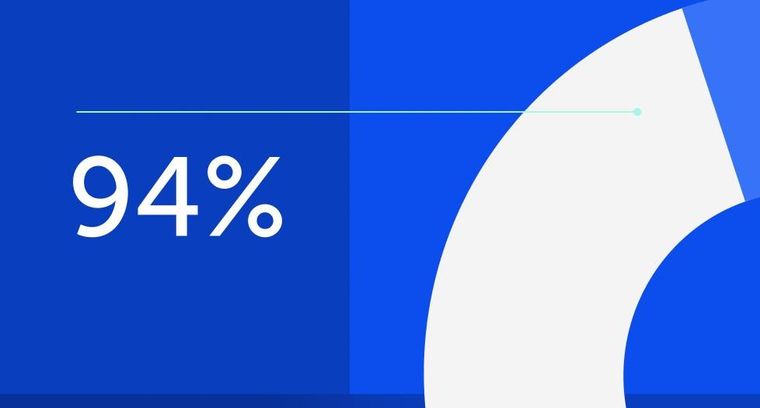
94% of researchers rate our articles as excellent or good
Learn more about the work of our research integrity team to safeguard the quality of each article we publish.
Find out more
ORIGINAL RESEARCH article
Front. Pharmacol., 16 April 2025
Sec. Ethnopharmacology
Volume 16 - 2025 | https://doi.org/10.3389/fphar.2025.1485952
This article is part of the Research TopicHerbal Medicine for the Treatment of Chronic Metabolic Diseases, Volume IIView all 18 articles
Background: Dracaena trifasciata (Prain) Mabb. [Asparagaceae; also known as Sansevieria trifasciata Prain (ST)] may have health-promoting activities, including resolution of diabetes mellitus (DM). This in vitro study evaluated whether and how a leaf extract of ST could directly protect pancreas-derived MIN6 cells against the diabetogenic toxin streptozotocin (STZ).
Methods: Composition of the ST extract (by 100% methanol) was investigated using high resolution mass spectrometry, which revealed several compounds with beneficial bioactive efficacy. MIN6 cells were exposed to 50% lethal dose of STZ, with or without ST extract. Cell viability was assessed using the MTT method. Inflammatory activity of ST extract was assessed in MIN6 cells and macrophage-like RAW cells, and addition of TNF-α to combinations of ST and STZ were tested on MIN6 cell viability. The role of the NF-κB pathway in effects of STZ and ST were investigated using the proteosome inhibitor MG132.
Results: Exposure of MIN6 cells to the ST extract (in concentrations that did not notably affect MIN6 cells: 5-15 mg/mL) was indeed able to minimize STZ-induced toxicity in MIN6 cells. Exposing macrophage-like RAW cells to ST extract (at 10-15 mg/mL) increased TNF-α gene expression, and this response was highly augmented by co-exposure to lipopolysaccharide (LPS, 1.0 mg/mL), indicating that ST extract contained inflammatory compounds too. The implication of this finding was investigated by exposing MIN6 cells to a subthreshold dose (100 ng/mL) of TNF-α, which 1) prevented the protective effect of the ST extract (10-15 mg/mL) against STZ toxicity, and 2) caused ST to become toxic to MIN6 cells even without the presence of STZ. TNF-α is known to activate the NF-κB pathway leading to cell death, however, NF-κB is also known to stimulate cell proliferation and survival. To investigate the relevance of the NF-κB pathway in our findings, we treated MIN6 cells with the proteasome inhibitor MG132 (at doses ≥0.2 μM), and observed that ST extract was no longer able to block STZ toxicity in MIN6 cells (p < 0.05), and to block CIAP2 expression, an anti-apoptotic target downstream from NF-κB.
Conclusion: These data suggest that a leaf extract of ST has anti-diabetogenic efficacy, which may depend on the integrity of the NF-κB pathway. This protective effect appears to be impeded in a pro-inflammatory environment.
According to the IDF 537 million adults are living with diabetes. This number is predicted to rise to 634 million by 2030 and 783 million by 2045 (Cho et al., 2018). Loss of beta cell mass is a critical process in the etiology of type 1 (T1DM) and late-stage type 2 (T2DM) Diabetes Mellitus. While loss of beta cell mass in T1DM represents the key culprit of the disease (by autoimmune or inflammatory processes), progression of insulin resistance and hyperlipidemia leads to metabolic and inflammatory stress in pancreatic beta cells, ultimately leading to their death (by apoptosis) in T2DM (Rachdaoui, 2020; Xu et al., 2015). Diabetes can be experimentally induced with a beta cell toxin like alloxan or streptozotocin (Lenzen, 2008; Furman, 2015), and is often studied in rats or mice with representation of many characteristics also seen in humans with diabetes. Humans exposed to persistent metabolic and inflammatory stressors can experience a clinically relevant loss of beta cell mass, but some individuals appear to be less sensitive than others (Cerf, 2013; Chacón et al., 2007). It is possible that these individuals have a defense repertoire within their pancreatic beta cells capable of counteracting the damaging effects of metabolic and inflammatory stress (Bonora, 2008).
Diabetes is often accompanied by tissue infiltration of monocytes and macro-phages (Goldfine et al., 2011; Eguchi and Nagai, 2017) and recent advances have stimulated research targeting inflammatory pathways as part of the strategy to prevent or control the disease (Goldfine et al., 2011), but mixed results are found (Kolb and Eizirik, 2012; Sheehy et al., 2019). During metabolic and/or inflammatory stress, cells that express anti-apoptotic genes are able to counteract the pathways leading to cell death (Cerf, 2013; Kolb and Eizirik, 2012; Elmore, 2007). It is possible that failure of the defense response of pancreatic beta cells is due to the fact that the inflammatory pathways/cytokines “switch” from an anti-apoptotic phenotype towards a more pro-apoptotic one. TNF-α and the NF-κB pathway have been stated to regulate this “switch” between anti-apoptotic and pro-apoptotic pathways (Karin and Lin, 2002). Indeed, changes within the NF-κB pathway resulting in a shift towards apoptosis have been observed in rat hepatocytes and pancreatic beta cells during inflammatory stress (Hun et al., 2005; Schoemaker et al., 2002). Targeting this pathway may protect beta cells against metabolic and inflammatory stress and may curb the pathophysiology of diabetes.
Research on traditional medicine increased in recent years (Erejuwa et al., 2012; Shakib et al., 2019; Patel et al., 2012; Qomariyah et al., 2012; González-Ponce et al., 2018). Many bioactive ingredients in plants have diverse therapeutic niches, either as FDA-approved drug or as a supplement, and some have entered the market successfully (Li and Weng, 2017), with the prototypical example being metformin. Because of the great potential of plant compounds for clinical purposes (Erejuwa et al., 2012; Shakib et al., 2019; Patel et al., 2012; Qomariyah et al., 2012; González-Ponce et al., 2018; Li and Weng, 2017; World Health Organization WHO, 2013), the World Health Organization (WHO) has developed a strategy to implement traditional medicine as a way of coping with the relentless rise of non-communicable diseases (World Health Organization WHO, 2013).
The Dracaena trifasciata (Prain) Mabb. [Asparagaceae; better known under its synonym Sansevieria trifasciata Prain (ST), the name used in this paper], is used as a “traditional medicine” and has shown potential benefits as treatment against T2DM (Qomariyah et al., 2012; El-Din El-Hawary et al., 2021; Raslan et al., 2021). Oral administration of extracts of ST leaves to decrease circulating blood glucose levels and increase insulin-containing granule density in pancreatic beta cells in a diabetic rat model (Qomariyah et al., 2012). Under the assumption that some of the bioactive molecules within ST may actually be absorbed and affect key organs (such as the pancreas) involved in cardiometabolic health, the aim of the current in vitro study was to identify the phytochemical profile of ST leaf extract and evaluate whether ST leaf extract could directly protect cells from a pancreatic beta cell line (MIN6) against the pancreatic beta cell toxin streptozotocin (STZ), and – if so – to identify potential mechanisms underlying such a protective effect.
Freshly cultivated leaves of Sansevieria trifasciata Prain (ST) were collected from the Tropical Biopharmaca Research Center (IPB), Bogor Agricultural Institute, Bogor, Indonesia (March 2014; see Supplementary Material S1 for the letter of the head of the Tropical Biopharmaca Research Center). A botanist (Dr. J.S. Rahajoe) identified ST with the specimen code BMK0130092016 (Biopharmaka taxonomy database; Tropical Biopharmaca Research Center (IPB), Bogor Agricultural Institute, Bogor, Indonesia; see Supplementary Material S2). The leaves were dried at 38°C ± 2°C for 7 days, after which the leaves were powdered and kept at room temperature in dry and dark conditions followed by cold maceration extraction. In brief, the pulverized leaf material was dissolved in 100% methanol (5 mL/g material) and the leaf extract was shaken at room temperature for 5 days (Mimaki et al., 1996). The leaf extract was filtered and evaporated using the rotary evaporator system at 60°C under vacuum for 3 h (Rotavapor, RTV-01, Buchi, Switzerland) to obtain a semi-solid extract (Qomariyah et al., 2012; Lontoc et al.). The product was kept in a closed flask and stored at −20°C until the start of the experiment. Before experiments, the semi-solid ST extract was dissolved in PBS, filtered (4.5 μm pore size), and shielded from light.
The full fingerprint following the standards established in the ConPhyMP statement (see Supplementary Materials S3, S4) of the ST extract was determined with liquid chromatography – high resolution mass spectrometry (LC-HRMS; Laboratorium Sentral Ilmu Hayati Universitas Brawijaya, Malang, Indonesia and Interfaculty Mass Spectrometry Center Department of Analytical Biochemistry University of Groningen, Groningen, the Netherlands). The LC-HRMS column (H5PMS, J&W Scientific) was a hypersil GOLD AQ Colum 50 × 1 mm and 1.9 μm particle size. The column and autosampler temperature were set at 50°C, respectively. The flow was set at 40 μL/min. Mobile phase A was water with 0.1% formic acid, and mobile phase B acetonitrile with 0.1% formic acid. A linear gradient was used: 0–2 min 5% B, 2–12 min linear increase to 40% B, 12–14 min linear increase to 60% B, 14–15.5 min linear increase to 100% B, 15.5–17 min held at 100% B, 17–17.1 min decrease to 5% B, and 17.1–20 min held at 5% B.
MS and MS/MS analyses were performed with electrospray ionization in positive mode at a spray voltage of 3.5 kV, and sheath and auxiliary gas flow set at 47.5 and 11.25, respectively. The ion transfer tube temperature was 256°C. Spectra were acquired in data-dependent mode with a survey scan at m/z 100–1,500 at a resolution of 70,000 followed by MS/MS fragmentation of the top 5 precursor ions at a resolution of 17,500. A normalized collision energy (NCE) of 30 was used for fragmentation and fragmented precursor ions were dynamically excluded for 10s. The data was analyzed by Compound Discoverer with MzCloud MS/MS Library (Adriani et al., 2022).
MIN6 cells (a mouse beta cell line originally established from an insulinoma developed in an IT6 transgenic C57BL/6 mouse line, which expresses the SV40 T antigen under control of the human insulin promoter (Miyazaki et al., 1990); passage 35–44) were cultured in Dulbecco’s modified Eagle’s (DMEM) high glucose medium, supplemented with 2 mM L-glutamine, 10% FCS, 0.2 µM β-mercapto-ethanol, 1% P/S, and humidified 5% CO2 at 37°C. RAW cells (ATCC® TIB-71, a mouse macrophage cell line originally established from a tumor in male mice induced with the Abselon murine leukemia virus (Raschke et al., 1978); passage 28–32) were cultured in DMEM medium, supplemented with 2 mM L-glutamine, 10% FCS and 0.1% Gentamicin, and humidified 5% CO2 at 37°C. The media of both cell types were changed 3 times a week (Ahn et al., 2020; Zhou et al., 2014).
Streptozotocin (STZ; Sigma-Aldrich, S0130-5G) was used due to its specific toxicity towards insulin-producing pancreatic beta cells (Eleazu et al., 2013). It has similarities with glucose and is transported into the cell by the glucose transport two protein (GLUT 2) (Eleazu et al., 2013; Saini et al., 1996). Before individual experiments, STZ was dissolved in 0.1 M citrate buffer (pH = 4.5), filtered (4.5 µm pore size), kept on ice, and shielded from light. The protease inhibitor MG132 (Sigma-Aldrich, CAS Number 133407-82-6) was used as blocker of the NF-κB pathway (Zanotto-Filho et al., 2012; Zanotto-Filho et al., 2010). TNF-α (Peprotech; catalog 315-01A) and lipopolysaccharide (LPS; Sigma-Aldrich CAS Number L2880) were used to induce an inflammatory response (Chen et al., 2018; Chang et al., 2003).
Cell viability of MIN6 cells was assessed using the MTT (3-(4, 5-dimethylthiazol-2-yl)-2, 5-diphenyltetrazolium bromide) assay (Kumar et al., 2018). Before the experiments, cells were washed twice with phosphate buffered saline (PBS), and detached from the flasks with 0.5 mL Trypsin (incubation time: 5 min at 37°C and 5% CO2). The cells were collected in medium and centrifuged for 5 min at 14,000 RPM. Cell count was performed with 50 µL of 0.4% Trypan Blue, 3.0 × 105 cells/mL (60.000 cells/well) were plated in a 96-wells plate and incubated overnight (37°C and 5% CO2).
To validate if the methanol extract of ST could be safely used, different doses (2.5–20 mg/mL; 1.5%–10% v/v) of the ST extract were dissolved in different standard cell culture solvents (PBS, EtOH 0.5% and Saline) and applied to MIN6 cells. Viability of MIN6 cells was studied with several concentrations of streptozotocin (STZ: 0–20 mM) creating a dose-response curve (Eleazu et al., 2013; Gao et al., 2000), allowing the determination of the lethal dose of 50% (LD50%).
To investigate the protective effect of ST extract, cells were exposed to a LD 50% dose of STZ (10–15 mM) for 1 hour. Based on pilot experiments, doses of ST extract (2.5–20 mg/mL in PBS, 1.5%–10% v/v) were added 30 min before (prophylactic treatment) or directly after the addition of STZ (therapeutic treatment). To investigate whether the activity of ST extract was influenced by a pro-inflammatory environment, MIN6 cells were incubated with TNF-α (100 ng/mL (Chang et al., 2003)) for 6 hours (Start: 5 hours before the exposure to STZ). To investigate if the activity of ST extract was dependable on the NF-kB pathway, the proteasome inhibitor MG132 was administered for 3 hours (Start: 2 hours before the exposure to STZ) (Zanotto-Filho et al., 2010).
After the experiments, medium was refreshed and cells were incubated for 24 hours (37°C and 5% CO2). A volume of 22 µL MTT (0.5 mg/mL) was added to each well and shielded from light and the MIN6 cells were incubated for a final 2.5 h. The formazan crystals formed by the reduction of MTT under the action of mitochondrial dehydrogenase were dissolved with dimethyl sulfoxide (DMSO; 150 µL) and measured by a Micro-plate Reader (Bio-Tec Instruments Inc.) between wavelengths 570–630 nm. The value was considered to reflect the activity of cell metabolism and is expressed as cell viability (% compared to the untreated cells: indicated as negative control).
To investigate whether ST extract had inflammatory properties, gene expression was determined in MIN6 and RAW cells. 3.0 × 105 cells/mL (6.0 × 105 cells/well) were plated in a 6-wells plate and incubated overnight. The MIN6 cells were exposed to MG132, STZ and ST extract as described before, and the RAW cells were exposed to different doses of ST extract (2.5–20 mg/mL in PBS, 1.5%–10% v/v) 30 min before (prophylactic treatment) or directly after (therapeutic treatment) the addition of a toxic dose of LPS (1.0 mg/mL).
RNA was isolated using Trizol Reagent (Sigma-Aldrich) and the RNA quality and quantity were determined using Nanodrop spectrophotometer (Thermo Scientific, Wilmington, DE, United States). Reverse transcription PCR (RT-PCR) was performed using the Moloney murine leukemia virus (M–MLV) reverse transcriptase system and random nanomers from Life Technologies (Breda, Netherlands). RT-PCR was performed in three steps: 10 min at 25°C, 1 h at 37°C and 5 min at 95°C with the GenAmp PCR system (Applied Biosystems, Niewekerk a/d IJssel, the Netherlands). Quantitative real-time PCR (qPCR) was performed using 4 µL 20-fold diluted cDNA in combination with 2x master mix (Eurogentec, Maastricht, Netherlands) in a total volume of 20 µL. Gene expression was expressed as fold induction and normalized for 18S as house-keeping gene (see Supplementary Material S7) (Wang et al., 2024).
All assays were repeated three times in duplicate or triplicate. Data is expressed as mean ± standard error of the mean (SEM). Differences between groups were tested for significance using one-way ANOVA with post hoc Tukey analysis. P-values <0.05 were considered statistically significant and were performed with IBM SPSS software 23.
LC-HRMS analysis showed the presence of 142 compounds in the ST extract. The full fingerprint of compound identification based on mass spectrometry is shown in Supplementary Material S5 (Heinrich et al., 2022). Only compounds with a quality ≥90% are stated in Table 1, the specific spectra and compound identification of Table 1 is shown in Supplementary Material S6.
Table 1. Retention time (RT), identification and analysis of the compounds of Sansevieria trifasciata (ST). Only compounds with quality ≥90% are taken into account (full list available in the Supplementary Material S2).
MIN6 cells were incubated with methanol leaf extract of ST that was redissolved in different vehicles commonly used in cell culture research (PBS, Saline, EtOH 0.5%). The MTT-assay revealed no effect of vehicle (PBS, Saline or EtOH 0.5%) on cell viability compared to the negative control (NC: no ST was added to this group; Figure 1A). For this reason, PBS was used as vehicle for the ST extract for the remainder of the experiments. MIN6 cells incubated with streptozotocin (STZ) resulted in a dose-dependent decrease of cell viability compared to the untreated cells (Figure 1B; R2 = 0.8315; p < 0.001). In order to determine if the ST extract had protective effects against STZ-induced reduction in cell viability (LD50%), the ST extract was used in a prophylactic and therapeutic regimen. Both the prophylactic (Figure 1C; p < 0.05) and therapeutic (Figure 1D; p < 0.05) treatment with ST extract (in the doses of 5–15 mg/mL) significantly increased viability of MIN6 cells (from 50% to ± 75% cell viability; p < 0.05) following exposure to STZ at a dose causing 50% reduction of cell viability.
Figure 1. Cell viability (%) of MIN6 cells after administration of Sansevieria trifasciata leaf extract (ST) in different vehicles [(A); NC = negative control (no ST was added to this group), PBS = phosphate buffered saline, EtOH 0.5% = ethanol 0.5%], (B) after exposure to increasing concentrations of streptozotocin (STZ). Cell viability (%) after prophylactic (C) and therapeutic (D) treatment of ST against STZ-induced reduction in cell viability (LD50%; 10 mM). Data is shown as mean ± SEM and compared to the STZ control group (indicated as; −/+), * p < 0.05 (C, D).
Infiltration of reactive inflammatory macrophages into the pancreatic islets plays an important role in beta cell loss and the pathogenesis of diabetes (Goldfine et al., 2011; Eguchi and Nagai, 2017). To this end, we studied the effect of ST extract on the inflammatory properties of macrophage-like cells (RAW cells). ST extract caused an increase in gene expression of the inflammatory genes COX2 (p < 0.001; 10–15 mg/mL; Figure 2A), iNOS (p < 0.05, 15 mg/mL; Figure 2B) and TNF-α (10–5 mg/mL; Figure 2C) in inactive RAW cells. However, ST extract showed no effect on gene expression of IL-10 (Figure 2D).
Figure 2. Relative gene expression of (A) COX2, (B) iNOS, (C) TNF-α and (D) IL10 in RAW-cells after administration of Sansevieria trifasciata leaf extract (ST). The experiment is repeated three times in du-plicate and data is shown as mean ± SEM and compared to the negative control group (indicated as; −/−), * p < 0.05, *** p < 0.001.
Next, we evaluated the effect of ST extract on LPS-activated RAW cells. LPS significantly increased the expression of COX2, iNOS and TNF-α compared to the negative control (p < 0.01-p<0.001; Figure 3). Treatment with ST extract decreased gene expression of COX2 only when applied as therapeutic regimen in the dose of 10 mg/mL (p < 0.05; Figure 3B). In contrast, gene expression of TNF-α was increased, after prophylactic (p < 0.05–0.01; 5–10 mg/mL; Figure 3E) and therapeutic (p < 0.001; 10 mg/mL; Figure 3F) treatment with ST extract. Gene expression of iNOS (Figures 3A, B) and IL-10 (Figures 3G, H) were not influenced by the administration of ST extract after LPS challenge.
Figure 3. Relative gene expression of (A, B) COX2, (C, D) iNOS, (E, F) TNF-α and (G, H) IL-10 in RAW-cells after prophylactic (A, C, E, G) or therapeutic (B, D, F, H) treatment with Sansevieria trifasciata leaf extract (ST) either or not combined with lipopolysaccharide (LPS). The experiment was repeated three times in duplicate and data is shown as mean ± SEM and compared to the LPS control group (indicate as; −/+), * p < 0.05, ** p < 0.01, *** p < 0.001.
In this study, treatment of MIN6 cells with ST extract did not result in increased gene expression of TNF-α (Supplementary Material S7). Because ST extract did cause an increased expression of TNF-α in activated and non-activated RAW cells, and assuming that this would lead to increased production and release of TNF-α, we investigated whether addition of TNF-α alone or in combination with ST extract to the medium with MIN6 cells would influence STZ-induced reduction in cell viability of MIN6 cells.
TNF-α administration alone did not influence cell viability (Figure 4), however when combined with the highest dose of ST (15 mg/mL) it did attenuate cell viability. As shown before, ST extract alone could protect MIN6 cells against STZ-induced reduction in cell viability in the doses 5–15 mg/mL (p < 0.05–0.01), however addition of TNF-α rendered ST no longer protective against STZ-induced reduction in cell viability in MIN6 cells. Specifically, while a combined administration of TNF-α and 5 mg/mL ST extract was still beneficial against STZ-induced cell death (p < 0.05; Figure 4B), the higher doses of ST extract (10–15 mg/mL) that were found to be protective without TNF-α, lost their protective effects when TNF-α was added (Figures 4C, D). Furthermore, cell viability of MIN6 cells was already decreased when ST extract (15 mg/mL) was combined with TNF-α.
Figure 4. The effect of Sansevieria trifasciata leaf extract (ST), TNF-α (100 ng/mL) or a combination of both on streptozotocin (STZ)-reduced MIN6 viability. (A) 2 mg/mL, (B) 5 mg/mL, (C) 10 mg/mL and (D) 15 mg/mL of ST leaf extract. All groups are compared with the STZ control (indicated as; −/−/+) and differences are indicated with * p < 0.05, ** p < 0.01.
To investigate whether the protective effect of ST extract depends on the NF-κB pathway, we used the proteasome inhibitor MG132 (in concentrations from 0.04 to 1.0 µM) as blocker of this pathway (Figure 5). As shown before, the ST extract did not result in reduced MIN6 cell viability per sé, and could protect substantially against STZ-induced reduction in cell viability (p < 0.05–0.01). Administration of MG132 alone (0.04 µM–1.0 µM), or in combination with ST (5 mg/mL) did not influence MIN6 cell viability. However, when ST extract was combined with MG132 (≥0.2 µM) as treatment against STZ-induced reduction in cell viability, the protective effect of the ST extract was lost. Furthermore, administration of MG132 (≥0.2 µM) in combination with STZ (15 mM) showed a slightly lower cell viability compared to that following STZ alone.
Figure 5. The effect of Sansevieria trifasciata leaf extract (ST), the proteasome inhibitor MG132 or a combination of both on streptozotocin (STZ)-reduced MIN6 viability. (A) 0.04 µM, (B) 0.2 µM, (C) 0.5 µM and (D) 1.0 µM of MG132. All groups are compared with the STZ control (indicated as; −/−/+) and differences are indicated with * p < 0.05, ** p < 0.01 (or $ p < 0.05 when the addition of MG132 had an effect).
Protease inhibitors affect many pathways (amongst others NF-κB activity), therefore, to validate if the NF-κB pathway was blocked, we assessed expression of a NF-κB pathway dependent anti-apoptotic gene, the cellular inhibitor of apoptosis 2 (cIAP2). Administration of 0.04 µM MG132, which was not effective to decrease the protective effect of ST extract, also did not affect expression level of cIAP2. However, the dose of 0.5 µM MG132, which was able to decrease the protective effect of ST extract, also caused significant reductions in cIAP2 expression in MIN6 cells across all valid comparisons (p < 0.05–0.01; Figure 6). These data show the importance of the NF-κB pathway on cell survival and that the effect of ST extract may dependent on the NF-κB pathway.
Figure 6. The effect of Sansevieria trifasciata leaf extract (ST), the proteasome inhibitor MG132 or a combination of the two on cIAP2 expression in MIN6 cells. (A) 0.04 µM, (B) 0.5 µM MG132. All groups are compared with the STZ control (indicated as; −/−/+) and differences are indicated with * p < 0.05, ** p < 0.01.
Plants are known to contain bioactive compounds with a potential for the treatment of diseases. Among these is Sansevieria trifasciata (ST), native to eastern Africa and southern Asia, with presumed anti-diabetic activity (Qomariyah et al., 2012). Mass spectrometric characterization of the ST extract in this study revealed the presence of a variety of bioactive compounds, including a relative high abundance of choline, isoleucine, α-linolenic acid, which are known to lower risk of T2DM and/or reduced insulin resistance (Alencar et al., 2018; Ward et al., 2017; Nemecz et al., 2019; Virtanen et al., 2020). The lower abundance compounds – like pyroglutamic acids (Yoshinari and Igarashi, 2011) and oxophytodienoic acid (Zhang et al., 2022) – have several health improving effects in diabetic/inflammatory conditions too, although some others are also less helpful or even toxic (Al Za’Abi et al., 2018). In our experiments, however, the ST leaf extract (by methanol and redissolved in PBS) applied to a medium containing MIN6 cells (1.5%–10% v/v) was capable of protecting against streptozotocin (STZ)-induced toxicity. Important for consideration of these findings was that addition of the ST extract alone did not affect viability/toxicity of MIN6 cells in any way. Although much work needs to be done to pinpoint these effects to the specific bioactive compounds within ST that can actually be absorbed and circulate in the blood stream, our results are in line with an in vivo study showing that ST extract has anti-diabetic effects in a rodent model for T2DM induced by alloxan (Qomariyah et al., 2012).
Diabetes is often accompanied by activation of the inflammatory system, which a.o. includes pancreatic tissue infiltration of monocytes and macrophages (Goldfine et al., 2011; Eguchi and Nagai, 2017) that locally secrete inflammatory cytokines like TNF-α. For this reason, we also investigated the effect of the ST extract on RAW cells, a macrophage-like cell line. To our surprise, we observed that ST extract itself induced a low-grade inflammatory response in inactive RAW cells as indicated by increasing gene expression of COX2, iNOS and TNF-α, especially with the higher doses of ST extract (10–15 mg/mL). Gene expression of the anti-apoptotic cytokine IL-10 was not affected by addition of ST extract, although it needs to be noted that anti-apoptotic cytokines have slower kinetics, and we simply could have missed a response of IL-10 within the time frame of this experiment (Kolb and Eizirik, 2012). Activation of RAW cells by LPS profoundly increased the expression of TNF-α, which appeared to be further enhanced by prophylactic as well as therapeutic addition of ST leaf extract. Expression of other pro-inflammatory markers, like iNOS and COX2, were not enhanced by ST extract. In fact, ST leaf extract reduced LPS-enhanced COX2 gene in the range of 10 mg/mL, which could be interesting in light of relief of insulin resistance (Hsieh et al., 2009). Although the ST extract did not induce gene expression of TNF-α in MIN6 cells, these data suggest that the ST extract does have pro-inflammatory activity, which may be relevant for the macrophage-pancreas interactions that underlie progression of diabetes.
To mimic (at least part of) the condition of infiltrated macrophages that secrete TNF-α, we administered TNF-α directly in the medium containing MIN6 cells, and assessed their viability following STZ administration, with and without ST leaf extract. While the used dose of TNF-α was too low to induce apoptosis of MIN6 cells (Stephens et al., 1999) either alone or in combination with STZ, the dose of TNF-α did limit the efficacy of ST extract at higher doses to counter the STZ toxicity in MIN6 cells. While more work needs to be done on this front (e.g., by applying different doses of TNF-α in this experimental setting), these data may be interpreted to indicate that ST leaf extract could sensitize TNF-α for its well-known apoptotic effects in the context of STZ treatment. TNF-α mediates its effects via nuclear factor (NF)-κB pathway, leading to deregulated processes involving activation of inhibitory κB proteins (IκB) kinase complex, and its activation has deleterious effects in pancreatic beta cells (Meyerovich et al., 2016).
Besides the above mentioned deleterious role of the NF-κB pathway, there are however also studies showing that NF-kB pathway is required for protection against cell death in vitro in pancreatic beta cells (Chang et al., 2003) as well as in vivo in diabetic animal models (Kim et al., 2007; Hofmeister-Brix et al., 2013). We have previously shown that inhibition of NF-κB activity leads to TNF-α-induced apoptosis of hepatocytes via inhibition of the induction of NF-κB dependent anti-apoptotic genes (Schoemaker et al., 2002). The protective effects of the ST extract against STZ-induced toxicity could also very well be mediated too via an NF-κB dependent anti-apoptotic pathway. To put this idea to the test, we investigated the protective effects of the ST extract against STZ-induced toxicity and employed 1) different doses of MG132, a compound that blocks a. o. the NF-kB pathway, and 2) measurement of expression levels of inhibitor of apoptosis protein 2 (cIAP2) (Hun et al., 2005; Stehlik et al., 1998), a direct target gene of NF-κB needed for its anti-apoptotic properties (Schoemaker et al., 2002; Zanotto-Filho et al., 2012). With the lowest dose of MG132, we did not influence the protective effect of the ST extract against STZ-induced toxicity, nor did it alter the expression level of cIAP2 in any of the conditions, implying that this dose of MG132 was simply too low to block an anti-apoptotic pathway potentially induced by ST. The same low dose of MG132, however, was able to markedly attenuate STZ-induced toxicity, implying that MG132 blocked an apoptotic pathway responsible for the STZ-induced toxicity. At this moment, we do not know whether or to what extent MG132 blocked specifically an apoptotic pathway via NF-kB activation or any alternative apoptotic pathway involving the proteasome (Zanotto-Filho et al., 2010; Stehlik et al., 1998), but it certainly is in line with the involvement of NF-kB in beta cell apoptosis following STZ treatment (Eldor et al., 2006). When increasing the dose of MG132, however, it was observed that the protective effects of ST extract to alleviate STZ-induced toxicity was lost, and this was associated with a strong decline in cIAP2 expression, indeed suggestive of ST’s dependency on the NF-kB pathway involving cIAP2 to engage its anti-apoptotic effect. Meanwhile, the higher doses of MG132 were no longer capable of blocking the STZ-induced toxicity itself, and in fact STZ became more toxic with increasing MG132 concentrations. Our data may be interpreted to indicate that STZ is inducing apoptotic pathways, but beta cells react to this by promoting anti-apoptotic responses, with the latter ones potentially aimed at counterbalancing the apoptotic ones.
One caution that needs to be raised here is that MG132 affects many pathways, and may be not very specific. For example, MG132 could also activate apoptosis via the JNK/p38 pathway (Zanotto-Filho et al., 2012), however administration of MG132 in a dose of 0.04 µM protected against STZ-induced toxicity, implying anti-apoptotic activation instead. In conditions where ST extract was no longer effective in protecting STZ-induced toxicity (i.e., when MG132 was dosed at 0.5 µM), we found that expression of cIAP was inhibited, indicative of blockade of an anti-apoptotic pathway downstream form NF-κB (Schoemaker et al., 2002; Zanotto-Filho et al., 2012). cIAP2 is probably not driving this anti-apoptotic effect of ST extract, as the gene expression of cIAP2 was not increased in ST-treated beta cells on top of the STZ-treatment, but a constitutive level of cIAP2 expression could be regarded permissive to these anti-apoptotic effects. We also cannot exclude other mechanisms involving the proteasome, which are blocked by MG132 too. There are several publications discussing the balance of both the NF-κB pathway and TNF-α in pro- and anti-apoptotic programming (Chang et al., 2003; Xu et al., 1998; Wang et al., 2008; Wang et al., 1998; Webster and Vucic, 2020). This balance is not only dependent on NF-κB, but also on caspase signaling (Wang et al., 1998). In this study, emphasis is put on downstream (anti- and pro-apoptotic) pathways from NF-κB, however NF-κB protein levels or changes in localization of NF-κB after treatment with ST leaf extract should further be investigated. In addition, to refine the therapeutic application of ST leaf extract, we also aim to further explore the interaction between the NF-κB pathway with other pathways (e.g., caspase signaling and JNK/p38 pathways) that could potentially be involved in the effects of ST leaf extract. Finally, another important factor by which the ST extract could have influenced cell number is that it stimulates proliferation. While also more work needs to be done on that front (e.g., by assessing the effect of the ST extract over a longer-period than 24 h in MIN6 cells), ST extract indeed caused a slight increase in beta-cell number when added alone, and any proliferative effect on top of its protective effects should be kept in mind too.
In summary, we show that ST extract (in part) protects MIN6 cells against STZ-induced cytotoxicity. This positive effect of ST extract is likely dependent on the integrity of the NF-κB pathway. Furthermore, ST extract could activate a low-grade inflammatory response by increasing the mRNA expression of COX2, iNOS and TNF-α in non-activated RAW cells in the dose range of 10–15 mg/mL. Finally, ST extract could increase expression of TNF-α and decrease COX2 in LPS-activated RAW cells. However, ST extract loses its protective effect against STZ-induced cytotoxicity when combined with TNF-α, which may be explained by a sensitizing effect of bioactive compounds in ST that render MIN6 cells sensitivity to canonical and non-canonical pathways involved in STZ-induced cell death, which apparently overpower the protective pathways induced by ST. Future studies should shed light on the bioactive compounds present in ST leaf extract that show their efficacy in pancreatic beta cell survival via potentially anti-apoptotic and/or proliferative actions (Figure 7).
Figure 7. Schematic representation of the effect of Sansevieria trifasciata leaf extract (ST) on pancreatic beta (MIN6) cells and macrophage-like (RAW) cells. ST extract protects pancreatic beta cells (dashed line) against streptozotocin (STZ)-induced toxicity (solid line). This positive effect of ST extract is dependent on the NF-κB pathway, as the addition of MG132 (blocker of the NF-κB pathway) counters the effect of ST extract. This could be the result of a “switch” from an anti-apoptotic program towards a pro-apoptotic program or a decrease in proliferation. Furthermore, ST extract increases expression of TNF-α potentially increasing TNF-α release (dotted line) in LPS-activated macrophages. However, TNF-α in combination with ST extract results in a loss of the effect of ST extract against STZ-induced toxicity. This may be explained by a sensitizing effect of bioactive compounds in ST that sensitize MIN6 cells to a sub-threshold dose of TNF-α that overpowers the positive effects of ST to counter STZ-induced toxicity.
The raw data supporting the conclusions of this article will be made available by the authors, without undue reservation.
Ethical approval was not required for the studies on animals in accordance with the local legislation and institutional requirements because only commercially available established cell lines were used.
CH: Conceptualization, Data curation, Formal Analysis, Investigation, Methodology, Project administration, Software, Validation, Visualization, Writing–original draft, Writing–review and editing. NQ: Conceptualization, Data curation, Formal Analysis, Investigation, Methodology, Project administration, Software, Validation, Visualization, Writing–original draft, Writing–review and editing. HG-P: Conceptualization, Data curation, Formal Analysis, Methodology, Visualization, Writing–original draft, Writing–review and editing. APV: Conceptualization, Funding acquisition, Resources, Supervision, Writing–original draft, Writing–review and editing. HM: Conceptualization, Funding acquisition, Investigation, Methodology, Project administration, Resources, Visualization, Writing–original draft, Writing–review and editing. GVD: Conceptualization, Data curation, Funding acquisition, Investigation, Methodology, Project administration, Validation, Visualization, Writing–original draft, Writing–review and editing.
The author(s) declare that financial support was received for the research and/or publication of this article. CH received a grant from Stichting de Cock – Hadders 2020-66 and the Ph. D scholarship of NQ was supported by the Directorate General of Higher Education from the Ministry of Education, Culture, Research and Technology, Republic of Indonesia and personal funding of GVD.
We thank dr. Janine Kruit of the Department of Pediatrics, University of Groningen, University Medical Center Groningen, Groningen, the Netherlands, for providing us the MIN6 cells, who received them from the Department of Pathology and Laboratory Medicine, Faculty of Medicine, the University of British Colombia, Vancouver, Canada; Manon Buist-Homan of the Department of Gastroenterology and Hepatology, University of Groningen, University Medical Center Groningen, Groningen, the Netherlands and Wanda Douwenga of the Department of Molecular Neuroscience; Groningen institute for Evolutionary Life Sciences; University of Groningen, Groningen, the Netherlands for technical support.
The authors declare that the research was conducted in the absence of any commercial or financial relationships that could be construed as a potential conflict of interest.
The author(s) declare that no Generative AI was used in the creation of this manuscript.
All claims expressed in this article are solely those of the authors and do not necessarily represent those of their affiliated organizations, or those of the publisher, the editors and the reviewers. Any product that may be evaluated in this article, or claim that may be made by its manufacturer, is not guaranteed or endorsed by the publisher.
The Supplementary Material for this article can be found online at: https://www.frontiersin.org/articles/10.3389/fphar.2025.1485952/full#supplementary-material
SUPPLEMENTARY FILE S1 | Letter from the head of the Tropical Biopharmaca Research Center.
SUPPLEMENTARY FILE S2 | Identification of the Sansevieria trifasciata plant.
SUPPLEMENTARY FILE S3 | ConPhyMP checklists Table 1.
SUPPLEMENTARY FILE S4 | ConPhyMP checklist Table 2a.
SUPPLEMENTARY FILE S5 | Full fingerprint of compound identification based on mass spectrometry.
SUPPLEMENTARY FILE S6 | Specific spectra and compound identification of compounds listed in Table 1.
SUPPLEMENTARY FILE S7 | Sequences used for real-time qPCR analysis.
SUPPLEMENTARY FILE S8 | The effect of Sansevieria extract on TNF-α expression in MIN6 cells.
Adriani, A., Noorhamdani, N., Ardyati, T., and Winarsih, S. (2022). Non-targeted screening with LC-HRMS and in-silico study on diabetic activity of ethyl acetate extract of Sanrego (Lunasia amara Blanco). Res. J. Pharm. Technol. 15 (3), 1077–1084. doi:10.52711/0974-360X.2022.00180
Ahn, C. H., Choi, E. H., Oh, T. J., and Cho, Y. M. (2020). Ileal transposition increases pancreatic β cell mass and decreases β cell senescence in diet-induced obese rats. Obes. Surg. 30 (5), 1849–1858. doi:10.1007/s11695-020-04406-6
Alencar, MVOB, Islam, M. T., Ali, E. S., Santos, J. V. O., Paz, M. F. C. J., Sousa, J. M. C., et al. (2018). Association of phytol with toxic and cytotoxic activities in an antitumoral perspective: a meta-analysis and systemic review. Anticancer Agents Med. Chem. 18 (13), 1828–1837. doi:10.2174/1871520618666180821113830
Al Za’Abi, M., Al, S. S., Al Suleimani, Y., Manoj, P., Nemmar, A., and Ali, B. H. (2018). Gum Acacia improves renal function and ameliorates systemic inflammation, oxidative and nitrosative stress in streptozotocin-induced diabetes in rats with adenine-induced chronic kidney disease. Cell Physiol. Biochem. 45 (6), 2293–2304. doi:10.1159/000488176
Bonora, E. (2008). Protection of pancreatic beta-cells: is it feasible? Nutr. Metab. Cardiovasc Dis. 18 (1), 74–83. doi:10.1016/j.numecd.2007.05.004
Cerf, M. E. (2013). Beta cell dysfunction and insulin resistance. Front. Endocrinol. (Lausanne) 4 (37), 37–12. doi:10.3389/FENDO.2013.00037
Chacón, M. R., Vendrell, J., Miranda, M., Ceperuelo-Mallafré, V., Megía, A., Gutiérrrez, C., et al. (2007). Different TNFalpha expression elicited by glucose in monocytes from type 2 diabetes mellitus patients. Atherosclerosis 194 (2), e18–e25. doi:10.1016/j.atherosclerosis.2006.12.011
Chang, I., Kim, S., Kim, J. Y., Cho, N., Kim, Y. H., Kim, H. S., et al. (2003). Nuclear factor kappaB protects pancreatic beta-cells from tumor necrosis factor-alpha-mediated apoptosis. Diabetes 52, 1169–1175. doi:10.2337/diabetes.52.5.1169
Chen, C., Ma, X., Yang, C., Nie, W., Zhang, J., Li, H., et al. (2018). Hypoxia potentiates LPS-induced inflammatory response and increases cell death by promoting NLRP3 inflammasome activation in pancreatic β cells. Biochem. Biophys. Res. Commun. 495 (4), 2512–2518. doi:10.1016/j.bbrc.2017.12.134
Cho, N. H., Shaw, J. E., Karuranga, S., Huang, Y., da Rocha Fernandes, J. D., Ohlrogge, A. W., et al. (2018). IDF Diabetes Atlas: global estimates of diabetes prevalence for 2017 and projections for 2045. Diabetes Res. Clin. Pract. 138, 271–281. doi:10.1016/j.diabres.2018.02.023
Eguchi, K., and Nagai, R. (2017). Islet inflammation in type 2 diabetes and physiology. J. Clin. Invest. 127 (1), 14–23. doi:10.1172/JCI88877
El-Din El-Hawary, S. S., El-Mahdy El-Tantawy, M., Rabeh, M. A., Ali, Z. Y., Albohy, A., and Fawaz, N. E. (2021). Sansevieria: an evaluation of potential cytotoxic activity in reference to metabolomic and molecular docking studies. Egypt J. Chem. 64 (2), 835–849. doi:10.21608/ejchem.2020.43384.2877
Eldor, R., Yeffet, A., Baum, K., Doviner, V., Amar, D., Ben-Neriah, Y., et al. (2006). Conditional and specific NF-kappaB blockade protects pancreatic beta cells from diabetogenic agents. Proc. Natl. Acad. Sci. U. S. A. 103 (13), 5072–5077. doi:10.1073/pnas.0508166103
Eleazu, C. O., Eleazu, K. C., Chukwuma, S., and Essien, U. N. (2013). Review of the mechanism of cell death resulting from streptozotocin challenge in experimental animals, its practical use and potential risk to humans. J. Diabetes Metab. Disord. 121 (1), 60–67. doi:10.1186/2251-6581-12-60
Elmore, S. (2007). Apoptosis: a review of programmed cell death. Toxicol. Pathol. 35 (4), 495–516. doi:10.1080/01926230701320337
Erejuwa, O. O., Sulaiman, S. A., and Ab Wahab, M. S. (2012). Honey: a novel antioxidant. Molecules 17 (4), 4400–4423. doi:10.3390/molecules17044400
Furman, B. L. (2015). Streptozotocin-induced diabetic models in mice and rats. Curr. Protoc. Pharmacol. 1(70):5.47.1–5.47.5. doi:10.1002/0471141755.ph0547s70
Gao, Y., Parker, G. J., and Hart, G. W. (2000). Streptozotocin-induced beta-cell death is independent of its inhibition of O-GlcNAcase in pancreatic Min6 cells. Arch. Biochem. Biophys. 383 (2), 296–302. doi:10.1006/ABBI.2000.2094
Goldfine, A. B., Fonseca, V., and Shoelson, S. E. (2011). Therapeutic approaches to target inflammation in type 2 diabetes. Clin. Chem. 57 (2), 162–167. doi:10.1373/clinchem.2010.148833
González-Ponce, H. A., Rincón-Sánchez, A. R., Jaramillo-Juárez, F., and Moshage, H. (2018). Natural dietary pigments: potential mediators against hepatic damage induced by over-the-counter non-steroidal anti-inflammatory and analgesic drugs. Nutrients 10 (2), 117. doi:10.3390/nu10020117
Heinrich, M., Jalil, B., Abdel-Tawab, M., Echeverria, J., Kulić, Ž., McGaw, L. J., et al. (2022). Best Practice in the chemical characterisation of extracts used in pharmacological and toxicological research-The ConPhyMP-Guidelines. Front. Pharmacol. 13, 953205. doi:10.3389/fphar.2022.953205
Hofmeister-Brix, A., Lenzen, S., and Baltrusch, S. (2013). The ubiquitin-proteasome system regulates the stability and activity of the glucose sensor glucokinase in pancreatic β-cells. Biochem. J. 456, 173–184. doi:10.1042/BJ20130262
Hsieh, P. S., Jin, J. S., Chiang, C. F., Chan, P. C., Chen, C. H., and Shih, K. C. (2009). COX-2-mediated inflammation in fat is crucial for obesity-linked insulin resistance and fatty liver. Obes. (Silver Spring) 17 (6), 1150–1157. doi:10.1038/oby.2008.674
Hun, S. K., Kim, S., and Lee, M. S. (2005). IFN-gamma sensitizes MIN6N8 insulinoma cells to TNF-alpha-induced apoptosis by inhibiting NF-kappaB-mediated XIAP upregulation. Biochem. Biophys. Res. Commun. 336 (3), 847–853. doi:10.1016/j.bbrc.2005.08.183
Karin, M., and Lin, A. (2002). NF-kappaB at the crossroads of life and death. Nat. Immunol. 3 (3), 221–227. doi:10.1038/ni0302-221
Kim, S., Millet, I., Kim, H. S., Kim, J. Y., Han, M. S., Lee, M. K., et al. (2007). NF-κB prevents β cell death and autoimmune diabetes in NOD mice. Proc. Natl. Acad. Sci. U. S. A. 104 (6), 1913–1918. doi:10.1073/pnas.0610690104
Kolb, H., and Eizirik, D. L. (2012). Resistance to type 2 diabetes mellitus: a matter of hormesis. Nat. Rev. Endocrinol. 8 (3), 183–192. doi:10.1038/nrendo.2011.158
Kumar, P., Nagarajan, A., and Uchil, P. D. (2018). Analysis of cell viability by the MTT assay. Cold Spring Harb. Protoc. 2018 (6), pdb.prot095505–471. doi:10.1101/PDB.PROT095505
Lenzen, S. (2008). The mechanisms of alloxan- and streptozotocin-induced diabetes. Diabetologia 51 (2), 216–226. doi:10.1007/s00125-007-0886-7
Li, F. S., and Weng, J. K. (2017). Demystifying traditional herbal medicine with modern approaches. Nat. Plants 31 (3), 17109. doi:10.1038/nplants.2017.109
Lontoc, S. M. H., Soriano, C. F., Comia, S. A. M. M., Hernandez, A., and Dumaoal, O. S. R. In vitro antioxidant activity and total phenolic content of Sansevieria trifasciata (Snake plant) crude ethanolic and aqueous leaf extracts. Asia Pac. J. Allied Heal Sci. 218AD, 35–58.
Meyerovich, K., Ortis, F., Allagnat, F., and Cardozo, A. K. (2016). Endoplasmic reticulum stress and the unfolded protein response in pancreatic islet inflammation. J. Mol. Endocrinol. 57 (1), R1–R17. doi:10.1530/JME-15-0306
Mimaki, Y., Inoue, T., Kuroda, M., and Sashida, Y. (1996). Steroidal saponins from Sansevieria trifasciata. Phytochemistry 43 (6), 1325–1331. doi:10.1016/S0031-9422(96)00397-4
Miyazaki, J. I., Araki, K., Yamato, E., Ikegami, H., Asano, T., Shibasaki, Y., et al. (1990). Establishment of a pancreatic beta cell line that retains glucose-inducible insulin secretion: special reference to expression of glucose transporter isoforms. Endocrinology 127 (1), 126–132. doi:10.1210/ENDO-127-1-126
Nemecz, M., Constantin, A., Dumitrescu, M., Alexandru, N., Filippi, A., Tanko, G., et al. (2019). The distinct effects of palmitic and oleic acid on pancreatic beta cell function: the elucidation of associated mechanisms and effector molecules. Front. Pharmacol. 9, 1554. doi:10.3389/fphar.2018.01554
Patel, D. K., Prasad, S. K., Kumar, R., and Hemalatha, S. (2012). An overview on antidiabetic medicinal plants having insulin mimetic property. Asian Pac J. Trop. Biomed. 2 (4), 320–330. doi:10.1016/S2221-1691(12)60032-X
Qomariyah, N., Sarto, M., and Pratiwi, R. (2012). Antidiabetic effects of a decoction of leaves of Sansevieria trifasciata in alloxan-induced diabetic white rats (Rattus norvegicus L.). ITB J. Sci. 44A (4), 308–316. doi:10.5614/itbj.sci.2012.44.4.2
Rachdaoui, N. (2020). Insulin: the friend and the foe in the development of type 2 diabetes mellitus. Int. J. Mol. Sci. Sci. 21 (5), 1770. doi:10.3390/ijms21051770
Raschke, W. C., Baird, S., Ralph, P., and Nakoinz, I. (1978). Functional macrophage cell lines transformed by Abelson leukemia virus. Cell 15 (1), 261–267. doi:10.1016/0092-8674(78)90101-0
Raslan, M. A., Abdel-Rahman, R. F., Fayed, H. M., Ogaly, H. A., and Tahere, R. F. (2021). Metabolomic profiling of Sansevieria trifasciata hort ex. Prain leaves and roots by HPLC-PAD-ESI/MS and its hepatoprotective effect via activation of the NRF2/ARE signaling pathway in an experimentally induced liver fibrosis rat model. Egypt J. Chem. 64 (11), 0–6671. doi:10.21608/EJCHEM.2021.78970.3877
Saini, K. S., Thompson, C., Winterford, C. M., Walker, N. I., and Cameron, D. P. (1996). Streptozotocin at low doses induces apoptosis and at high doses causes necrosis in a murine pancreatic beta cell line, INS-1. Biochem. Mol. Biol. Int. 39 (6), 1229–1236. doi:10.1080/15216549600201422
Schoemaker, M. H., Ros, J. E., Homan, M., Trautwein, C., Liston, P., Poelstra, K., et al. (2002). Cytokine regulation of pro- and anti-apoptotic genes in rat hepatocytes: NF-kappaB-regulated inhibitor of apoptosis protein 2 (cIAP2) prevents apoptosis. J. Hepatol. 36 (6), 742–750. doi:10.1016/S0168-8278(02)00063-6
Shakib, Z., Shahraki, N., Razavi, B. M., and Hosseinzadeh, H. (2019). Aloe vera as an herbal medicine in the treatment of metabolic syndrome: a review. Phyther Res. 33 (10), 2649–2660. doi:10.1002/ptr.6465
Sheehy, D. F., Quinnell, S. P., and Vegas, A. J. (2019). Targeting type 1 diabetes: selective approaches for new therapies. Biochemistry 58 (4), 214–233. doi:10.1021/ACS.BIOCHEM.8B01118
Stehlik, C., De Martin, R., Kumabashiri, I., Schmid, J. A., Binder, B. R., and Lipp, J. (1998). Nuclear factor (NF)-kappaB-regulated X-chromosome-linked iap gene expression protects endothelial cells from tumor necrosis factor alpha-induced apoptosis. J. Exp. Med. 188 (1), 211–216. doi:10.1084/jem.188.1.211
Stephens, L. A., Thomas, H. E., Ming, L., Darwiche, M. G. R., Volodin, L., Kay, T. W. H., et al. (1999). Tumor necrosis factor-alpha-activated cell death pathways in NIT-1 insulinoma cells and primary pancreatic beta cells. Endocrinology 140 (7), 3219–3227. doi:10.1210/ENDO.140.7.6873
Virtanen, J. K., Tuomainen, T. P., and Voutilainen, S. (2020). Dietary intake of choline and phosphatidylcholine and risk of type 2 diabetes in men: the kuopio ischaemic heart disease risk factor study. Eur. J. Nutr. 59 (8), 3857–3861. doi:10.1007/S00394-020-02223-2
Wang, C. Y., Mayo, M. W., Korneluk, R. G., Goeddel, D. V. B. A. J., and Baldwin, A. S. (1998). NF-kappaB antiapoptosis: induction of TRAF1 and TRAF2 and c-IAP1 and c-IAP2 to suppress caspase-8 activation. Science 281 (5383), 1680–1683. doi:10.1126/science.281.5383.1680
Wang, J., Zhao, F., Brouwer, L. A., Buist-Homan, M., Wolters, J. C., Moshage, H., et al. (2024). Collagen-rich liver-derived extracellular matrix hydrogels augment survival and function of primary rat liver sinusoidal endothelial cells and hepatocytes. Int. J. Biol. Macromol. 278 (Pt 2), 134717. doi:10.1016/J.IJBIOMAC.2024.134717
Wang, L., Du, F., and Wang, X. (2008). TNF-Α induces two distinct caspase-8 activation pathways. Cell 133 (4), 693–703. doi:10.1016/j.cell.2008.03.036
Ward, M. G., Li, G., Barbosa-Lorenzi, V. C., and Hao, M. (2017). Stigmasterol prevents glucolipotoxicity induced defects in glucose-stimulated insulin secretion. Sci. Rep. 7 (1), 9536. doi:10.1038/s41598-017-10209-0
Webster, J. D., and Vucic, D. (2020). The balance of TNF mediated pathways regulates inflammatory cell death signaling in healthy and diseased tissues. Front. Cell Dev. Biol. 8, 365. doi:10.3389/fcell.2020.00365
World Health Organization (WHO) (2013). WHO traditional medicine strategy 2014-2023. Geneva, Switzerland: World Health Organization. Available online at: https://apps.who.int/iris/handle/10665/92455.
Xu, L., Kitade, H., Ni, Y., and Ota, T. (2015). Roles of chemokines and chemokine receptors in obesity-associated insulin resistance and nonalcoholic fatty liver disease. Biomolecules 5, 1563–1579. doi:10.3390/biom5031563
Xu, Y., Bialik, S., Jones, B. E., Iimuro, Y., Kitsis, R. N., Srinivasan, A., et al. (1998). NF-kappaB inactivation converts a hepatocyte cell line TNF-alpha response from proliferation to apoptosis. Am. J. Physiol. Cell Physiol. 275 (4), C1058–C1066. doi:10.1152/ajpcell.1998.275.4.c1058
Yoshinari, O., and Igarashi, K. (2011). Anti-diabetic effect of pyroglutamic acid in type 2 diabetic Goto-Kakizaki rats and KK-Ay mice. Br. J. Nutr. 106 (7), 995–1004. doi:10.1017/S0007114511001279
Zanotto-Filho, A., Braganhol, E., Oliveira Battastini, A. M., and Fonseca Moreira, J. C. (2012). Proteasome inhibitor MG132 induces selective apoptosis in glioblastoma cells through inhibition of PI3K/Akt and NFkappaB pathways, mitochondrial dysfunction, and activation of p38-JNK1/2 signaling. Invest. New Drugs 30 (6), 2252–2262. doi:10.1007/s10637-012-9804-z
Zanotto-Filho, A., Delgado-Cañedo, A., Schröder, R., Becker, M., Klamt, F., and Moreira, J. C. F. (2010). The pharmacological NFkappaB inhibitors BAY117082 and MG132 induce cell arrest and apoptosis in leukemia cells through ROS-mitochondria pathway activation. Cancer Lett. 288 (2), 192–203. doi:10.1016/j.canlet.2009.06.038
Zhang, Y. Y., Yao, Y. Da, Chen, F., Guo, X., Kang, J. L., Huang, Y. F., et al. (2022). (9S,13R)-12-oxo-phytodienoic acid attenuates inflammation by inhibiting mPGES-1 and modulating macrophage polarization via NF-κB and Nrf2/HO-1 pathways. Pharmacol. Res. 182, 106310. doi:10.1016/J.PHRS.2022.106310
Keywords: Sansevieria trifasciata, diabetes, streptozotocin, cell viability, TNF-α, NF-κB
Citation: Hoornenborg CW, Qomariyah N, González-Ponce HA, Van Beek AP, Moshage H and Van Dijk G (2025) Dracaena trifasciata (Prain) Mabb leaf extract protects MIN6 pancreas-derived beta cells against the diabetic toxin streptozotocin: role of the NF-κB pathway. Front. Pharmacol. 16:1485952. doi: 10.3389/fphar.2025.1485952
Received: 25 August 2024; Accepted: 13 March 2025;
Published: 16 April 2025.
Edited by:
Michael Heinrich, University College London, United KingdomReviewed by:
Ahmad Khusairi Azemi, Universiti Malaysia Terengganu, MalaysiaCopyright © 2025 Hoornenborg, Qomariyah, González-Ponce, Van Beek, Moshage and Van Dijk. This is an open-access article distributed under the terms of the Creative Commons Attribution License (CC BY). The use, distribution or reproduction in other forums is permitted, provided the original author(s) and the copyright owner(s) are credited and that the original publication in this journal is cited, in accordance with accepted academic practice. No use, distribution or reproduction is permitted which does not comply with these terms.
*Correspondence: Gertjan Van Dijk, Z2VydGphbi52YW4uZGlqa0BydWcubmw=
†These authors have contributed equally to this work and share first authorship
Disclaimer: All claims expressed in this article are solely those of the authors and do not necessarily represent those of their affiliated organizations, or those of the publisher, the editors and the reviewers. Any product that may be evaluated in this article or claim that may be made by its manufacturer is not guaranteed or endorsed by the publisher.
Research integrity at Frontiers
Learn more about the work of our research integrity team to safeguard the quality of each article we publish.