1333005. doi:10.3389/fcvm.2024.1333005
Gou, X., Qin, L., Wu, D., Xie, J., Lu, Y., Zhang, Q., et al. (2023). Research progress of Takeda G protein-coupled receptor 5 in metabolic syndrome. Molecules 28, 5870. doi:10.3390/molecules28155870
Grammatika Pavlidou, N., Dobrev, S., Beneke, K., Reinhardt, F., Pecha, S., Jacquet, E., et al. (2023). Phosphodiesterase 8 governs cAMP/PKA-dependent reduction of L-type calcium current in human atrial fibrillation: a novel arrhythmogenic mechanism. Eur. Heart J. 44, 2483–2494. doi:10.1093/eurheartj/ehad086
Guo, C., Chen, W. D., and Wang, Y. D. (2016). TGR5, not only a metabolic regulator. Front. Physiol. 7, 646. doi:10.3389/fphys.2016.00646
Guo, Y., Qiao, R., Xie, G., Yao, Y., Du, C., Shao, Y., et al. (2024). Activation of TGR5 increases urine concentration by inducing AQP2 and AQP3 expression in renal medullary collecting ducts. Kidney Dis. (Basel) 10, 181–192. doi:10.1159/000538107
Hodgson, R., Kennedy, B. K., Masliah, E., Scearce-Levie, K., Tate, B., Venkateswaran, A., et al. (2020). Aging: therapeutics for a healthy future. Neurosci. Biobehav Rev. 108, 453–458. doi:10.1016/j.neubiorev.2019.11.021
Holter, M. M., Chirikjian, M. K., Govani, V. N., and Cummings, B. P. (2020). TGR5 signaling in hepatic metabolic health. Nutrients 12, 2598. doi:10.3390/nu12092598
Ishimwe, J. A., Dola, T., Ertuglu, L. A., and Kirabo, A. (2022). Bile acids and salt-sensitive hypertension: a role of the gut-liver axis. Am. J. Physiol. Heart Circ. Physiol. 322, H636–H646. doi:10.1152/ajpheart.00027.2022
Izzo, C., Vitillo, P., Di Pietro, P., Visco, V., Strianese, A., Virtuoso, N., et al. (2021). The role of oxidative stress in cardiovascular aging and cardiovascular diseases. Life (Basel) 11, 60. doi:10.3390/life11010060
Jen, H. L., Yin, W. H., Chen, J. W., and Lin, S. J. (2017). Endothelin-1-Induced cell hypertrophy in cardiomyocytes is improved by fenofibrate: possible roles of adiponectin. J. Atheroscler. Thromb. 24, 508–517. doi:10.5551/jat.36368
Jiang, H., Fang, T., and Cheng, Z. (2023). Mechanism of heart failure after myocardial infarction. J. Int. Med. Res. 51, 3000605231202573. doi:10.1177/03000605231202573
Jiang, W., Xiong, Y., Li, X., and Yang, Y. (2021). Cardiac fibrosis: cellular effectors, molecular pathways, and exosomal roles. Front. Cardiovasc Med. 8, 715258. doi:10.3389/fcvm.2021.715258
Jin, W., Zheng, M., Chen, Y., and Xiong, H. (2024). Update on the development of TGR5 agonists for human diseases. Eur. J. Med. Chem. 271, 116462. doi:10.1016/j.ejmech.2024.116462
Kaya, D., Kaji, K., Tsuji, Y., Yamashita, S., Kitagawa, K., Ozutsumi, T., et al. (2019). TGR5 activation modulates an inhibitory effect on liver fibrosis development mediated by anagliptin in diabetic rats. Cells 8, 1153. doi:10.3390/cells8101153
Kim, D. H., Park, J. S., Choi, H. I., Kim, C. S., Bae, E. H., Ma, S. K., et al. (2023). The role of the farnesoid X receptor in kidney health and disease: a potential therapeutic target in kidney diseases. Exp. Mol. Med. 55, 304–312. doi:10.1038/s12276-023-00932-2
Kong, P., Cui, Z. Y., Huang, X. F., Zhang, D. D., Guo, R. J., and Han, M. (2022). Inflammation and atherosclerosis: signaling pathways and therapeutic intervention. Signal Transduct. Target Ther. 7, 131. doi:10.1038/s41392-022-00955-7
Lacanna, G., Wagenaar, C., Avermaete, T., and Swami, V. (2019). Evaluating the psychosocial impact of indoor public spaces in complex healthcare settings. HERD 12, 11–30. doi:10.1177/1937586718812439
Li, J., Cheng, R., and Wan, H. (2020). Overexpression of TGR5 alleviates myocardial ischemia/reperfusion injury via AKT/GSK-3β mediated inflammation and mitochondrial pathway. Biosci. Rep. 40. doi:10.1042/BSR20193482
Li, X., Han, K. Q., Shi, Y. N., Men, S. Z., Li, S., Sun, M. H., et al. (2017). Effects and mechanisms of ursodeoxycholic acid on isoprenaline-Induced myocardial fibrosis in mice. Zhonghua Yi Xue Za Zhi 97, 387–391. doi:10.3760/cma.j.issn.0376-2491.2017.05.013
Li, Y., Gao, Y. N., Zhu, Y. B., Lu, W. F., Yu, J. Y., Dong, Y. Y., et al. (2024). Taurocholic acid ameliorates hypertension through the activation of TGR5 in the hypothalamic paraventricular nucleus. Food Funct. 15, 5088–5102. doi:10.1039/d4fo00808a
Lin, Y. K., Chen, Y. A., Lee, T. I., Chen, Y. C., Chen, S. A., and Chen, Y. J. (2018). Aging modulates the substrate and triggers remodeling in atrial fibrillation. Circ. J. 82, 1237–1244. doi:10.1253/circj.CJ-17-0242
Lun, W., Yan, Q., Guo, X., Zhou, M., Bai, Y., He, J., et al. (2024). Mechanism of action of the bile acid receptor TGR5 in obesity. Acta Pharm. Sin. B 14, 468–491. doi:10.1016/j.apsb.2023.11.011
Ma, J., Hong, Y., Zheng, N., Xie, G., Lyu, Y., Gu, Y., et al. (2020). Gut microbiota remodeling reverses aging-associated inflammation and dysregulation of systemic bile acid homeostasis in mice sex-specifically. Gut Microbes 11, 1450–1474. doi:10.1080/19490976.2020.1763770
Ma, K., Tang, D., Yu, C., and Zhao, L. (2021). Progress in research on the roles of TGR5 receptor in liver diseases. Scand. J. Gastroenterol. 56, 717–726. doi:10.1080/00365521.2021.1903547
Mills, K. T., Stefanescu, A., and He, J. (2020). The global epidemiology of hypertension. Nat. Rev. Nephrol. 16, 223–237. doi:10.1038/s41581-019-0244-2
Miyazaki-Anzai, S., Masuda, M., Kohno, S., Levi, M., Shiozaki, Y., Keenan, A. L., et al. (2018). Simultaneous inhibition of FXR and TGR5 exacerbates atherosclerotic formation. J. Lipid Res. 59, 1709–1713. doi:10.1194/jlr.M087239
Moretti, A., Li, Q., Chmielowski, R., Joseph, L. B., Moghe, P. V., and Uhrich, K. E. (2018). Nanotherapeutics Containing lithocholic acid-based Amphiphilic Scorpion-Like Macromolecules reduce in vitro inflammation in macrophages: implications for atherosclerosis. Nanomaterials (Basel) 8, 84. doi:10.3390/nano8020084
Pavlovic, N., Stanimirov, B., and Mikov, M. (2017). Bile acids as novel Pharmacological agents: the interplay between gene polymorphisms, epigenetic factors and drug response. Curr. Pharm. Des. 23, 187–215. doi:10.2174/1381612822666161006161409
Perino, A., Demagny, H., Velazquez-Villegas, L., and Schoonjans, K. (2021). Molecular Physiology of bile acid signaling in health, disease, and aging. Physiol. Rev. 101, 683–731. doi:10.1152/physrev.00049.2019
Queen, N. J., Hassan, Q. N., and Cao, L. (2020). Improvements to Healthspan through environmental enrichment and Lifestyle interventions: where are We Now? Front. Neurosci. 14, 605. doi:10.3389/fnins.2020.00605
Reilly-O'Donnell, B., Ferraro, E., Tikhomirov, R., Nunez-Toldra, R., Shchendrygina, A., Patel, L., et al. (2024). Protective effect of UDCA against IL-11- induced cardiac fibrosis is mediated by TGR5 signalling. Front. Cardiovasc Med. 11, 1430772. doi:10.3389/fcvm.2024.1430772
Sauerbruch, T., Hennenberg, M., Trebicka, J., and Beuers, U. (2021). Bile acids, liver Cirrhosis, and Extrahepatic vascular dysfunction. Front. Physiol. 12, 718783. doi:10.3389/fphys.2021.718783
Sheikh, M. S. A., and Salma, U. (2024). Impact of microRNAs on cardiovascular diseases and aging. J. Int. Med. Res. 52, 3000605241279190. doi:10.1177/03000605241279190
Shu, H., Peng, Y., Hang, W., Nie, J., Zhou, N., and Wang, D. W. (2022). The role of CD36 in cardiovascular disease. Cardiovasc Res. 118, 115–129. doi:10.1093/cvr/cvaa319
Su, J., Zhao, Y., Chen, W. D., and Wang, Y. D. (2024). Activation of the G protein-coupled bile acid receptor TGR5 modulates the HCP5/miR-139-5p/DDIT4 Axis to antagonize Cervical cancer progression. Int. J. Mol. Sci. 25, 8932. doi:10.3390/ijms25168932
Sweeney, M., Corden, B., and Cook, S. A. (2020). Targeting cardiac fibrosis in heart failure with preserved ejection fraction: mirage or miracle? EMBO Mol. Med. 12, e10865. doi:10.15252/emmm.201910865
Tamma, G., Goswami, N., Reichmuth, J., De Santo, N. G., and Valenti, G. (2015). Aquaporins, vasopressin, and aging: current perspectives. Endocrinology 156, 777–788. doi:10.1210/en.2014-1812
Tanaka, C., Harada, N., Teraoka, Y., Urushizaki, H., Shinmori, Y., Onishi, T., et al. (2024). Mogrol stimulates G-protein-coupled bile acid receptor 1 (GPBAR1/TGR5) and insulin secretion from pancreatic beta-cells and alleviates hyperglycemia in mice. Sci. Rep. 14, 3244. doi:10.1038/s41598-024-53380-x
Tiwari, S., Li, L., Riazi, S., Halagappa, V. K., and Ecelbarger, C. M. (2009). Sex and age result in differential regulation of the renal thiazide-sensitive NaCl cotransporter and the epithelial sodium channel in angiotensin II-infused mice. Am. J. Nephrol. 30, 554–562. doi:10.1159/000252776
Tracy, E., Rowe, G., and LeBlanc, A. J. (2020). Cardiac tissue remodeling in healthy aging: the road to pathology. Am. J. Physiol. Cell Physiol. 319, C166-C182–C182. doi:10.1152/ajpcell.00021.2020
van Nierop, F. S., Scheltema, M. J., Eggink, H. M., Pols, T. W., Sonne, D. P., Knop, F. K., et al. (2017). Clinical relevance of the bile acid receptor TGR5 in metabolism. Lancet Diabetes Endocrinol. 5, 224–233. doi:10.1016/S2213-8587(16)30155-3
Wang, C., Ma, Q., and Yu, X. (2023). Bile acid network and vascular Calcification-associated diseases: Unraveling the intricate Connections and therapeutic potential. Clin. Interv. Aging 18, 1749–1767. doi:10.2147/CIA.S431220
Wang, H., Wang, J., Cui, H., Fan, C., Xue, Y., Liu, H., et al. (2024). Inhibition of fatty acid uptake by TGR5 prevents diabetic cardiomyopathy. Nat. Metab. 6, 1161–1177. doi:10.1038/s42255-024-01036-5
Wang, J., Zhang, J., Lin, X., Wang, Y., Wu, X., Yang, F., et al. (2021). DCA-TGR5 signaling activation alleviates inflammatory response and improves cardiac function in myocardial infarction. J. Mol. Cell Cardiol. 151, 3–14. doi:10.1016/j.yjmcc.2020.10.014
Wang, X. X., Luo, Y., Wang, D., Adorini, L., Pruzanski, M., Dobrinskikh, E., et al. (2017). A dual agonist of farnesoid X receptor (FXR) and the G protein-coupled receptor TGR5, INT-767, reverses age-related kidney disease in mice. J. Biol. Chem. 292, 12018–12024. doi:10.1074/jbc.C117.794982
Wang, X. X., Xie, C., Libby, A. E., Ranjit, S., Levi, J., Myakala, K., et al. (2022). The role of FXR and TGR5 in reversing and preventing progression of Western diet-induced hepatic steatosis, inflammation, and fibrosis in mice. J. Biol. Chem. 298, 102530. doi:10.1016/j.jbc.2022.102530
Xia, X., Xu, Q., Liu, M., Chen, X., Liu, X., He, J., et al. (2020). Deubiquitination of CD36 by UCHL1 promotes foam cell formation. Cell Death Dis. 11, 636. doi:10.1038/s41419-020-02888-x
Xie, S., Xu, S. C., Deng, W., and Tang, Q. (2023). Metabolic landscape in cardiac aging: insights into molecular biology and therapeutic implications. Signal Transduct. Target Ther. 8, 114. doi:10.1038/s41392-023-01378-8
Xu, L., Wu, X., Long, L., Li, S., Huang, M., Li, M., et al. (2025). TGR5 attenuates DOCA-salt hypertension through regulating histone H3K4 methylation of ENaC in the kidney. Metabolism 165, 156133. doi:10.1016/j.metabol.2025.156133
Xu, N., He, Y., Zhang, C., Zhang, Y., Cheng, S., Deng, L., et al. (2024). TGR5 signalling in heart and brain injuries: focus on metabolic and ischaemic mechanisms. Neurobiol. Dis. 192, 106428. doi:10.1016/j.nbd.2024.106428
Yan, W., Xia, Y., Zhao, H., Xu, X., Ma, X., and Tao, L. (2024). Stem cell-based therapy in cardiac repair after myocardial infarction: Promise, challenges, and future directions. J. Mol. Cell Cardiol. 188, 1–14. doi:10.1016/j.yjmcc.2023.12.009
Ye, D., He, J., and He, X. (2024). The role of bile acid receptor TGR5 in regulating inflammatory signalling. Scand. J. Immunol. 99, e13361. doi:10.1111/sji.13361
Yin, X., Yin, X., Pan, X., Zhang, J., Fan, X., Li, J., et al. (2023). Post-myocardial infarction fibrosis: Pathophysiology, examination, and intervention. Front. Pharmacol. 14, 1070973. doi:10.3389/fphar.2023.1070973
Zhan, K., Wu, H., Xu, Y., Rao, K., Zheng, H., Qin, S., et al. (2024). The function of the gut microbiota-bile acid-TGR5 axis in diarrhea-predominant irritable bowel syndrome. mSystems 9, e0129923. doi:10.1128/msystems.01299-23
Zhang, L., Pitcher, L. E., Yousefzadeh, M. J., Niedernhofer, L. J., Robbins, P. D., and Zhu, Y. (2022). Cellular senescence: a key therapeutic target in aging and diseases. J. Clin. Invest. 132, e158450. doi:10.1172/JCI158450
Zhao, C., Wu, K., Hao, H., Zhao, Y., Bao, L., Qiu, M., et al. (2023). Gut microbiota-mediated secondary bile acid alleviates Staphylococcus aureus-induced mastitis through the TGR5-cAMP-PKA-NF-κB/NLRP3 pathways in mice. NPJ Biofilms Microbiomes 9, 8. doi:10.1038/s41522-023-00374-8
Keywords: aging, cardiovascular disease, TGR5, heart failure, myocardial infarction
Citation: He Y, Liu S, Zhang Y, Zuo Y, Huang K, Deng L, Liao B, Zhong Y and Feng J (2025) Takeda G protein–coupled receptor 5 (TGR5): an attractive therapeutic target for aging-related cardiovascular diseases. Front. Pharmacol. 16:1493662. doi: 10.3389/fphar.2025.1493662
Received: 09 September 2024; Accepted: 07 March 2025;
Published: 20 March 2025.
Edited by:
Isac Almeida de Medeiros, Universidade Federal da Paraíba, BrazilReviewed by:
Dwijendra K. Gupta, Allahabad University, IndiaCopyright © 2025 He, Liu, Zhang, Zuo, Huang, Deng, Liao, Zhong and Feng. This is an open-access article distributed under the terms of the Creative Commons Attribution License (CC BY). The use, distribution or reproduction in other forums is permitted, provided the original author(s) and the copyright owner(s) are credited and that the original publication in this journal is cited, in accordance with accepted academic practice. No use, distribution or reproduction is permitted which does not comply with these terms.
*Correspondence: Jian Feng, amVycnlmZW5nQHN3bXUuZWR1LmNu
†These authors have contributed equally to this work
Disclaimer: All claims expressed in this article are solely those of the authors and do not necessarily represent those of their affiliated organizations, or those of the publisher, the editors and the reviewers. Any product that may be evaluated in this article or claim that may be made by its manufacturer is not guaranteed or endorsed by the publisher.
Research integrity at Frontiers
Learn more about the work of our research integrity team to safeguard the quality of each article we publish.