- 1Department of Neurology, The Affiliated Lihuili Hospital of Ningbo University, Ningbo, Zhejiang, China
- 2Neuroscience Medical Center, Ningbo Medical Center Lihuili Hospital, Ningbo University, Ningbo, Zhejiang, China
Parkinson’s disease (PD), as a refractory neurological disorder with complex etiology, currently lacks effective therapeutic agents. Natural products (NPs), derived from plants, animals, or microbes, have shown promising effects in PD models through their antioxidative and anti-inflammatory properties, as well as the enhancement of mitochondrial homeostasis and autophagy. The misfolding and deposition of α-Synuclein (α-Syn), due to abnormal overproduction and impaired clearance, being central to the death of dopamine (DA) neurons. Thus, inhibiting α-Syn misfolding and aggregation has become a critical focus in PD discovery. This review highlights NPs that can reduce α-Syn aggregation by preventing its overproduction and misfolding, emphasizing their potential as novel drugs or adjunctive therapies for PD treatment, thereby providing further insights for clinical translation.
1 Introduction
Parkinson’s disease (PD) is a complex progressive neurodegenerative disorder characterized by motor and non-motor symptom (NMS), which may result from genetic and environmental factors. Both genetic and sporadic PD have the loss of dopamine (DA) neurons in substantia nigra (SN) and the presence of Lewy bodies (Bloem et al., 2021; Moore et al., 2005). The primary clinical features include classic dyskinesia symptoms caused by DA loss in the basal ganglia, such as bradykinesia, muscle rigidity, resting tremor, postural instability, and speech disorders. Additionally, NMS arises from the involvement of multiple brain areas and includes swallowing difficulties, drooling, cognitive and emotional disturbances, gastrointestinal dysfunction, and sleep disorders, which appears at all stages of the disease. Although diagnosis primarily focuses on motor symptoms, NMS is also significant. Studies have shown that these symptoms can persist for over 10 years before dyskinesia appears, leading to a decline in patients’ quality of life, with many severely affected by these NMS (Postuma et al., 2012a; Schapira et al., 2017; Balestrino and Martinez-Martin, 2017).
PD is currently the second most common age-dependent neurodegenerative disorder. By 2040, the number of PD patients is expected to reach 17 million, suggesting a “PD pandemic” (Dorsey et al., 2018). Despite decades of research, PD remains incurable (Bloem et al., 2021). Current treatments, including levodopa, DA agonists, catechol-O-methyltransferase (COMT) inhibitors, monoamine oxidase-B (MAO-B) inhibitors, and anticholinergics, only alleviate symptoms without slowing disease progression. Additionally, their effectiveness diminishes over time (Fox et al., 2018). These medications can cause significant clinical side effects, such as motor complications, cognitive and emotional disturbances, psychiatric disorders, and adverse neurological reactions (Bonifácio et al., 2007; Thanvi and Lo, 2004). Therefore, there is an urgent need to develop new therapies that can effectively slow the progression of debilitating motor symptoms.
PD can manifest in two forms: familial/genetic and sporadic. Familial/genetic PD is closely associated with gene mutations, inherited through autosomal dominant or recessive patterns. Mutations in genes such as SNCA, LRRK2, VPS35, EIF4G1, and DNAJC13 are linked to autosomal dominant PD, while PRKN, PINK1, and DJ-1 mutations are associated with autosomal recessive forms. Familial/genetic PD typically presents at an earlier age and progresses more rapidly, with Parkin mutations being the most common genetic factor in familial cases. However, the majority of PD cases are sporadic, with no definitive causative factors identified. Lifestyle, adverse environmental exposures, and advanced age are believed to be closely related to the onset of sporadic PD (Costa et al., 2022). For example, a history of psychiatric disorders like anxiety or depression, pesticide exposure, head trauma, rural living, beta-blocker use, and consumption of well water can increase the risk of developing PD. Conversely, factors like smoking, coffee consumption, the use of non-steroidal anti-inflammatory drugs (NSAIDs) and calcium channel blockers, and alcohol consumption are negatively correlated with PD incidence (Noyce et al., 2012). While PD caused by gene mutations is relatively rare, both familial/genetic and sporadic forms present with classic symptoms of bradykinesia, rigidity, and resting tremor. Both forms can also manifest atypical NMS, with neuropsychiatric symptoms potentially more prevalent in familial/genetic PD (Chao et al., 2015).
The hallmark pathological feature of PD is the degeneration of dopaminergic neurons in the SN and striatum. The SN is typically the most severely affected region, with moderate to severe DA neurons loss in this area potentially contributing to the development of bradykinesia. Moreover, the loss of DA neurons extends beyond the SN, affecting multiple brain regions in cases with longer disease duration. These regions include the basal ganglia, hypothalamus, locus coeruleus, medullary tegmentum, hippocampus, temporal lobe, and the pons (Dickson, 2012).
Another crucial pathological feature of PD is the deposition of Lewy bodies, primarily composed of insoluble aggregates formed by misfolded α-Synuclein (α-Syn). The α-Syn aggregation hypothesis has gained significant attention in recent years. Notably, the toxic intermediate oligomers and protofibrils formed during aberrant α-Syn aggregation are particularly detrimental, with soluble protofibrils exhibiting greater toxicity than insoluble mature fibrils, ultimately leading to neurotoxicity (Lashuel et al., 2013; Lücking and Brice, 2000). These toxic intermediates can trigger a cascade of events, including mitochondrial dysfunction, neuroinflammation, neuronal deformation, and ferroptosis, all of which are closely linked to the pathogenesis of PD. Furthermore, they can disrupt synaptic transmission, impair organelle function and cytoskeleton integrity, compromise membrane structure, and disrupt the blood-brain barrier (BBB) (Pacheco et al., 2015; Burré et al., 2018).
The α-Syn aggregates primarily propagate and spread in a “prion-like” manner. Additionally, they can disseminate through tunneling nanotubes (Abounit et al., 2016), exosomes (Grey et al., 2015), and other mechanisms, leading to the widespread deposition of these pathological proteins throughout the brain, particularly in the neocortex, hippocampus, striatum, thalamus, and cerebellum (Burré et al., 2018). Importantly, the abnormal aggregation of α-Syn is not confined to the brain, it has also been observed in the spinal cord and peripheral nervous system, including the sympathetic ganglia, vagus nerve, cardiac nerves, and gastrointestinal system (Beach et al., 2010).
The autophagy-lysosome pathway (ALP) and the ubiquitin-proteasome system (UPS) are the two primary mechanisms for degrading misfolded and aggregated α-Syn proteins. In addition to aggregation driven by structural changes, functional impairments in these degradation pathways contribute to a reduced clearance rate of α-Syn aggregates (Senkevich and Gan-Or, 2020). Interestingly, α-Syn aggregates can also exert inhibitory effects on their own degradation pathways, creating a vicious cycle of aggregation due to their continuous formation, accumulation, and impaired clearance (Yang B. et al., 2023; Park et al., 2023). Therefore, targeting the inhibition of aberrant α-Syn aggregation and fibrillation, along with promoting the breakdown of existing aggregates to mitigate cellular toxicity, represents a promising therapeutic strategy for PD. To date, a limited number of drugs have been developed with this approach, including Anle138b (patent: WO2010000372) (Wagner et al., 2013), NPT200-11 (patent: CN102725284) (Price et al., 2018), and UCB0599 (the R-enantiomer of NPT200-11) (patent: CN110198938) (Smit et al., 2022). These aggregation inhibitors have demonstrated neuroprotective properties in related trials, suggesting that targeting α-Syn aggregation is a viable therapeutic avenue for PD.
Natural products (NPs) are increasingly recognized as important and valuable resources. To date, the development of NPs as emerging therapeutic agents remains a significant area of research in disease treatment, particularly for neurodegenerative disorders like PD. Extensive research has shown the potential of NPs to modulate oxidative stress and mitochondrial damage in PD, with significant discoveries emerging from natural plant-derived products. However, there is a relative paucity of research focusing on the potential of NPs to inhibit α-Syn aggregation, a key pathological hallmark of PD. Therefore, this review aims to bridge this gap by presenting evidence supporting the role of NPs in PD treatment through the inhibition of α-Syn aggregation. The findings discussed herein highlight promising lead compounds for the future development of novel α-Syn-targeted therapeutics and lay a foundation for further exploration of NPs as a therapeutic avenue for PD intervention.
2 The structure and aggregation of α-Syn
2.1 Structure and aggregation
α-Syn is a small, highly aggregation-prone protein composed of 140 amino acids. It can be divided into three distinct regions: the N-terminal region (residues 1–60), the nonamyloid-β component (NAC) region (residues 61–95), which forms the non-amyloid β component of amyloid plaques, and the C-terminal region (residues 96–140) (Burré et al., 2018). Notably, mutations in the α-Syn gene are linked to the development of familial PD, with A30P, E46K, and A53T being the most extensively studied mutation sites (Polymeropoulos et al., 1997; Krüger et al., 1998; Zarranz et al., 2004) (Figure 1).
(1) N-terminal region: This part of α-Syn is rich in amphipathic amino acid residues and contains seven imperfect repeat sequences along with a conserved sequence KTKEGV. This conserved sequence plays a role in mitochondrial function regulation and has been implicated in mitochondrial dysfunction (Hui, 2020; Burré et al., 2018). Acetylation of the N-terminal can enhance its affinity for lipid membranes (LM) and stabilize its helical structure (Figure 1). Many α-Syn mutation sites reside within the N-terminal region, leading to varying degrees of alteration in membrane binding affinity and aggregation propensity. For instance, the A30P, G51D, and A53E mutations decrease LM affinity, while the E46K mutation increases it. Additionally, the A30P, E46K, and A53T mutations may promote α-Syn aggregation, whereas the G51D mutation hinders it (Dikiy and Eliezer, 2014). Upon binding to LMs, the N-terminal structure of α-Syn undergoes a conformation change from a random coil to a helical structure (Davidson et al., 1998).
(2) NAC region: This region of α-Syn was first identified in the brain senile plaques of Alzheimer’s disease patients. This region exhibits strong hydrophobicity and is crucial for the stabilization and aggregation of α-Syn (Han et al., 1995; Uéda et al., 1993). The NAC region encompasses the minimal sequence necessary for α-Syn aggregation. However, recent studies suggest that specific sequences outside the NAC region also influence α-Syn aggregation, indicating that multiple regions may work in concert to regulate this process (Tripathi, 2020; El-Agnaf and Irvine, 2002) (Figure 1).
(3) C-terminal region: This part of α-Syn is rich in acidic amino acid residues, particularly glutamic acid, enabling it to interact with a diverse array of proteins. Post-translational modifications of α-Syn, including phosphorylation, acetylation, ubiquitination, glycosylation, and nitration, likely play critical roles in regulating its misfolding, abnormal aggregation, and neurotoxicity (Burré et al., 2018). Furthermore, the C-terminal structure is essential for the chaperone activity of α-Syn. Notably, the deletion of the C-terminal region abolishes this chaperone activity, thereby promoting α-Syn aggregation (Kim et al., 2002) (Figure 1).
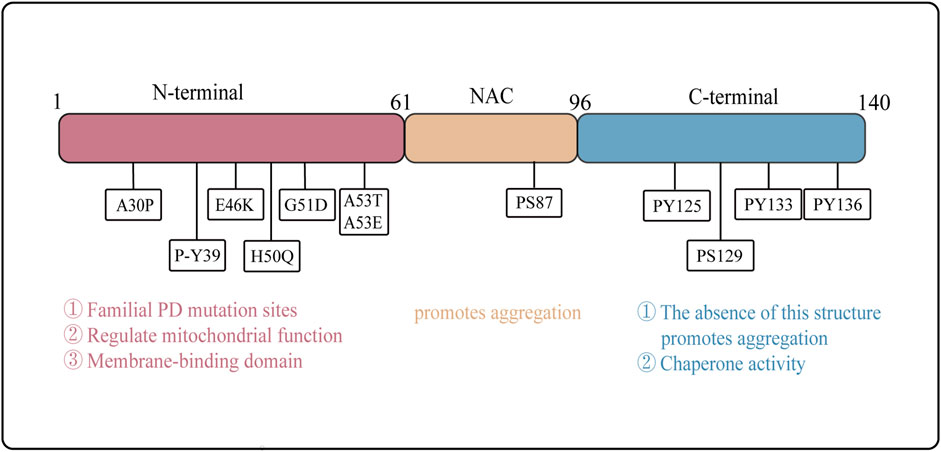
Figure 1. Structure and Function of α-Syn Domains. The structural of α-Syn is illustrated, emphasizing the N-terminal region (residues 1–60), the NAC region (residues 61–95), and the C-terminal region (residues 96–140). Arrows indicate the sites of familial Parkinson’s disease mutations. The distinct functions of the N-terminal, NAC region, and C-terminal domains are delineated, highlighting their respective roles in the protein’s overall activity and pathology.
α-Syn aggregation follows the general kinetics of protein aggregation, encompassing distinct phases: nucleation (lag phase), elongation, growth, aggregation/fibrillation, and equilibrium/saturation (Xiong et al., 2010; Almeida and Brito, 2020). In solution, α-Syn exists primarily as an unstructured random coil. However, it adopts α-helical structures upon binding to lipids and forms β-sheet-rich structures during aggregation. Under normal physiological conditions, α-Syn exists predominantly in monomeric or tetrameric forms, characterized by stable helical structures that confer resistance to aggregation (Bartels et al., 2011; Burré et al., 2018; Xiong et al., 2010). However, under pathological conditions, α-Syn undergoes misfolding, leading to conformational changes that drive abnormal aggregation. This process results in the formation of various species, including oligomers, protofibrils, and mature aggregates or fibrils, all of which can be cytotoxic to neurons and contribute to neuronal death. Notably, recent studies suggest that intermediate α-Syn oligomers, formed during the aggregation process, may exhibit greater toxicity than mature aggregates and fibrils (Lücking and Brice, 2000) (Figure 2A).
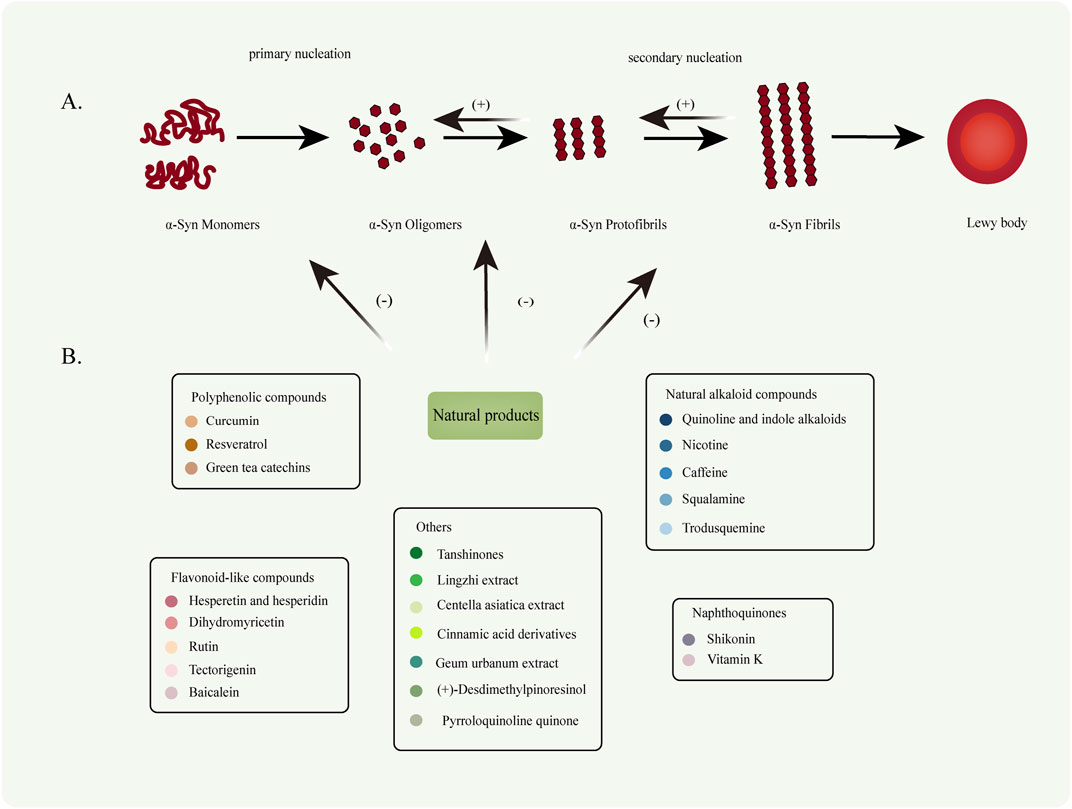
Figure 2. (A) Under pathological conditions, naturally disordered α-Syn monomers abnormally aggregate to form oligomers (primary nucleation). These oligomers subsequently extend to generate protofibrils and mature fibrils (secondary nucleation). (B) Various types of NPs can inhibit the conversion of α-Syn monomers into oligomers, thereby suppressing oligomer fibrillation and preventing fibril formation. Furthermore, NPs facilitate the degradation of fibrillar products.
2.2 The impact of α-Syn aggregation
The toxicity of α-Syn to dopaminergic neurons is multifaceted. Mitochondria, the primary source of reactive oxygen species (ROS) within cells, are particularly vulnerable to α-Syn aggregation. This aggregation is believed to induce oxidative stress and mitochondrial dysfunction, both of which are critical factors contributing to the deformation and death of DA neurons in PD. Studies have revealed that α-Syn negatively impacts mitochondria through various mechanisms including binding to mitochondrial respiratory chain complexes, interacting with the mitochondrial permeability transition pore, interfering with mitochondrial protein import, and disrupting mitochondrial quality control (Sohrabi et al., 2023) (Figure 3A). Moreover, α-Syn aggregation disrupts the ALP and UPS, the two major protein degradation pathways in cells, thereby impairing intracellular protein transport and the clearance or damaged proteins (Xilouri et al., 2013a).
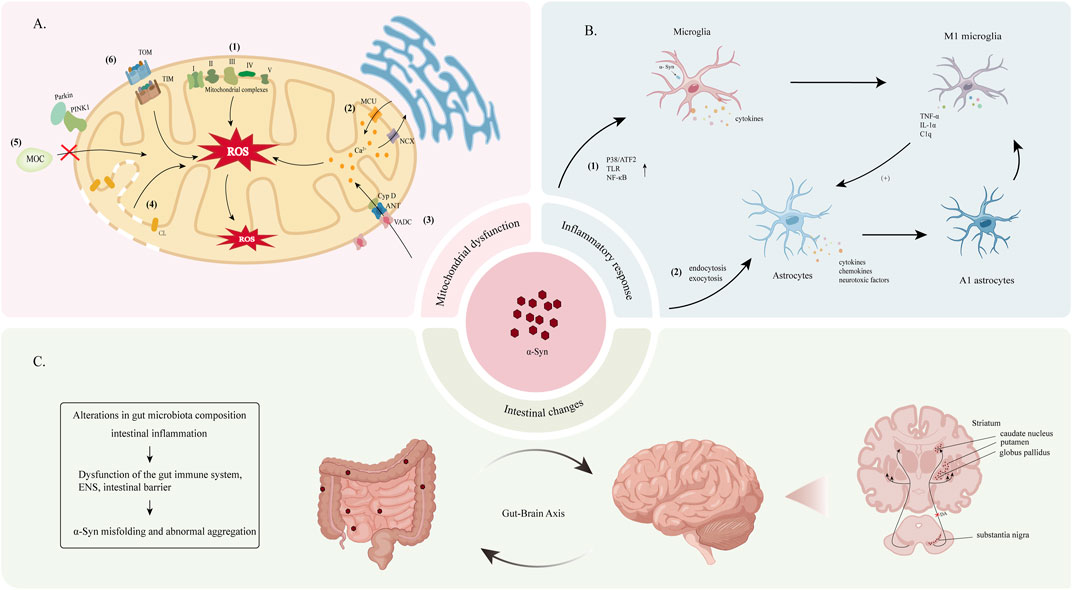
Figure 3. (A) The underlying mechanism of α-Syn-induced mitochondrial dysfunction leads to increased intracellular ROS. (1) α-Syn disrupts the activity of mitochondrial electron transport chain complexes, including complex I (Luth et al., 2014), III (Ellis et al., 2005), IV (Danyu et al., 2019; Elkon et al., 2002) and V (Ludtmann et al., 2018), with the most pronounced damage observed in complex I. (2) The accumulation of α-Syn affects the stability of the endoplasmic reticulum-mitochondria association, leading to impaired Ca2+ transport and subsequent the disruption of mitochondrial calcium homeostasis (Cali et al., 2012; Paillusson et al., 2017). (3) Fibrillated products of α-Syn interact with components of the mitochondrial permeability transition pore, such as the voltage-dependent anion channel (VDAC), adenine nucleotide translocator (ANT), and mitochondrial matrix protein cyclophilin D (CypD), This interaction activates the mitochondrial permeability transition pore and altering mitochondrial permeability (Torpey et al., 2020; Halestrap, 2009; Lu et al., 2013; Zhu et al., 2011). (4) Cardiolipin (CL)-rich environments enhance the interaction between α-Syn and mitochondria, increasing mitochondrial membrane permeability (Ghio et al., 2019). (5) α-Syn interacts with Parkin and PINK1, disrupting the mitochondrial quality control (MQC) pathway (Thorne and Tumbarello, 2022). (7) α-Syn binds to the TOM and TIM proteins on the outer mitochondrial membrane (OMM) and inner mitochondrial membrane (IMM), interfering with the mitochondrial protein import mechanism (Thorne and Tumbarello, 2022). (B) Pathological α-Syn activates microglia and astrocytes, leading to sustained neuroinflammation and neurotoxicity. (1) Pathological α-Syn enhances the activation of p38/ATF2, TLR, and NF-κB in microglia, and promoting their transition to the M1 phenotype. (2) α-Syn enters and activates astrocytes through endocytosis and exocytosis (Lee et al., 2010). Activated astrocytes produce cytokines, chemokines, and toxic factors, which further enhance microglial activation. M1-type microglia release tumor necrosis factor-alpha (TNF-α), interleukin-1α (IL-1α), and complement component 1q (C1q), promoting the formation of A1-type astrocytes. (C) Overexpression and abnormal aggregation of α-Syn in the gastrointestinal tract can lead to its transport to the brain via the gut-brain axis, resulting in accumulation within the CNS. α-Syn is predominantly deposited in several brain regions, with the SN and striatum being the most commonly affected areas.
Under physiological conditions, microglia and astrocytes function as key central nervous system (CNS) immune and supporting cells, respectively. However, in the presence of pathological α-Syn, microglial proliferation is promoted through mechanisms such as the activation of Toll-like receptors (TLR) and the p38/ATF2 and nuclear factor κB (NF-κB) signaling pathways. This activation leads to the production of various inflammatory factors and ROS, ultimately contributing to DA neurons dysfunction and death (Kam et al., 2020). Furthermore, the accumulation of α-Syn in astrocytes induces the production of proinflammatory factors, chemokines, and neurotoxic factors, further propagating microglial activation (Miyazaki and Asanuma, 2020). (Figure 3B).
One theory proposes that α-Syn aggregates activate microglia to differentiate into a pro-inflammatory phenotype (M1 phenotype). These activated M1 microglia secrete factors such as IL-1α, TNF-α, and C1q, which, in turn, induce astrocytes to transform into a neurotoxic phenotype (A1 phenotype) (Liddelow et al., 2017). Both M1 microglia and A1 astrocytes release inflammatory factors and chemokines that exacerbate neuroinflammation, potentially enhancing the aggregation and spread of α-Syn (Figure 3B). Concurrently, α-Syn activates pericytes, leading to BBB disruption and the infiltration of CD4+ and CD8+ T lymphocytes, further mediating neuroimmune responses (Delgado-Minjares et al., 2021). In this context, the chronic activation of microglia and astrocytes perpetuates neuroinflammation and neurotoxic responses, contributing to the pathogenesis of PD.
The gut-brain hypothesis posits that the pathological processes of PD may originate in the gut and subsequently spread to the brain, a concept supported by evidence from rat models (Holmqvist et al., 2014). α-Syn may induce alterations in gut microbiota composition and the presence of intestinal inflammation, leading to dysfunction within the gut immune system, enteric nervous system (ENS), and intestinal barrier. Furthermore, the activation of enteric glial cells, increased intestinal permeability, and oxidative stress can elevate α-Syn expression levels in the gut, potentially leading to its misfolding and abnormal aggregation. Moreover, chronic peripheral inflammation may compromise BBB integrity, facilitating the transport of α-Syn from the gut to the CNS (Tan et al., 2022; Houser and Tansey, 2017) (Figure 3C).
3 PD models
3.1 Neurotoxin-induced models
3.1.1 6-Hydroxydopamine (6-OHDA)
6-OHDA shares structural similarities with DA and norepinephrine, rendering it selectively toxic to catecholaminergic neurons. Due to its inability to cross the BBB, 6-OHDA typically requires direct, targeted injection into the brain to induce neurotoxicity. Once inside the brain, 6-OHDA is taken up by DA/norepinephrine membrane transporters (DMT/NMT), leading to intracellular accumulation and the generation of hydrogen peroxide and ROS. This, in turn, exacerbates oxidative stress, inhibits mitochondrial complex activity, and induces mitochondrial dysfunction, ultimately culminating in neuronal degeneration within specific brain regions. Targeted injection of 6-OHDA into the SN pars compacta, striatum, or medial forebrain bundle disrupts the dopaminergic nigrostriatal system, producing classic Parkinsonian motor deficits in mice. This process effectively recapitulates the progression of pathological and clinical manifestations observed in PD, establishing 6-OHDA as a widely used neurotoxin for inducing PD animal models (Simola et al., 2007).
3.1.2 1-Methyl-4-phenyl-1,2,3,6-tetrahydropyridine (MPTP)
MPTP, due to its high lipophilicity, readily crosses the BBB to reach the CNS. Within astrocytes, MPTP is metabolized to 1-methyl-4-phenylpyridinium (MPP+), which then enters dopaminergic neurons via the DA transporter. Inside neurons, MPP+ binds to neuromelanin and is transported and stored in synaptic vesicles via the vesicular monoamine transporter type 2 (VMAT-2). Prolonged exposure to MPP+ activates microglia, triggering the release of proinflammatory factors that contribute to neurotoxic responses. Furthermore, MPP+ inhibits the activity of mitochondrial complex I and suppresses the expression of anti-apoptotic proteins, ultimately impairing ATP synthesis within the electron transport chain and disrupting mitochondrial function. This disruption leads to excessive ROS production, which further promotes the generation and aggregation of α-Syn into toxic oligomers. The cumulative effects of these mechanisms damage dopaminergic neurons in the nigrostriatal pathway, effectively simulating the pathophysiological processes of PD (Mustapha and Taib, 2021).
3.2 Pesticide-induced models
Epidemiological studies have established a strong correlation between pesticide exposure and an increased risk of developing PD. Currently, rotenone and paraquat, two widely used pesticides, are frequently employed to generate PD models. Rotenone, a potent mitochondrial complex I inhibitor, shares a mechanism of action like that of MPTP. Due to its high lipophilicity, rotenone readily crosses the BBB, inhibiting mitochondrial complex I function and inducing widespread mitochondrial dysfunction. Additionally, rotenone activates microglia, exacerbating oxidative stress and promoting the accumulation of α-Syn. Research suggests that chronic, low-dose administration of rotenone may more accurately recapitulate the pathophysiology of PD (Innos and Hickey, 2021; Ibarra-Gutiérrez et al., 2023). In contrast, paraquat, an herbicide structurally similar to MPTP, does not inhibit mitochondrial complexes. Instead, paraquat exerts its toxic effects by disrupting the redox cycling of mitochondria, effectively diminishing cellular antioxidant capacity. The toxicity of paraquat to dopaminergic neurons is thought to be mediated by the DMT. Specifically, when paraquat (PQ2+) is reduced to the monovalent cation (PQ+), it can act as a substrate for DMT, leading to its accumulation within dopaminergic neurons and subsequent oxidative stress and cytotoxicity (Rappold et al., 2011).
3.3 Genetic models
Genetic mutations are strongly implicated in familial or genetic forms of PD. Genes such as SNCA, PRKN, LRRK2, and GBA are recognized risk factors for PD. Consequently, gene knockout represents a viable approach to model PD in animals. In previous studies, most models lacking specific PD-related genes have exhibited pathophysiological hallmarks of PD, including dopaminergic neurons degeneration, mitochondrial dysfunction, oxidative stress, neuroinflammation, and characteristic motor deficits such as bradykinesia. Furthermore, transgenic animal models have been developed to mimic PD pathology in vivo by expressing mutant SNCA genes, including those encoding wild-type, A53T, A30P and E46K α-Syn proteins (Lim and Ng, 2009).
4 Natural products (NPs)
NPs are compounds derived from natural sources such as plants, animals, and microorganisms. NPs have been extensively utilized in drug development and disease treatment. With advancements in modern chemistry and pharmacological techniques, the isolation and characterization of NPs have become increasingly sophisticated, providing new avenues for drug discovery. In recent years, studies have demonstrated that many NPs possess significant biological activity and exhibit promising therapeutic effects in a wide range of diseases, including cardiovascular diseases, gastrointestinal disorders, respiratory diseases, and infectious diseases (Joo, 2014; Peter et al., 2021). Given that PD remains an incurable neurodegenerative disorder, and considering the limitations and adverse effects associated with existing pharmacological treatments, researchers have turned their attention to exploring NPs as potentially safer and more effective therapeutic or adjunct agents for PD.
4.1 Polyphenolic compounds
Polyphenolic compounds, a class of NPs ubiquitous in plants, are primarily obtained from dietary sources such as fruits, vegetables, tea, red wine, and certain nuts. Their characteristic chemical structure, typically comprising multiple hydroxyl and aromatic rings, confers potent antioxidant properties. Polyphenols can be broadly categorized into flavonoids, phenolic acids, anthocyanins, tannins, and other subgroups. These compounds exhibit a wide array of biological activities, including antioxidant, anti-inflammatory, anti-tumor, antibacterial, and cardiovascular protective effects, highlighting their potential therapeutic value in preventing and treating various chronic diseases. Research suggests that a reasonable intake of polyphenolic compounds may contribute to improved health outcomes and a reduced risk of disease development (de Araújo et al., 2021).
4.1.1 Curcumin
Curcumin (Figure 4.1), a natural polyphenolic compound found in turmeric, possesses a distinctive chemical structure of 1,6-heptadiene-3,5-dione-1,7-bis(4-hydroxy-3-methoxyphenyl)-(1E, 6E) (Goel et al., 2008). It exhibits high solubility in organic solvents but poor solubility in aqueous solutions. Additionally, curcumin remains stable in acidic environments but readily decomposes under neutral and alkaline conditions (Wang et al., 1997). Curcumin has long been recognized for its diverse biological activities, including antioxidant (Menon and Sudheer, 2007), anti-inflammatory (Menon and Sudheer, 2007), anticancer (Cheng et al., 2001), antimicrobial (Shao et al., 2024), antithrombotic (Keihanian et al., 2018), blood sugar-lowering (Nabavi et al., 2015), and cardioprotective effects (Zhang et al., 2020).
Curcumin’s remarkable ability to penetrate the BBB has led to its widespread investigation as a potential therapeutic agent for neurodegenerative diseases, including PD. Sharma et al. demonstrated in a PD animal model that curcumin treatment significantly inhibited astrocyte activation, downregulated NF-κB transcription factor expression, and suppressed the production of inflammatory factors, ultimately modulating α-Syn aggregation. Moreover, curcumin supplementation was found to inhibit the α-Syn formation and aggregation by suppressing α-Syn gene expression (Sharma and Nehru, 2018).
Several studies suggest that curcumin exerts its inhibitory effects by directly interacting with α-Syn during its phase separation. For instance, Xu et al. reported that while curcumin does not interfere with the initial formation and conformation of α-Syn condensates, it significantly inhibits their transformation into amyloid-like proteins by reducing α-Syn mobility within these condensates (Xu et al., 2022). Similarly, Ahmad et al. discovered that curcumin possesses a unique remodeling capacity. Specifically, its interaction with α-Syn monomers disrupts long-range interactions within the protein chain, leading to an increased refolding rate, prevention of α-Syn aggregation, and temperature-dependent reaction kinetics (Ahmad and Lapidus, 2012).
The dose-dependent inhibitory effect of curcumin on α-Syn aggregation was further corroborated by Pandey et al., who observed that curcumin, particularly at concentrations of 10−6–10−7 M, significantly increased the soluble fractions of α-Syn monomers, dimers, and oligomers. This increase in solubility reduces the stability of these aggregates, rendering them more susceptible to degradation (Pandey et al., 2008). These findings align with those of Ono et al., who also emphasized the importance of curcumin dosing in inhibiting α-Syn aggregation and fibril growth (Ono and Yamada, 2006). Furthermore, curcumin has been shown to bind to preformed α-Syn fibrils and aggregates, altering their hydrophobic surface structure and reducing α-Syn toxicity. Intriguingly, curcumin appears to selectively bind to the ordered structure of the protein, with the degree of binding correlating with the extent of α-Syn oligomerization (Singh D. et al., 2012).
Research on curcumin as an adjunct therapy for cancer has been extensive, consistently demonstrating its favorable safety profile. Curcumin is generally well-tolerated, even at single oral doses as high as 12 g/d. However, its clinical application and development have been hampered by its poor solubility, inherent instability, and suboptimal pharmacokinetic profile. Specifically, inadequate intestinal absorption, rapid metabolism, and rapid systematic elimination result in low plasma and tissue concentrations, limiting its therapeutic efficacy. Interestingly, co-administration with piperine, a natural alkaloid found in black pepper, has been shown to increase curcumin bioavailability by an impressive 2,000%, highlighting the potential of piperine as a bioenhancer for curcumin (Sharma et al., 2007; Anand et al., 2007).
To address the inherent limitations of curcumin, researchers have focused on synthesizing curcumin analogs with enhanced biological activity. For instance, Jha et al. synthesized a series of curcumin analogs by substituting the hydroxyl group on the phenyl ring with various functional groups. These modifications differentially reduced the hydrophobic surface exposure of α-Syn oligomers, effectively mitigating their cytotoxicity (Jha et al., 2016). Notably, 4-arylidene curcumin derivatives (Figure 4.2) exhibited superior anti-aggregation properties, effectively targeting both α-Syn fibrils and oligomers (Liu W. et al., 2023). Similarly, two other stable curcumin analogs, curcumin pyrazole (Figure 4.3) and curcumin isoxazole (Figure 4.4), have demonstrated promising anti-amyloidogenic activity, effectively preventing fibrillation, disrupting preformed fibrils, and inhibiting the formation of toxic A11 protein conformations (Ahsan et al., 2015). Furthermore, Gadad et al. synthesized curcumin-glucoside (Figures 4.5, 4.6), which also exhibited robust inhibitory effects on α-Syn aggregation and fibril formation under in vitro conditions (Gadad et al., 2012). These findings collectively suggest that curcumin and its related polyphenolic compounds hold significant promise as therapeutic candidates for PD and other related neurodegenerative disorders (Table 1).
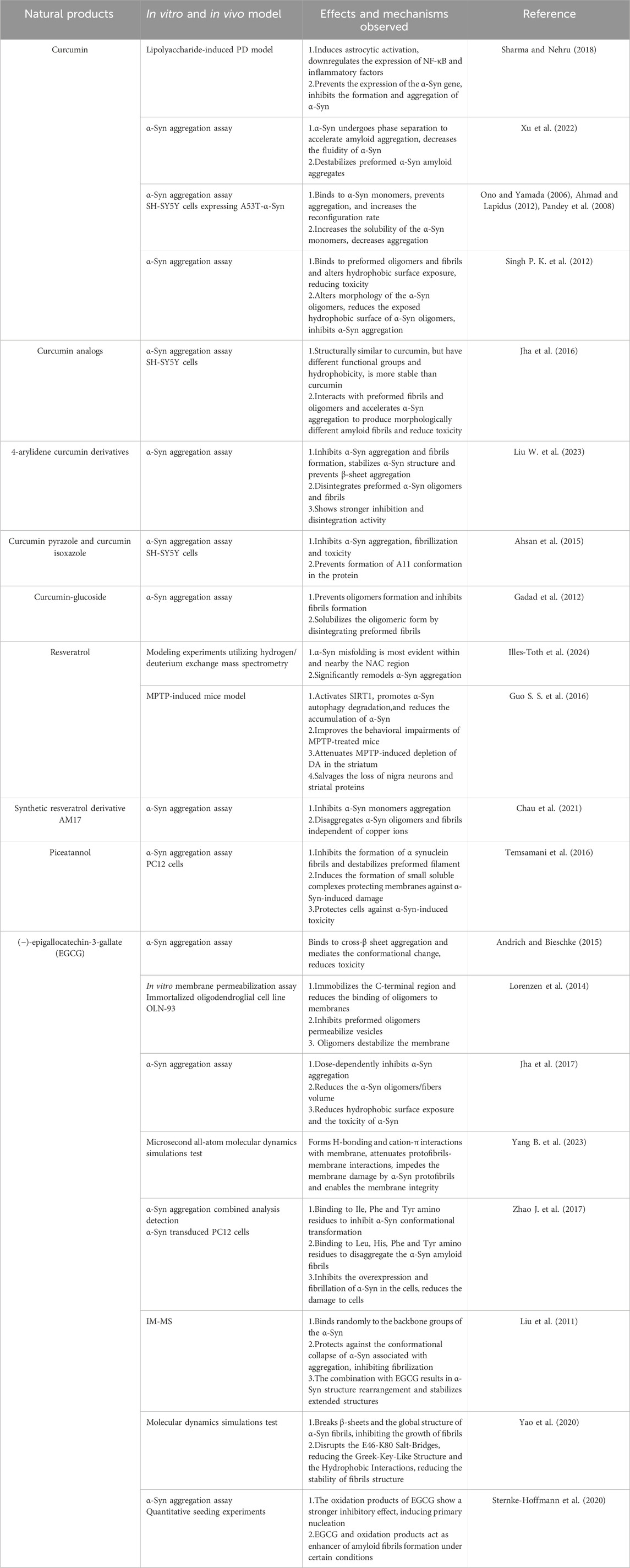
Table 1. Positive effects of polyphenolic compounds targeting α-Syn in vivo and in vitro models of PD.
4.1.2 Resveratrol
Resveratrol (Figure 4.7), a low molecular-weight polyphenolic compound, is abundant in various plants and trees, including pine, eucalyptus, grapes, blueberries, and cranberries. It exhibits a wide range of protective activities, including antioxidant, anti-inflammatory, free radical scavenging, and neuroprotective effects, establishing its potential as a dietary supplement for neurodegenerative diseases (Bastianetto et al., 2015). Kinetic modeling experiments employing hydrogen/deuterium exchange mass spectrometry have revealed that resveratrol significantly remodels α-Syn aggregation (Illes-Toth et al., 2024).
In PD models induced by 6-OHDA, MPTP, and rotenone, resveratrol effectively ameliorates motor deficits in mice (Zhao X. et al., 2017; Jin et al., 2008; Lu et al., 2008; Liu D. et al., 2019). It reduces α-Syn expression and toxicity while enhancing cell survival, likely through the activation of sirtuin 1, which promotes α-Syn degradation via autophagy (Guo S. S. et al., 2016). Resveratrol analogs have also demonstrated comparable neuroprotective effects. For instance, the resveratrol derivative AM17 (Figure 4.8) and piceatannol (Figure 4.9) both inhibit α-Syn monomer aggregation and disassemble preformed α-Syn oligomers and fibrils, independent of copper ions (Temsamani et al., 2016; Chau et al., 2021).
Although resveratrol readily crosses the BBB (Marier et al., 2002), its rapid metabolism, low bioavailability, and inherent chemical instability limit its clinical utility, even at high concentrations (Calamini et al., 2010). While resveratrol exhibits an oral absorption rate of approximately 75%, it undergoes rapid first-pass metabolism and elimination, resulting in low systemic exposure. Furthermore, circadian rhythms may influence resveratrol bioavailability. Studies suggest that morning administration may enhance its absorption. The recommended oral dosage range for resveratrol is 100–1,000 mg. Doses exceeding 2 g/d may lead to adverse effects such as diarrhea, nausea, vomiting, or headaches (Vesely et al., 2021).
To overcome these limitations, structural modifications aimed at improving bioavailability, metabolic stability, and biological activity without compromising its protective effects are being actively pursued. Current modification strategies include hydroxylation, amination/amidation/imination, methoxylation, prenylation, and glycosylation. Notably, resveratrol derivatives and analogs, such as oxyresveratrol, piceatannol, and imine resveratrol derivatives, exhibit more favorable pharmacokinetic profiles compared to the parent compound, demonstrating faster absorption, higher bioavailability, and greater metabolic stability (Li S. Y. et al., 2014; Setoguchi et al., 2014; Jung et al., 2009). Moreover, novel drug delivery systems, such as nanoparticle-loaded resveratrol and vitamin E-loaded resveratrol nanoemulsions, have shown reduce oxidative stress, enhance intracranial drug concentrations, and diminish degenerative lesions, demonstrating promising therapeutic effects against PD (Pangeni et al., 2014) (Table 1).
4.1.3 Green tea catechins
The major polyphenolic compounds found in green tea are collectively known as catechins (flavan-3-ols). Catechins have demonstrated a wide array of beneficial health effects, including anti-inflammatory, antioxidant, antibacterial, anticancer, infection-preventive, cognitive-enhancing, memory-improving, and neuroprotective properties. These compounds have been implicated in the treatment of chronic diseases such as cardiovascular diseases, diabetes, obesity, and neurodegenerative disorders. Green tea catechins primarily include (-)-epicatechin (EC) (Figure 4.10), (-)-epigallocatechin (EGC) (Figure 4.11), (-)-epicatechin-3-gallate (ECG) (Figure 4.12), and (-)-epigallocatechin-3-gallate (EGCG) (Figure 4.13). The antioxidant activity of these catechins varies depending on the number of hydroxyl groups and the structure of their substituent groups. EGCG is the most abundant catechin in green tea, followed by EGC (Musial et al., 2020).
Studies have shown that green tea catechins can mitigate α-Syn pathology in PD models. For instance, in MPTP-induced PD monkeys, administration of catechin-rich tea polyphenol extracts significantly reduced α-Syn aggregation in the striatum and hippocampus, attenuating α-Syn-induced dopaminergic neurons loss and motor dysfunction (Chen et al., 2015). Using parallel mass spectrometry to analyze green tea metabolites, Williams et al. found that, in addition to EGCG, both catechin and EC not only inhibited the formation of α-Syn fibrils but also destabilized preformed α-Syn fibrils (Williams et al., 2007).
To date, EGCG (Figure 4.13) is one of the most extensively studied and bioactive catechins extracted from green tea. In preformed α-Syn fibril models, EGCG disrupts the structural integrity of α-Syn fibrils by interfering with hydrogen bonds, aromatic stacking, and cation-π interactions, thereby inhibiting α-Syn fibrillation. In cellular models, EGCG directly binds to the β-sheet structure of α-Syn aggregates, inducing conformational changes that inhibit aggregation and promote the breakdown of aggregates into smaller, less toxic, and more disordered species (Andrich and Bieschke, 2015; Bieschke et al., 2010; Dominguez-Meijide et al., 2020). The destabilizing effect of EGCG on α-Syn fibrils is closely associated with the disruption of the E46-K80 salt bridge (Yao et al., 2020). Moreover, EGCG interaction with α-Syn reduces the affinity between α-Syn and lipid membranes, thereby mitigating hydrophobic surface exposure and cytotoxicity (Zhao J. et al., 2017; Lorenzen et al., 2014; Jha et al., 2017; Andrich and Bieschke, 2015; Yang Z. et al., 2023).
Furthermore, EGCG has been shown to interfere with copper (II) (Cu(II))-induced ROS generation, protecting cells from the toxic effects of α-Syn overexpression and fibrillation (Teng et al., 2019). Additionally, EGCG may influence α-Syn expression in vivo by modulating both the expression of the SNCA gene and the methylation status of CpG sites within its promoter region (Ramakrishna et al., 2016). In MPTP-treated mice, EGCG attenuates α-Syn accumulation and reduces neuronal death, potentially through mechanisms involving increased Bcl-2 protein expression, suppressed Bax protein expression, and upregulation of the PKC pathway (Mandel et al., 2004).
Ion mobility-mass spectrometry (IM-MS) confirmed that EGCG binds to α-Syn in a non-specific manner, inducing a more compact protein structure that resists conformational changes and reduces fibrillar aggregate formation (Liu et al., 2011). Interestingly, EGCG oxidation products exhibit even stronger inhibitory effects on α-Syn aggregation than the parent compound. Paradoxically, some studies have reported that both EGCG and its oxidation products can accelerate α-Syn fibril formation. This discrepancy may be attributed to variations in experimental conditions and warrants further investigation (Sternke-Hoffmann et al., 2020) (Table 1).
Despite its potent neuroprotective properties, the therapeutic application of EGCG is hindered by its inherent instability and poor bioavailability. The rapid metabolic degradation of EGCG often necessitates high doses to achieve therapeutic efficacy. However, such high doses may lead to toxicity, presenting a significant challenge for clinical transition. To overcome these limitations, researchers are actively exploring strategies to enhance EGCG bioavailability and reduce its toxicity, including chemical modification and nanoparticle-based delivery systems (Mehmood et al., 2022).
4.1.4 Flavonoid-like compounds
Flavonoids, the most abundant and diverse group of polyphenolic compounds, are found ubiquitously in plants and dietary sources. They share a common structural backbone consisting of a 2-phenyl-benzo-alpha-pyran or flavan nucleus, comprising two benzene rings (A and B) linked by a C heterocyclic pyran ring. Based on variations in the pyran ring structure, flavonoids are classified into six major subclasses: flavonols, flavanones, flavanols, flavones, anthocyanins, and isoflavones. Recognized for their wide-ranging biological activities, flavonoids are often consumed as dietary supplements, offering numerous health benefits. The recommended daily dosage for flavonoids typically ranges from 500 to 1,000 mg (Billowria et al., 2024).
4.1.4.1 Hesperetin and hesperidin
Hesperetin (HST) (Figure 5.1) is a naturally occurring bioflavonoid found abundantly in citrus fruits (Rutaceae), including lemons, oranges, limes, tangerines, and grapefruits. Both HST and its glycoside derivative, hesperidin (HSD) (Figure 5.2), are potent antioxidants that effectively protect cells from oxidative stress-induced damage. Their anti-oxidant, anti-inflammatory, and neuroprotective properties have been implicated in mitigating the progression of neurodegenerative diseases, including PD (Evans et al., 2022; Cho, 2006; Hajialuani et al., 2019). Moreover, HST and HSD have demonstrated efficacy in enhancing both non-spatial and spatial learning and memory. These beneficial effects are attributed to their ability to bolster antioxidant defenses and enhance cholinergic and brain-derived neurotrophic factor (BDNF) signaling. Furthermore, HST and HSD have been shown to reduce dopaminergic neurons injury, mitochondrial dysfunction, and apoptosis in PD mouse models (Antunes et al., 2021; Ishola et al., 2019). Importantly, HSD, when administered in conjunction with low-dose levodopa, significantly inhibits ROS accumulation and oxidative stress-induced damage while ameliorating mitochondrial dysfunction and motor deficits (Kesh et al., 2021). This finding suggests that combining HSD with conventional PD medications may synergistically enhance therapeutic outcomes.
In cellular experiments, Wang et al. demonstrated that HST effectively inhibits α-Syn fibril formation by interfering with both the initial nucleation and elongation phases. Moreover, HST interacts with α-Syn monomers and disassembles preformed α-Syn aggregates, leading to the formation of shorter, thinner, and less structured oligomers. This structural remodeling reduces the cytotoxicity and neurotoxicity associated with α-Syn aggregates.
The protective effects of HST have been observed in in vivo models as well. In the Transgenic Caenorhabditis elegans (C. elegans) model, HST treatment significantly reduced α-Syn aggregation in the NL5901 strain, extending lifespan and improving overall health (Wang Q. et al., 2023). In Drosophila models, HST demonstrated beneficial effects on reproductive capacity and locomotor activity, suggesting its potential in ameliorating PD-related motor dysfunction (Ishola et al., 2021). HSD also exerts protective effects against α-Syn pathology. It downregulates the expression of key kinases involved in α-Syn production, including LRRK2, GSK3β, caspase-3, caspase-9, and POLG, thereby reducing α-Syn levels and cytotoxicity (Kesh et al., 2021). Notably, the effects of HST are concentration-dependent, with a cell viability rate of up to 98.4% observed at a concentration of 100 μM HST, indicating a favorable safety and tolerability profile (Wang W. et al., 2023) (Table 2).
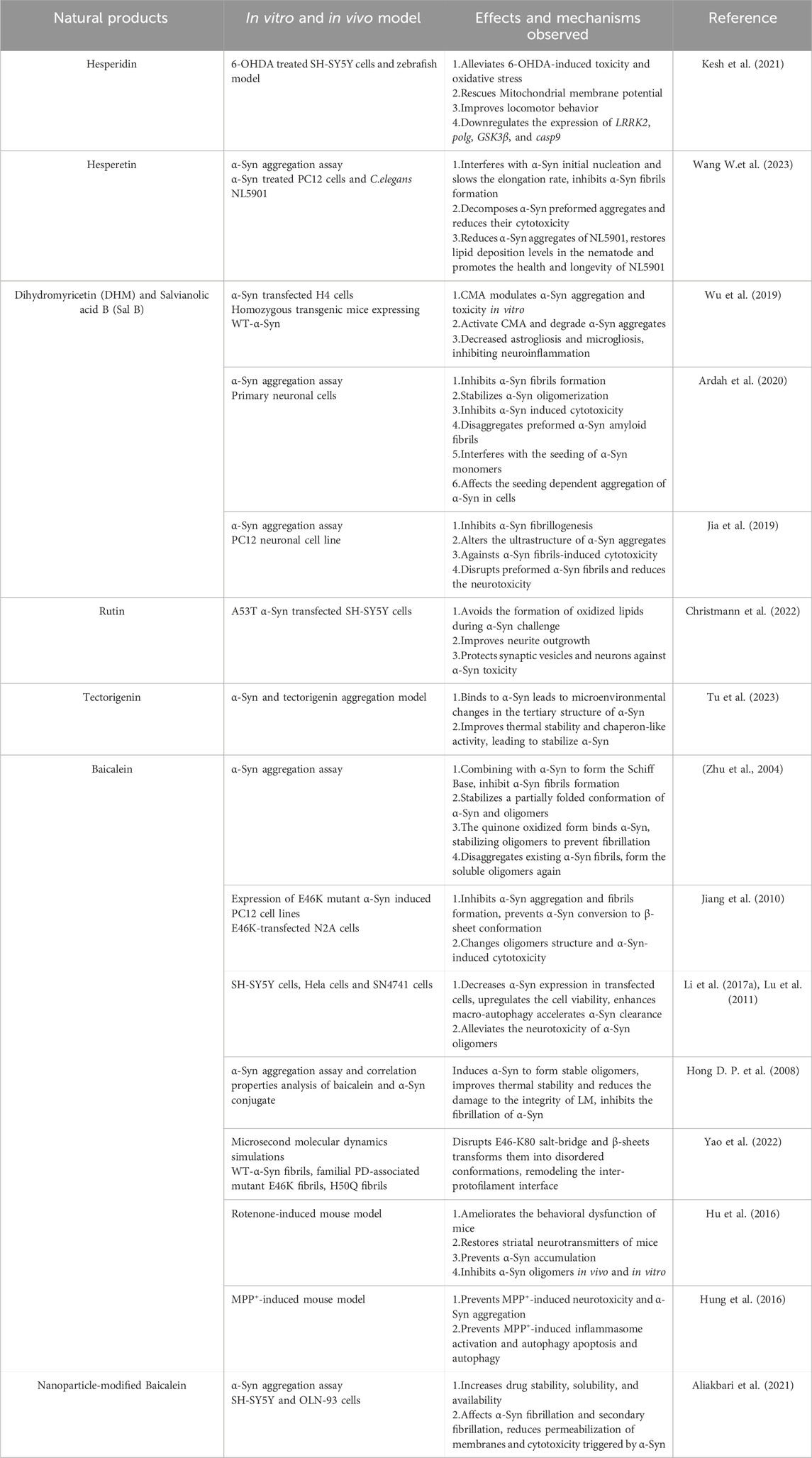
Table 2. Positive effects of flavonoid-like compounds targeting α-Syn in vivo and in vitro models of PD.
Studies investigating the pharmacokinetics of HST and HSD have reported no significant adverse effects following oral administration. HST is rapidly absorbed into the bloodstream, appearing in plasma within 20 min of ingestion and reaching peak concentrations at 4 h. The serum half-life of HST ranges from 3.7 to 7 h, while its elimination half-life in urine is approximately 25 h (Kanaze et al., 2007; Yáñez et al., 2008). Collectively, these findings suggest that HST, alone or in combination with HSD, holds promise as a dietary supplement for modulating α-Syn fibrillation and aggregation, potentially preventing or delaying the onset and progression of PD.
4.1.4.2 Dihydromyricetin
Dihydromyricetin (DHM) (Figure 5.3) is a bioactive flavonoid found predominantly in the stems and leaves of rattan grapes (Ampelopsis grossedentata), where its concentration can reach 30%–40% (Sun et al., 2021). DHM is also present in other medicinal plants and plant-based foods, including grapes, bayberries, Ginkgo biloba, Hovenia dulcis, and Cedrus deodara (Liu M. et al., 2020; Liu Q. et al., 2019). DHM possesses a wide range of pharmacological properties, including antioxidant, free radical scavenging, anti-inflammatory, antitumor, antimicrobial, cell death-modulating, and lipid- and glucose-regulating activities (Li H. et al., 2017). Importantly, acute and long-term toxicity studies, as well as genotoxicity tests, have confirmed the non-toxic nature and long-term safety of DHM (Zhang et al., 2021).
Previous studies have established that chaperone-mediated autophagy (CMA) plays a crucial role in the degradation of α-Syn (Xilouri et al., 2013b; Cuervo et al., 2004). CMA is a highly regulated cellular process that selectively targets proteins for lysosomal degradation (Kaushik and Cuervo, 2008). DHM has been shown to induce autophagy through the regulation of the AMPK/PCG pathway and other signaling pathways (Shi et al., 2015a; Shi et al., 2015b). Building upon these findings, Wu et al. demonstrated that treatment with DHM and salvianolic acid B (Sal B) (Figure 5.4) effectively inhibited the accumulation and aggregation of α-Syn fibrils both in vitro and in vivo. DHM and Sal B treatment led to a significant increase in the expression of LAMP-2A, a key marker of CMA, confirming the involvement of DHM in this pathway. The authors further showed that DHM and Sal B reduce α-Syn levels by enhancing CMA activation, thereby mitigating cytotoxicity and inhibiting inflammatory responses (Wu et al., 2019). Ardah et al. extended these findings, reporting that both compounds stabilized α-Syn oligomers. Interestingly, they observed distinct binding preferences for each compound. DHM preferentially bound to α-Syn oligomers, while Sal B exhibited higher affinity for α-Syn monomers. Remarkably, DHM also demonstrated the ability to degrade preformed α-Syn fibrils (Ardah et al., 2020). These findings are consistent with those reported by other research groups (Jia et al., 2019) (Table 2).
4.1.4.3 Rutin
Rutin (Figure 5.5) is a widely distributed polyphenolic flavonoid found in various plants and fruits, including buckwheat, apricots, oranges, cherries, and grapes (Huang et al., 2012; Kreft et al., 1999; Ganeshpurkar and Saluja, 2017). Structurally, rutin is a glycoside composed on the flavonol aglycone quercetin and the disaccharide rutinose. Extensive research has highlighted the numerous health-promoting properties of rutin, including antioxidant, neuroprotective, vasoprotective, and cytoprotective effects (Kim et al., 2009; Javed et al., 2012; Enogieru et al., 2018; Kalgaonkar et al., 2010; Kamalakkannan and Prince, 2006; Khan et al., 2009).
The gut-brain axis hypothesis highlights the critical importance of early intestinal intervention in the initial stages of neurodegenerative diseases like PD. Christmann et al. investigated the efficacy of early intervention with rutin supplementation in both ENS and CNS. Their findings demonstrated that rutin effectively mitigated the deleterious effects of α-Syn on cells, promoting neuronal growth and protecting synaptic vesicles and neurons from α-Syn toxicity. These protective effects are attributed to rutin’s ability to reduce lipid peroxidation and scavenge ROS.
Despite its promising preclinical profile, the clinical application of rutin is limited by its poor solubility and absorption, resulting in low oral bioavailability (Liu Y. et al., 2020). Interestingly, Christmann et al. observed that rutin nanocrystals exhibited enhanced protective capabilities compared to conventional rutin particles, suggesting that nanoformulations may improve its bioavailability and therapeutic efficacy (Christmann et al., 2022) (Table 2). These findings underscore the potential of antioxidant dietary supplements like rutin, particularly when administered via the intestinal route, for early prevention and treatment of PD. Further research is warranted to optimize rutin delivery and evaluate its clinical efficacy in PD patients.
4.1.4.4 Tectorigenin
Tectorigenin (Figure 5.6) is a naturally occurring methoxylated flavone found predominantly in the rhizomes of Iridaceous plants, such as Iris spuria and Iris tectorum. It is also present in other genera, including Pueraria, Morus alba, and Codonopsis pilosula (Rong et al., 2023). Tectorigenin exhibits a wide range of biological activities, including antioxidant, anti-inflammatory, antibacterial, anticancer, hypoglycemic, and hepatoprotective effects (Hong D. P. et al., 2008; Thelen et al., 2005; Jiang et al., 2012; Bae et al., 1999; Lee et al., 2003; Pan et al., 2008; Park et al., 2002). In an MPP+-induced cellular model of PD, tectorigenin exhibited neuroprotective effects, potentially by mitigating oxidative stress. Similar to other polyphenolic compounds, tectorigenin readily crosses the BBB, particularly when conjugated to a delivery vehicle. This characteristic makes it a promising candidate for PD therapeutics (Youdim et al., 2004; Gong et al., 2017).
Recent studies have revealed that tectorigenin binds to α-Syn through a combination of hydrogen bonds and van der Waals forces. This interaction induces conformational changes in the tertiary structure of α-Syn, leading to the formation of a stable tectorigenin-α-Syn complex (Tu et al., 2023). Notably, complex formation significantly enhances the thermal stability and chaperone activity of α-Syn. Furthermore, tectorigenin exhibits a higher binding affinity for α-Syn fibrils than for monomers (Table 2).
While generally safe within therapeutic dosage ranges, tectorigenin’s toxicity appears to be dose-dependent. Exceeding a specific concentration or prolonging treatment duration may lead to adverse effects. However, like many flavonoids, its poor water solubility limits its bioavailability. Despite this limitation, tectorigenin’s relatively long half-life suggests a prolonged duration of action in vivo. This prolonged activity may offer advantages over other NPs (Rong et al., 2023). However, further research is necessary to confirm these findings. Current knowledge regarding the relationship between tectorigenin and α-Syn aggregation mechanisms in PD remains limited, lacking robust experimental and clinical evidence.
4.1.4.5 Baicalein
Baicalein (Figure 5.7) is a flavonoid compound derived from the rhizomes of Scutellaria baicalensis. In addition to the shared biological activities of flavonoids, baicalein has been identified as a potent inhibitor of α-Syn aggregation. Interestingly, its oxidized form demonstrates inhibitory effects even at low concentrations, likely due to its quinone structure (Figure 5.8), which may facilitate interactions with α-Syn and promote the formation of structurally stable, soluble aggregates (Zhu et al., 2004). Baicalein also shows protective effects against α-Syn aggregation-induced toxicity, including proteinase activity inhibition, mitochondrial dysfunction, and cytotoxicity, particularly in the context of the E46K mutation (Jiang et al., 2010).
Baicalein has shown multiple protective effects against α-Syn aggregation. It upregulates autophagy, reduces α-Syn expression, and prevents the sustained accumulation of α-Syn aggregates. Moreover, baicalin inhibits protofibril formation, degrades pre-formed protofibrils, and promotes the structural transformation of α-Syn into larger, soluble aggregates (Li et al., 2017a; Lu et al., 2011). Investigations into the structural characteristics of baicalein-stabilized oligomers have revealed that these oligomers adopt spherical structures with widths of only 8–22 nm. These spherical oligomers exhibit favorable thermodynamic and structural stability, preventing further conversion into fibrillar structures and mitigating their disruptive effects on LM (Hong J. et al., 2008).
Interestingly, baicalein differentially affects the structural integrity and stability of various α-Syn fibrils types. In E46K and H500 mutant fibrils, baicalein binds to both the C-terminal and N-terminal regions. In wild-type fibrils, it exhibits enhanced binding to the NAC region and disrupts the E46-K80 salt bridge. Furthermore, in E46K protofibrils, baicalein disrupts the E61-K80 salt bridge (Yao et al., 2022).
In vivo studies have provided further support for the neuroprotective effects of baicalein. In a rotenone-induced mouse model of PD, intraperitoneal (i.p.) administration of baicalein for 7–12 weeks significantly reduced α-Syn aggregation in multiple brain regions. This reduction in α-Syn aggregation was accompanied by the protection of dopaminergic neurons and improvement in behavioral deficits. However, baicalein did not appear to affect α-Syn secretion, as evidenced by the lack of change in α-Syn mRNA expression (Hu et al., 2016). Furthermore, in an MPP+-induced PD mouse model, baicalein exerted anti-inflammatory effects, likely mediated through the inhibition of α-Syn aggregation (Hung et al., 2016) (Table 2).
Despite its promising preclinical profile, baicalein suffers from poor water solubility and rapid metabolism, resulting in low bioavailability (13.1%–23.0%). Encouragingly, multiple human trials have demonstrated the safety and tolerability of baicalein within a dosage range of 100–2,800 mg (Pang et al., 2016; Li M. et al., 2014; Li H. et al., 2021). Nanoparticle-based delivery systems have shown promise in overcoming baicalein’s bioavailability limitations. These nanoformulations enhance baicalein’s solubility and stability, leading to improved BBB permeability and enhanced neuroprotection (Aliakbari et al., 2021). Taken together, in vivo and in vitro studies suggest that baicalein can inhibit various stages of neurotoxic α-Syn production, highlighting its potential as a neuroprotective agent for PD treatment.
4.2 Naphthoquinones
Naphthoquinone, a ubiquitous quinonoid organic compound, is characterized by an unsaturated six-carbon ring structure containing two carbonyl groups. It is primarily found in the natural metabolites of plants, animals, fungi, and bacteria. Naphthoquinone exhibits a wide range of biological activities, including antioxidant, anti-inflammatory, antimalarial, antitumor, antibacterial, and neuroprotective effects. Of particular interest, 1,4-naphthoquinone (1,4-NQ) (Figure 6.6) plays a crucial role in maintaining neuronal cell viability, effectively protecting cells against oxidative stress induced by neurotoxins (Santos et al., 2023; Aminin and Polonik, 2020).
4.2.1 Shikonin
Shikonin (SHK) (Figure 6.1) is a bioactive naphthoquinone compound extracted from the roots of Lithospermum erythrorhizon (Andújar et al., 2013). It is well-known for its free radical scavenging, antioxidant, and anti-inflammatory properties (Chen X. et al., 2001). Srivastava et al. demonstrated that SHK dose-dependently inhibits α-Syn aggregation both in vivo and in vitro. Increasing concentrations of SHK prolonged the lag phase of α-Syn aggregation, the lag phase of α-Syn aggregation can be extended to 26.14 h in the presence of equimolar α-Syn and SHK. Mechanistically, SHK binds to the C-terminus of α-Syn, preserving its helical and disordered secondary structures while reducing β-sheet content. This interaction stabilizes α-Syn monomers, reduces the complexity of aggregated structures, and delays fibril elongation. In C. elegans model, SHK significantly reduced α-Syn aggregation, improved motor deficits, and attenuated dopaminergic neurons degeneration (Srivastava et al., 2023) (Table 3).
These neuroprotective effects highlight SHK’s potential as a therapeutic agent for synucleinopathies, including PD. However, SHK’s limited solubility and chemical stability hinder its biological activity. Pharmacokinetic studies have revealed a half-life of 630.7 ± 124.9 min, a maximum concentration of 83.6 ± 8.8 ng/mL, and a time to maximum concentration of 1.0 ± 0.0 min. Importantly, prolonged or rapid administration, particularly at high doses, can lead to hepatotoxicity and nephrotoxicity (Sun et al., 2022).
4.2.2 Vitamin K
Vitamin K, a fat-soluble vitamin found abundantly in green leafy vegetables, encompasses various forms, including Vitamin K1 (Figure 6.2), Vitamin K2 (Figure 6.3), Vitamin K3 (Figure 6.4), Vitamin K4 (Figure 6.5), and other forms (Mladěnka et al., 2021). While initially recognized for its essential role in blood clotting (Mishima et al., 2023), recent studies have highlighted the potential of vitamin K, particularly 1,4-NQ (Figure 6.6), in treating neurodegenerative diseases. These therapeutic benefits likely stem from vitamin K’s antioxidant, anti-inflammatory, and anti-demyelination properties (Santos et al., 2023; Emekli-Alturfan and Alturfan, 2023; Diachenko et al., 2024; Sandeep et al., 2023). Fernanda et al. demonstrated the inhibitory effects of vitamin K and 1,4-NQ on α-Syn aggregation. Both compounds effectively decelerated α-Syn fibrillation by interacting with the N-terminal repeat domain of α-Syn monomers. Treatment with vitamin K or 1,4-NQ resulted in the formation of smaller, fragmented fibrils and amorphous aggregates, which exhibited a reduced capacity to induce vesicle leakage (Table 3).
Interestingly, a case-control study found significantly lower serum vitamin K levels in PD patients compared to healthy controls. Moreover, serum vitamin K levels were higher in early-stage PD patients than in those with later-stage disease, suggesting that vitamin K deficiency may contribute to PD pathogenesis and that vitamin K supplementation may hold therapeutic and preventive potential (Liu L. et al., 2023; Yu et al., 2020). Given that inhibiting MAO activity is a key pharmacological target in PD treatment, and based on the structural features of 1,4-NQ, vitamin K, shows promise for developing MAO inhibitors and represents a promising avenue for developing inhibitors of α-Syn fibrillation and aggregation (da Silva et al., 2013).
4.3 Tanshinones
Danshen, a medicinal plant with a rich history of use in China, is renowned for its protective effects on cardiovascular and cerebrovascular function. Its safety has been well established through centuries of traditional use. Tanshinones, the primary bioactive constituents extracted from the dried roots of Danshen, include tanshinone I and tanshinone IIA (TAN I and IIA) (Figures 7.1, 7.2) (Li L. et al., 2021). Despite their therapeutic potential, the bioavailability of tanshinones is limited by their poor water solubility and dissolution rate. However, their lipophilic nature presents opportunities for enhancing their bioavailability through various formulation strategies. For example, compared to other extracts, tanshinone formulations have demonstrated improved maximum concentration and half-life values (Wang D. et al., 2020; Wang X. et al., 2020).
Both TAN I and IIA have demonstrated remarkable efficacy in inhibiting amyloid-β peptide aggregation and promoting the breakdown of existing amyloid fibrils (Ren et al., 2017; Ren et al., 2015; Wang et al., 2013). In vitro experiments have revealed that both compounds can prolong the lag phase of α-Syn aggregation and promote the degradation of preformed, mature α-Syn fibrils, likely by influencing β-sheet formation. Furthermore, treatment with TAN I and TAN IIA significantly extended the lifespan of NL5901, likely due to a reduction in α-Syn aggregation and fibril formation (Ji et al., 2016). (Table 4) These findings underscore the potential of tanshinones as therapeutic agents for neurodegenerative diseases, including PD.
4.4 Lingzhi extracts
Ganoderma lingzhi, commonly known as Lingzhi is a highly valued medicinal mushroom. Its triterpenoids are considered to be key active components contributing to its therapeutic effects (Boh et al., 2007). Numerous studies have demonstrated the efficacy of Ganoderma in preclinical models of PD, attributing its beneficial effects to several mechanisms, including selective protection of dopaminergic neurons in the SN, reduction of oxidative stress, preservation of mitochondrial function, mitigation of neuroinflammation, and modulation of neural immunity (Li et al., 2017b; Guo Y. J. et al., 2016; Ren et al., 2019; Zhang et al., 2011).
Methyl Ganoderate E (MGE) (Figure 7.3), a triterpenoid compound isolated from Lingzhi, has shown inhibitory effects on the aggregation of both α-Syn and amyloid-β proteins. In NL5901, treatment with MGE at a concentration of 10 μg/mL resulted in a 15% reduction in α-Syn aggregation (Okoro et al., 2024) (Table 4). These findings suggest that Lingzhi extracts, particularly its bioactive component MGE, holds promise as a potential therapeutic agent for PD. However, it is important to note that systematic studies on key pharmacological parameters of MGE, such as pharmacokinetics, bioavailability, safety, and efficacy are currently lacking, Further research is warranted to evaluate the clinical potential of MGE for treating PD.
4.5 Centella asiatica extracts
Centella asiatica (CA), commonly known as Gotu Kola, is a traditional herb with a long history of use in Chinese and Ayurvedic medicine (Singh P. K. et al., 2012). In vitro studies have confirmed the safety of CA, and it exhibits good BBB permeability without apparent toxic effects. However, the absolute bioavailability of CA is extremely low, only 1.86%, likely due to incomplete absorption and a rapid excretion rate (He et al., 2023). While traditionally used for treating skin conditions, emerging research has revealed the neuroprotective effects of CA. These neuroprotective benefits include enhancing memory, improving cognitive function, and stimulating neuronal growth (Gadahad et al., 2008; Veerendra Kumar and Gupta, 2002).
Extracts from CA have demonstrated promising effects against α-Syn aggregation. Studies have shown that CA extracts can stabilize α-Syn monomers, maintain their disordered structure, inhibit the formation of α-Syn aggregates and even promote the breakdown of preformed α-Syn fibrils, achieving a degradation rate of up to 70% (Berrocal et al., 2014). These protective effects are attributed to the synergistic actions of multiple compounds present in CA extracts, including caffeic acid, chlorogenic acid, gallic acid, and selenium. Furthermore, in a Drosophila model of PD, CA extracts significantly delayed motor dysfunction (Siddique et al., 2014). However, a recent study investigating the effects of Ayurvedic nootropics on α-Syn aggregation found that CA extracts, surprisingly, did not exhibit significant inhibitory activity (Anjaneyulu et al., 2020) (Table 4). This discrepancy might be attributed to variations in the composition of CA extracts obtained through different extraction methods. Further research is crucial to identify the specific CA extracts that confer the most potent therapeutic benefits in the context of PD.
4.6 Cinnamic acid derivatives (CADs)
CADs have garnered increasing attention due to their structural resemblance to curcumin and their reported antimicrobial, antifungal, anti-inflammatory, anticancer, and neuroprotective properties (Ruwizhi and Aderibigbe, 2020). Found abundantly in plants like Cinnamomum cassia and Panax ginseng, as well as in fruits, whole grains, and green coffee beans, CADs represent a promising class of bioactive compounds. Mara et al. demonstrated that natural CADs, 3,4-dimethoxycinnamic acid (Figure 7.4), 3-methoxy-4-acetamidoxycinnamic acid (Figure 7.5), and ferulic acid (Figure 7.6), can bind to both α-Syn oligomers and fibrils, effectively reducing β-sheet content within α-Syn aggregates. This interaction prevents the amyloidogenic transformation of α-Syn and ultimately inhibits its aggregation (Medvedeva et al., 2020). Importantly, both naturally extracted and chemically synthesized CADs exhibit these beneficial effects (Table 4). Notably, plasma levels of CADs increase significantly following the consumption of coffee containing CADs (Farrell et al., 2012; Nagy et al., 2011; Medvedeva et al., 2022). Their natural occurrence and presence in human blood, coupled with their potent anti-aggregation properties, highlight the potential of CADs as therapeutic candidates for PD.
4.7 Geum urbanum extracts
Geum urbanum (GU), a member of the Rosaceae family, is a medicinal herb with a long history of use in treating ailments such as gastric mucosal and oral inflammation, and it also exhibits cardioprotective properties. Ellagitannins, procyanidins, gallic acid, and vanillic acid constitute the primary components of GU extracts (Neshati et al., 2018; That et al., 2018). Recent studies have revealed that GU extracts not only stabilizes α-Syn fibrils but also promotes their disaggregation in a concentration-dependent manner (Lobbens et al., 2016). At a high concentration (0.25 mg/mL), the extracts inhibited fibril formation for up to 40 h, with the lag phase of fibrillation increasing proportionally with extracts concentration. Remarkably, the ability of α-Syn to form fibrils was attenuated to varying degrees under all tested conditions, with earlier addition of the extracts yielding more pronounced inhibitory effects. This observation suggests that GU extracts may reduce the fibrillation and aggregation propensity of one or more intermediate oligomeric species, thereby delaying α-Syn aggregation (Lobbens et al., 2016) (Table 4). However, due to the complex composition of the extracts, the precise structural characteristics and structure-function relationships of the active components remain to be elucidated. Moreover, the safety profiles of the derived oligomers warrant further investigation. Nevertheless, the strategy of targeting multiple intermediate species to mitigate their fibrillation potential represents a novel approach for developing disease-modifying therapies for PD.
4.8 (+)-Desdimethylpinoresinol
(+)-Desdimethylpinoresinol (03A10) (Figure 7.7), originally identified in the fruit of Vernicia fordii, has emerged as a potent inhibitor of α-Syn aggregation. In vitro studies have demonstrated that 03A10 does not significantly affect cell viability, supporting its safety profile as a potential therapeutic agent. Treatment with 03A10 resulted in a tenfold reduction in the size of α-Syn fibrils, accompanied by a decreased rate of aggregate formation and inhibition of β-sheet formation. Interestingly, 03A10 exhibited no appreciable affinity for α-Syn monomers, suggesting that its mechanism of action does not involve direct interaction with the monomeric form. Instead, 03A10 preferentially bound to α-Syn fibrils and displayed a high affinity for α-Syn aggregates. In a mouse model of PD, oral administration of 03A10 led to varying degrees of improvement in α-Syn aggregation and propagation, neuronal degeneration, behavioral deficits, olfactory dysfunction, and intestinal inflammation (Wang Q. et al., 2023) (Table 4).
4.9 Natural alkaloid compounds
Natural alkaloids, a class of nitrogen-containing organic compounds ubiquitous in plants, derive their name from their characteristically alkaline nature. These compounds exert a wide range of pharmacological effects, primarily by binding to specific receptors within biological systems. The remarkable diversity of their biological activities and pharmacological properties has garnered significant attention from researchers across various disciplines.
4.9.1 Quinoline and indole alkaloids
Natural alkaloids represent a promising source of bioactive compounds for modulating α-Syn aggregation. In a study investigating the relationship between alkaloids and α-Syn aggregation, six out of nine alkaloids tested (Figures 8.1–8.6), all derived from medicinal herbs, exhibited inhibitory effects on α-Syn seed fibril formation and seed-induced toxicity (Ghanem et al., 2021). Similarly, previous studies have shown that 2-(quinoline-8-carboxamido)benzoic acid (2-QBA) (Figure 8.8), a natural quinoline alkaloid isolated from Aspergillus sp. SCSIO06786, enhances proteasome activity, reduces the toxicity associated with α-Syn aggregates, and ameliorates motor dysfunction in a nematode model of PD (Lee et al., 2023). Moreover, Harmine (Figure 8.4), a β-carboline alkaloid found in Peganum harmala, and Lycorine (Figure 8.7), an isoquinoline alkaloid extracted from Amaryllidaceae plants, acts as natural enhancers of the UPS, can significantly enhance the phosphorylation of PKA, thereby activating the UPS to promote the degradation of pathogenic α-Syn in both in vitro and in vivo models of PD (Zhu et al., 2021; Cai et al., 2019). Furthermore, tetracyclic oxindole alkaloids, such as isorhynchophylline (Figure 8.9), corynoxine B, and corynoxine (an enantiomer of corynoxine B) (Figure 8.10), isolated from the Chinese herbal medicine Uncaria rhynchophylla, function as natural autophagy inducers. These alkaloids have been shown to mitigate α-Syn aggregation by inducing autophagy (Chen et al., 2014; Lu et al., 2012). Corynoxine B acts through the autophagy-regulating factor Beclin 1, whereas corynoxine exerts its effects through the AKT/mTOR pathway (Table 5). These findings provide compelling evidence for the potential of natural alkaloids as valuable leads for developing novel autophagy enhancers for the treatment of PD.
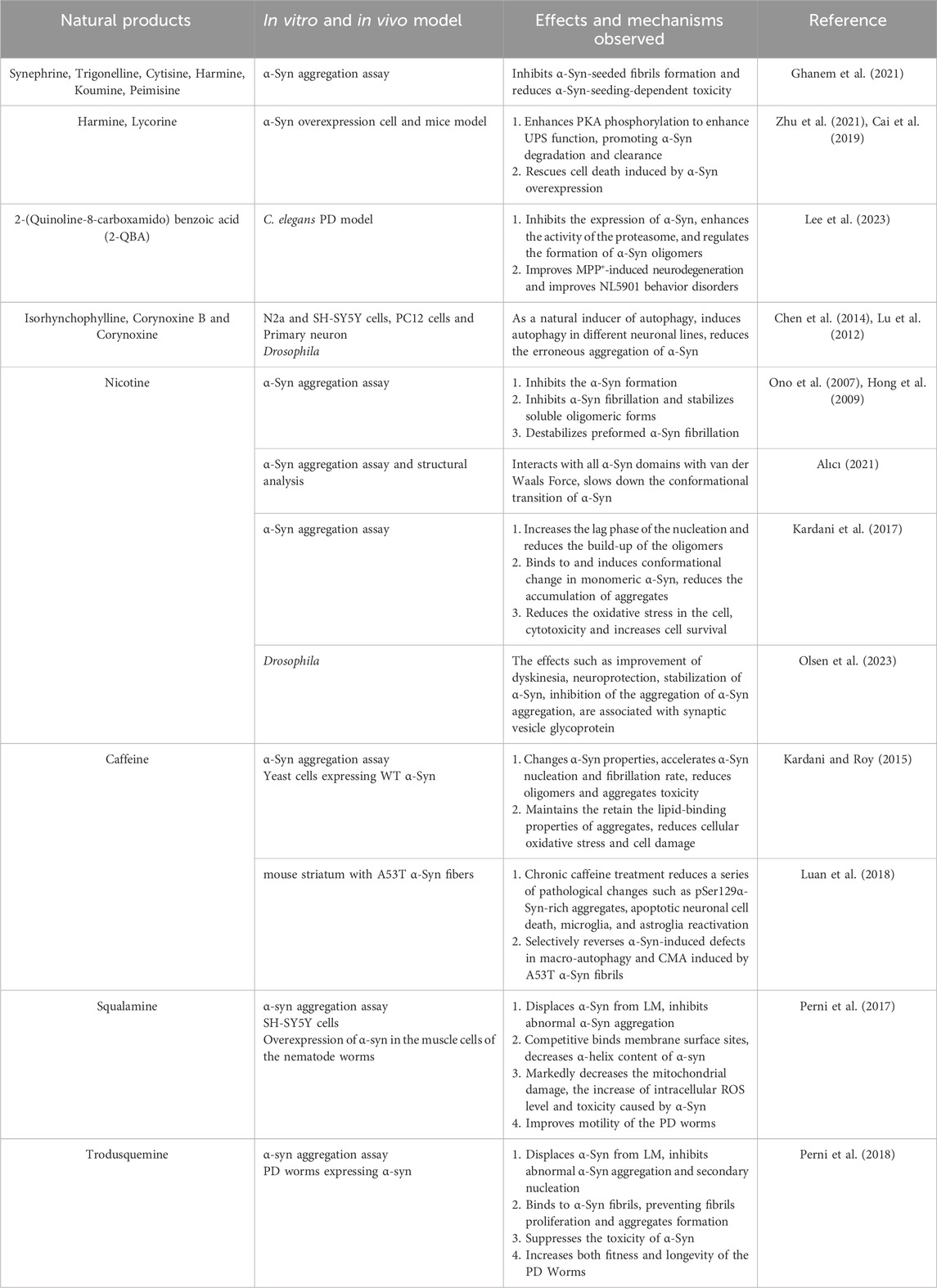
Table 5. Positive effects of natural alkaloid compounds targeting α-Syn in vivo and in vitro models of PD.
4.9.2 Nicotine
Epidemiological studies have consistently revealed a negative correlation between smoking and the incidence of PD (Quik, 2004). Nicotine (Figure 9.1), the primary alkaloid found in Solanaceae plants, has been shown to exert neuroprotective effects through the activation of nicotinic receptors located at dopaminergic terminals, which modulates DA release (Ma et al., 2017; Quik et al., 2012).
Studies suggest that nicotine can influence α-Syn dynamics and potentially modulate PD pathogenesis. Nicotine has been shown to stabilize soluble α-Syn oligomers, thereby inhibiting the formation of α-Syn fibrils and reducing the instability of preformed fibrils (Ono et al., 2007; Hong et al., 2009). It interacts extensively with α-Syn, engaging nearly all of its structural domains through van der Waals forces. This interaction helps maintain the helical structure of α-Syn and prevents detrimental conformational changes (Tavassoly et al., 2014; Alıcı, 2021). Moreover, nicotine can prolong the rate of aggregate nucleation, effectively delaying the conversion of α-Syn monomers into oligomers (Kardani et al., 2017). Interestingly, studies using synaptic vesicle glycoprotein 2 knockdown in Drosophila models suggest that nicotine may influence PD pathology by modulating vesicle release (Olsen et al., 2023) (Table 5). Collectively, these findings highlight the therapeutic potential of nicotine, and other agents targeting nicotinic acetylcholine receptors, as potential disease-modifying agents for PD.
Smoking represents the most rapid and efficient route for delivering nicotine to the bloodstream and brain, achieving stable concentrations within 30 min of intake. Due to the first-pass metabolism of the liver, the bioavailability of oral nicotine is about 20%–45%, while the bioavailability through inhalation is substantially higher (Hukkanen et al., 2005). While nicotine exhibits promising neuroprotective properties, its delivery via smoking is inextricably linked to the detrimental effects of tobacco smoke. The addictive and stimulant properties of nicotine, primarily attributed to its action on nicotine acetylcholine receptors, necessitate a cautious approach to its therapeutic application. This is particularly relevant for individuals with PD, especially elderly patients who may be more susceptible to nicotine addiction. Therefore, determining the effective dosage, optimal route of administration, and long-term safety of nicotine for PD treatment remains a significant challenge that warrants further investigation.
4.9.3 Caffeine
Caffeine (Figure 9.2), a xanthine alkaloid primarily derived from coffee, tea, and cocoa, has been linked to a reduced incidence of PD (Qi and Li, 2014). As a non-selective adenosine A2A receptor antagonist, caffeine may exert its neuroprotective effects on dopaminergic neurons by inhibiting A2A receptor signaling pathways (Ren and Chen, 2020; Qi and Li, 2014; Chen J. F. et al., 2001). Several studies have explored the intricate relationship between caffeine and α-Syn. Caffeine has been shown to induce conformational changes in α-Syn by binding to its N-terminal and C-terminal regions, thereby hindering its aggregation and preserving its lipid-binding properties (Tavassoly et al., 2014). Intriguingly, caffeine appears to modulate the aggregation kinetics of α-Syn in a complex manner. It has been reported to increase the formation of α-Syn oligomers and accelerate the conversion of oligomers into fibrils, ultimately leading to the formation of new, stable fibrils with reduced oligomer presence. This observation is significant because these newly formed fibrils exhibit lower toxicity compared to their oligomeric counterparts (Kardani and Roy, 2015). Furthermore, caffeine has demonstrated autophagy-enhancing properties. Chronic caffeine treatment has been shown to rescue macroautophagy defects induced by the A53T α-Syn mutation, accelerate the clearance and degradation of α-Syn, and alleviate α-Syn fibril-induced apoptosis in mice (Luan et al., 2018) (Table 5).
Moreover, caffeine’s dual solubility in water and lipids, along with its high BBB permeability, makes it a promising therapeutic agent for brain disorders. With an absorption rate of up to 99% in the gastrointestinal tract, a half-life of 2.5–4.5 h, and good oral bioavailability and tolerance, caffeine demonstrates favorable pharmacological properties (Fredholm et al., 1999). These characteristics, coupled with its ability to enhance autophagy and modulate α-Syn aggregation, suggest potential for targeted therapy in PD. However, despite its generally safe profile, excessive caffeine intake (exceeding grams) can lead to adverse effects, even fatal consequences, especially in individuals with certain metabolic disorders, liver diseases, or cardiovascular conditions (Musgrave et al., 2016). Therefore, careful evaluation of caffeine’s optimal dosage, adverse effects, and long-term toxicity remains crucial.
4.9.4 Squalamine
Squalamine (Figure 9.3), a water-soluble aminosterol isolated from the dogfish shark (Squalus acanthias), is well-known for its potent antibacterial properties (Moore et al., 1993). As a cationic amphipathic steroid, squalamine exhibits a high affinity for phospholipid membranes containing negatively charged headgroups (Selinsky et al., 1998; Brunel et al., 2005). Michele et al. demonstrated in vitro that squalamine’s positive charge mediates strong binding to anionic groups on LM, thereby inducing the dissociation of α-Syn from the LM. This competitive interaction effectively blocks α-Syn binding sites on the LM, inhibiting the formation of α-Syn aggregates. Furthermore, in a C. elegans model of α-Syn, squalamine treatment significantly reduced the cytotoxicity associated with α-Syn aggregates and ameliorated the behavioral and motor deficits (Perni et al., 2017). These findings suggest that squalamine inhibits α-Syn aggregation by targeting the kinetics of the aggregation process, a mechanism distinct from the previously reported inhibitory effects of NPs.
Squalamine has emerged as a promising therapeutic candidate for PD. Notably, ENT-01 (patent: CN106535902), the first squalamine-based drug, entered human clinical trials for PD in 2018 (NCT03781791). Phase II results indicated that ENT-01 exhibits a favorable safety profile, and 80% of patients experienced significant relief from constipation, a common NMS of PD, following ENT-01 treatment. Further evaluations of ENT-01’s effects on other neurological symptoms are planned for future studies (Hauser et al., 2019). Previous studies have provided evidence supporting the efficacy of squalamine in PD treatment, with preliminary findings suggesting that an oral dose of 500 mg/m2/day is relatively safe. However, further studies are needed to assess the potential for adverse effects associated with long-term or frequent squalamine administration (Hao et al., 2003).
4.9.5 Trodusquemine
Like squalamine, trodusquemine (Figure 9.4) is an aminosterol isolated from the liver of Squalus acanthias. Structurally similar to squalamine (Rao et al., 2000), trodusquemine also exerts inhibitory effects on α-Syn aggregation by displacing it from LM. However, in addition to inhibiting the initial nucleation event, trodusquemine can directly bind to preformed α-Syn fibrils, thereby preventing amplification of aggregation through secondary nucleation. This dual inhibition of nucleation processes may confer superior cytoprotective properties to trodusquemine compared to squalamine (Perni et al., 2018). Although experimental data regarding its pharmacokinetics, bioavailability, safety, and toxicological profile are currently limited, trodusquemine’s reported ability to penetrate the BBB and promote tissue regeneration makes it an attractive candidate for treating synucleinopathies, including PD.
4.10 Pyrroloquinoline quinone
Pyrroloquinoline quinone (PQQ) (Figure 7.8) is a potent antioxidant nutrient mainly synthesized by Gram-negative bacteria. It is naturally present in various foods, including natto, celery, tofu, kiwi, and oolong tea. Both animal and human studies have demonstrated the safety and tolerability of PQQ as an antioxidant and nutritional supplement. PQQ exhibits high oral absorption (62%) and does not accumulate significantly in the body. Moreover, no notable toxic effects have been reported (Akagawa et al., 2016).
PQQ has demonstrated inhibitory effects on amyloid formation, including that of α-Syn, β-amyloid protein, and mouse prion protein (Kim et al., 2010). Kobayashi et al. revealed through in vitro experiments that PQQ can inhibit α-Syn aggregation in a dose-dependent manner by forming a PQQ-α-Syn complex via a Schiff base structure (Kobayashi et al., 2006). Subsequently, the same group identified the α-Syn36-46 peptide as the key sequence responsible for the inhibitory effect of the PQQ–α-Syn complex. Interestingly, modifications of the α-Syn36-46 peptide with other compounds possessing quinone structures, such as curcumin and EGCG, also prevented α-Syn aggregation and fibril formation (Yoshida et al., 2013) (Table 4). These findings suggest that targeting the α-Syn36–46 peptide with specifically structured compounds, particularly those containing quinone moieties, represents a promising strategy for developing α-Syn aggregation inhibitors. While PQQ itself exhibits suboptimal BBB permeability, esterification of PQQ can effectively enhance its brain penetration. For example, PQQ-trimethylester, an esterified derivative of PQQ, has been shown to possess twice the BBB permeability of PQQ and exhibits a more potent inhibitory effect on α-Syn aggregation (Tsukakoshi et al., 2018).
5 Combined clinical trials and preclinical studies
Currently, clinical data on the use of NPs for the treatment or adjunctive treatment of PD remains limited. Clinical trials investigating the efficacy and safety of several NPs in PD, including curcumin (IRCT20191231045968N1, IRCT20101209005352N2, IRCT20171123037600N1), Ganoderma lucidum extracts (ChiCTR2100050538), nicotine (IRCT20210618051612N1, NCT01216904, NCT00873392, NCT01560754, NCT00957918, NCT02452125), and caffeine (NCT01190735, NCT00459420, NCT01738178), have been registered on clinical trials platforms (e.g., trialsearch. who.int, chictr. org.cn, clinicaltrials.gov). However, it is important to note that not all registered trials have been completed.
Clinical trials evaluating curcumin as a potential therapeutic agent for PD have yielded mixed results. Donadio et al. reported that curcumin supplementation (in combination with levodopa or DA agonists) in 21 PD patients led to significant improvements in dyskinesia and NMS, as well as a reduction in α-Syn deposition in the skin (Donadio et al., 2022). In contrast, a separate study involving 30 PD patients found that administration of curcumin nanomicelles for 9 months did not result in significant improvements in dyskinesia or NMS or quality of life (IRCT20171123037600N1) (Ghodsi et al., 2022).
Clinical trials exploring the therapeutic potential of nicotine in PD have produced conflicting results, likely due to variations in dosage and treatment duration. A study employing nicotine patches (maximum dose of 28 mg/24 h) for 52 weeks in individuals with early-stage PD (NCT01560754) did not find support for a beneficial effect of nicotine on disease progression (Oertel et al., 2023). Similarly, another using a comparable nicotine regimen (NCT00873392) reported similar findings (Villafane et al., 2018; Lemay et al., 2004).
In contrast, long-term maintenance therapy with higher doses of nicotine (more than 45–90 mg/day) has shown promise in improving motor function, reducing the need for dopaminergic medication, and potentially slowing the loss of DA transporters (Itti et al., 2009; Villafane et al., 2007). Furthermore, nicotine has consistently demonstrated a positive effect on semantic processing in PD patients, possibly due to its stimulatory effects on DA signaling and enhancement of inhibitory mechanisms (Holmes et al., 2011a; Holmes et al., 2011b). Interestingly, nicotine has also shown potential in managing hypotension, a potentially life-threatening complication of PD. A study investigating the effects of oral nicotine gum (NCT02452125) found that it effectively increased blood pressure in PD patients experiencing acute hypotension (DiFrancisco-Donoghue et al., 2019). These findings suggest that nicotine therapy may represent a novel treatment strategy for specific aspects of PD, including motor symptoms, cognitive impairment, and hypotension. A placebo-controlled, double-blind, randomized trial involving 24 PD patients found that a 100 mg dose of caffeine modestly improved attention (Sharma et al., 2022). Caffeine intake has also been associated with improvements in freezing of gait (Kitagawa et al., 2007). However, caffeine has not consistently demonstrated relief from NMS such as excessive daytime sleepiness (Postuma et al., 2012b). Similarly, two other clinical studies failed to show positive effects of caffeine therapy on motor symptoms or disease progression (Postuma et al., 2017; Simon et al., 2008).
A double-blind, randomized, cross-over trial involving 12 idiopathic PD patients revealed that caffeine accelerates levodopa uptake, significantly shortening the latency period for motor responses (Kyaw et al., 2013). This suggests that caffeine administration prior to levodopa intake may enhance the drug’s efficacy. Supporting this notion, a larger study (n = 222) found that caffeine use was associated with a reduced likelihood of developing motor complications in patients taking pramipexole and levodopa in PD (Wills et al., 2013). In summary, while caffeine exhibits certain positive effects in PD, including modest cognitive enhancement, potential benefits for freezing gait, and modulation of levodopa pharmacokinetics, its overall impact remains complex and likely varies among individuals.
Co-administration of certain natural products with conventional PD medications has shown potential in enhancing therapeutic outcomes. For instance, naringenin has been shown to significantly increase the maximum plasms concentration, brain concentration, and elimination half-life of rasagiline, a commonly prescribed DA agonist, when administered concurrently. Naringenin also reduces the clearance rate of rasagiline, likely due to its inhibitory effects on cytochrome P-450 1A2 (CYP1A2) metabolism (Pingili et al., 2016). Furthermore, naringenin itself exhibits MAO inhibitory activity, suggesting potential neuroprotective properties (Carradori et al., 2016). Similarly, theophylline has been shown to prolong the duration of levodopa’s effects and improve acute “off” periods in individuals with PD (Kulisevsky et al., 2002).
In a gait analysis experiment using a mouse model of PD, co-administration of baicalein with a low-dose of levodopa significantly improved gait disturbances, achieving therapeutic effects comparable to those observed with a high-dose of levodopa alone (Zheng et al., 2019). This suggests that baicalein may enhance the efficacy of levodopa, potentially allowing for lower, better-tolerated doses. Similarly, resveratrol has been shown to alleviate levodopa-induced motor dysfunction in rats without diminishing its anti-PD effects (Zheng et al., 2021).
Several NPs, including baicalein, curcumin, resveratrol, and EGCG, have also exhibited neuroprotective properties against the potential neurotoxicity associated with high-dose levodopa (Kang et al., 2013; Takeshima et al., 2011). These findings suggest that combination therapy with NPs may offer a multifaceted approach to PD treatment by enhancing the efficacy of conventional medications, reducing required doses, and providing neuroprotection. Further research is warranted to optimize these combination strategies and translate them into clinical practice.
6 Limitations of preclinical studies and future challenges
Preclinical studies have provided compelling evidence for the neuroprotective properties of natural products against α-Syn aggregation and deposition. However, several factors, particularly those related to pharmacokinetics, limit their clinical translation. Many NPs, including curcumin and resveratrol, suffer from poor bioavailability due to low intestinal absorption rates and extensive first-pass metabolism, which can result in the formation of inactive metabolites. Furthermore, their distribution to the CNS is often restricted by limited BBB permeability. Fortunately, strategies such as structural modification, formulation optimization, and advanced drug delivery systems (e.g., nanotechnology, drug carriers, micelles and cocrystals) have shown promise in overcoming these limitations by significantly improving bioavailability and BBB permeability (Liu M. et al., 2020; Mehmood et al., 2022; Rong et al., 2023; Xing et al., 2017).
Moreover, the inherent complexity and diversity of NPs structures pose challenges for isolating and extracting the specific active constituents responsible for the desired therapeutic effects. The lack of unified and standardized extraction and purification processes can lead to significant batch-to-batch variations in composition and activity, potentially impacting the efficacy and safety of NPs-based therapies. Therefore, establishing standardized protocols for NPs extraction and preparation is crucial for ensuring consistent product quality and facilitating clinical translation. Such standardization would enable more reliable assessment of efficacy and safety in clinical trials and support the development of well-defined, efficacious, and safe NPs-based therapeutics.
While our study highlights the therapeutic potential of NPs in mitigating abnormal α-Syn deposition, a significant knowledge gap exists regarding their precise mechanisms of action. Most studies have primarily focused on demonstrating a reduction in α-Syn aggregation, without delving into the underlying molecular mechanisms. Moreover, α-Syn aggregation is a dynamic, multi-step process, and current detection methods often fall short of capturing the entire aggregation and disaggregation process in real-time. This lack of in-depth mechanistic understanding and limitations in monitoring techniques hinder comprehensive assessment of the specific roles NPs play in inhibiting α-Syn aggregation.
In conclusion, the successful clinical translation of NPs for treating synucleinopathies like PD requires a multifaceted approach. Rigorous preclinical studies are essential for elucidating the precise molecular mechanisms of action, optimizing NPs formulations to enhance bioavailability and BBB permeability, and establishing standardized extraction and preparation processes to ensure product consistency. Furthermore, well-designed clinical trials are crucial for evaluating the therapeutic efficacy and safety of these NPs in humans. These trials should carefully consider appropriate dosage and administration routes, individual patient variability, long-term safety profiles, and potential pharmacological and toxicological interactions with other medications commonly prescribed for PD. Addressing these challenges will pave the way for harnessing the therapeutic potential of NPs and developing effective treatments for synucleinopathies.
Additionally, current research on NPs for PD relies heavily on preclinical models, including toxin-induced models (e.g., MPTP, 6-OHDA, paraquat), transgenic animal and cell models, and simpler organisms like C. elegans, Drosophila, and zebrafish. These models have proven valuable for studying specific aspects of PD pathogenesis, such as α-Syn misfolding, oxidative stress, mitochondrial dysfunction, and neuroinflammation. Studies utilizing these models have shown that NPs can modulate these pathological processes to varying degrees, leading to improvements in motor dysfunction. However, it is essential to recognize that no single model can fully recapitulate the complex PD phenotype observed in humans. Several factors contribute to the challenge of translating preclinical findings on NPs for PD into clinical applications. Species differences, variations in cellular microenvironments, and discrepancies between in vitro and in vivo conditions can all limit the translatability of preclinical data to humans. Moreover, inconsistencies in experimental design, such as variations in drug dosages, administration routes, treatment durations, and the lack of standardized outcome measures, can lead to conflicting results across studies, hindering the comparability and reliability of the findings. Research on the therapeutic effects of NPs for PD remains largely confined to preclinical cellular or animal models, with limited clinical data available to support their efficacy in humans. The scarcity of well-designed clinical trials represents a significant obstacle to advancing NPs-based therapies for PD.
7 Conclusion
The abnormal aggregation of α-Syn, culminating in the formation of Lewy bodies, represents a hallmark neuropathological feature of PD. The pervasive presence of α-Syn aggregates across diverse brain regions underscores the critical role of α-Syn in the pathogenesis of PD. The efficacy of current PD medications often diminishes over time as treatment duration increases and complications arise. This highlights the urgent need for novel therapeutic strategies that target the underlying mechanisms of disease onset and progression. NPs, derived from various sources in nature, are increasingly recognized as valuable sources of bioactive compounds for drug development and therapeutic applications. Recent research has unveiled a wide array of biological activities associated with NPs, including antioxidant, anti-inflammatory, antitumor, antibacterial, and immunomodulatory effects. Compared to synthetic drugs, NPs often exhibit greater structural complexity and exert their effects through multi-target mechanisms of action. This inherent characteristic of NPs often translates to a more favorable side effect profile, making them particularly attractive for treating complex diseases like PD, which involve intricate and interconnected pathological pathways.
Our study highlights the potential of various NPs in inhibiting the misfolding and abnormal aggregation of α-Syn (Figure 2B). Polyphenols, flavonoids, naphthoquinones, cinnamic acid, CA extracts, and natural alkaloids have emerged as promising candidates for PD therapy. These natural compounds exert anti-aggregation effects through multiple mechanisms, including slowing the nucleation rate of α-Syn, inhibiting oligomer formation, binding to intermediates to delay aggregate formation, destabilizing preformed α-Syn fibrils, and promoting the degradation and clearance of aggregates and fibrils. These multifaceted anti-aggregation properties of NPs offer a promising avenue for the prevention and treatment of PD.
MAO and COMT are important therapeutic targets for PD. Research has confirmed that curcumin, DHM, baicalein and EGCG exhibit potential in inhibiting COMT, while rutin, CAD, tanshinones, SHK, and CA extracts have shown potential in inhibiting MAO (Zheng et al., 2021; Kang et al., 2013; Prajapati et al., 2021; Takao et al., 2017; Zhao et al., 2021; Zhu and Jia, 2014), mean that new CMOT and MAO inhibitors can be developed and utilized on this basis.
We analyzed the existing clinical trials on the use of NPs for treating PD. Some studies have shown positive effects of Curcumin, nicotine, and caffeine, while others are negative. These discrepancies may be due to several factors, including the low absorption and metabolic efficiency, poor BBB permeability of many NPs in the body, or the use of inadequate doses and inappropriate administration methods in clinical trials, all of which can affect the bioactivity of the drugs and result in insufficient concentrations of active compounds to achieve the desired therapeutic effects. Additionally, in clinical settings, individual patient differences, experimental design, insufficient sample sizes, and inconsistent assessment methods can all impact the reliability and validity of results. And short-term observations may fail to capture significant therapeutic effects. Furthermore, multiple studies combining NPs with levodopa have shown that these supplements can effectively optimize levodopa’s efficacy and mitigate its side effects, particularly in cases of fluctuating clinical responses. Supplementation with polyphenolic compounds like naringin and curcumin at the onset of levodopa treatment shows promise as a strategy to potentially mitigate α-Syn aggregation and disease progression. Importantly, common structural motifs, such as quinones (six-membered rings with two double bonds and two ketones) and aromatic rings with hydroxyl groups, have been identified in several effective NPs (Caruana et al., 2011). These structural features appear to be crucial for inhibiting α-Syn aggregation and fibrillation, suggesting that they represent key pharmacophores for the development of novel α-Syn inhibitors. Further exploration of these structural motifs could provide valuable insights and guide drug discovery efforts for PD.
Emerging evidence suggests that therapeutic strategies targeting a single pathway may be insufficient for effectively treating complex diseases like PD. NPs, with their inherent multi-target pharmacological properties and neuroprotective effects, offer a promising alternative by potentially modulating multiple pathological pathways involved in PD pathogenesis. While the natural origin of NPs generally confers a degree of safety, several challenges hinder their clinical translation for treating neurodegenerative diseases like PD. Technical hurdles in extraction, purification, and structural characterization can impact standardization and quality control of NPs-based therapies. Furthermore, research on the pharmacokinetic properties, bioavailability, long-term safety, and potential toxicity of NPs in humans remains limited. Additionally, the BBB poses a significant obstacle to the delivery of many NPs to the brain, limiting their therapeutic efficacy. Developing novel strategies to enhance BBB permeability and facilitate efficient brain delivery of NPs and their bioactive constituents is crucial. Future research should focus on the neurobiological mechanisms by which NPs inhibit α-Syn aggregation and modulate pathways involved in neurodegeneration. Besides, conducting well-designed clinical trials to evaluate the safety, efficacy, optimal dosages, and long-term effects of promising NPs-based therapies in PD patients is needed for unlocking the full therapeutic potential of NPs and developing effective treatments for PD and other neurodegenerative disorders.
Author contributions
KY: Writing–review and editing, Writing–original draft, Visualization. ZL: Writing–review and editing, Writing–original draft, Visualization. WZ: Writing–review and editing, Visualization, Supervision. GL: Writing–review and editing, Visualization, Supervision. ChZ: Writing–original draft, Visualization. FQ: Writing–review and editing, Conceptualization. CuZ: Writing–review and editing, Conceptualization. KH: Writing–review and editing, Conceptualization. XC: Writing–review and editing, Visualization, Conceptualization. FF: Writing–review and editing, Visualization, Conceptualization. JL: Writing–review and editing, Visualization, Conceptualization. GX: Writing–review and editing, Project administration, Funding acquisition. HW: Writing–review and editing, Project administration, Funding acquisition. XW: Writing–review and editing, Project administration, Funding acquisition. WZ: Writing–review and editing, Writing–original draft, Supervision.
Funding
The author(s) declare that financial support was received for the research, authorship, and/or publication of this article. This study was funded by grants from the Medical and Health Research Project of Zhejiang Province (Grant No. 2022PY020), the Neurology Department of the National Key Clinical Speciality Construction Project, the Ningbo Major Research and Development Plan Project (Grant No. 2023Z196), and the Project of Ningbo Leading Medical and Health Discipline (Grant No. 2022-F05), the Ningbo Medical and Health Brand Discipline (Grant No. PPXK 2024-01) and the Key Project of Ningbo Science and Technology (2024Z184).
Conflict of interest
The authors declare that the research was conducted in the absence of any commercial or financial relationships that could be construed as a potential conflict of interest.
Publisher’s note
All claims expressed in this article are solely those of the authors and do not necessarily represent those of their affiliated organizations, or those of the publisher, the editors and the reviewers. Any product that may be evaluated in this article, or claim that may be made by its manufacturer, is not guaranteed or endorsed by the publisher.
References
Abounit, S., Bousset, L., Loria, F., Zhu, S., de Chaumont, F., Pieri, L., et al. (2016). Tunneling nanotubes spread fibrillar α-synuclein by intercellular trafficking of lysosomes. EMBO J. 35 (19), 2120–2138. doi:10.15252/embj.201593411
Ahmad, B., and Lapidus, L. J. (2012). Curcumin prevents aggregation in α-synuclein by increasing reconfiguration rate. J. Biol. Chem. 287 (12), 9193–9199. doi:10.1074/jbc.M111.325548
Ahsan, N., Mishra, S., Jain, M. K., Surolia, A., and Gupta, S. (2015). Curcumin pyrazole and its derivative (N-(3-Nitrophenylpyrazole)) curcumin inhibit aggregation, disrupt fibrils and modulate toxicity of wild type and mutant α-synuclein. Sci. Rep. 5, 9862. doi:10.1038/srep09862
Akagawa, M., Nakano, M., and Ikemoto, K. (2016). Recent progress in studies on the health benefits of pyrroloquinoline quinone. Biosci. Biotechnol. Biochem. 80 (1), 13–22. doi:10.1080/09168451.2015.1062715
Aliakbari, F., Mohammad-Beigi, H., Abbasi, S., Rezaei-Ghaleh, N., Lermyte, F., Parsafar, S., et al. (2021). Multiple protective roles of nanoliposome-incorporated baicalein against alpha-synuclein aggregates. Adv. Funct. Mater. 31 (7), 2007765. doi:10.1002/adfm.202007765
Alıcı, H. (2021). Investigation of inhibition effect of nicotine and dopamine on alpha-synuclein. J. Comput. Biophysics Chem. 20 (05), 477–494. doi:10.1142/S2737416521500265
Almeida, Z. L., and Brito, R. M. M. (2020). Structure and aggregation mechanisms in amyloids. Molecules 25 (5), 1195. doi:10.3390/molecules25051195
Aminin, D., and Polonik, S. (2020). 1,4-Naphthoquinones: some biological properties and application. Chem. Pharm. Bull. 68 (1), 46–57. doi:10.1248/cpb.c19-00911
Anand, P., Kunnumakkara, A. B., Newman, R. A., and Aggarwal, B. B. (2007). Bioavailability of curcumin: problems and promises. Mol. Pharm. 4 (6), 807–818. doi:10.1021/mp700113r
Andrich, K., and Bieschke, J. (2015). “The effect of (-)-Epigallo-Catechin-(3)-Gallate on amyloidogenic proteins suggests a common mechanism,” in Natural compounds as therapeutic agents for amyloidogenic diseases. Editor N. Vassallo (Berlin: Springer-Verlag Berlin), 139–161. Available at: https://webofscience.clarivate.cn/wos/alldb/full-record/WOS:000361795900008 (Accessed May 15, 2024).
Andújar, I., Recio, M. C., Giner, R. M., and Ríos, J. L. (2013). Traditional Chinese medicine remedy to jury: the pharmacological basis for the use of shikonin as an anticancer therapy. Curr. Med. Chem. 20 (23), 2892–2898. doi:10.2174/09298673113209990008
Anjaneyulu, J., R, V., and Godbole, A. (2020). Differential effect of ayurvedic nootropics on C. Elegans models of Parkinson’s disease. J. Ayurveda Integr. Med. 11 (4), 440–447. doi:10.1016/j.jaim.2020.07.006
Antunes, M. S., Ladd, F. V. L., Ladd, A. A. B. L., Moreira, A. L., Boeira, S. P., and Cattelan Souza, L. (2021). Hesperidin protects against behavioral alterations and loss of dopaminergic neurons in 6-OHDA-lesioned mice: the role of mitochondrial dysfunction and apoptosis. Metab. Brain Dis. 36 (1), 153–167. doi:10.1007/s11011-020-00618-y
Ardah, M. T., Ghanem, S. S., Abdulla, S. A., Lv, G., Emara, M. M., Paleologou, K. E., et al. (2020). Inhibition of alpha-synuclein seeded fibril formation and toxicity by herbal medicinal extracts. BMC Complementary Med. Ther. 20 (1), 73. doi:10.1186/s12906-020-2849-1
Bae, E. A., Han, M. J., Lee, K. T., Choi, J. W., Park, H. J., and Kim, D. H. (1999). Metabolism of 6″-O-xylosyltectoridin and tectoridin by human intestinal bacteria and their hypoglycemic and in vitro cytotoxic activities. Biol. and Pharm. Bull. 22 (12), 1314–1318. doi:10.1248/bpb.22.1314
Balestrino, R., and Martinez-Martin, P. (2017). Neuropsychiatric symptoms, behavioural disorders, and quality of life in Parkinson’s disease. J. Neurological Sci. 373, 173–178. doi:10.1016/j.jns.2016.12.060
Bartels, T., Choi, J. G., and Selkoe, D. J. (2011). α-Synuclein occurs physiologically as a helically folded tetramer that resists aggregation. Nature 477 (7362), 107–110. doi:10.1038/nature10324
Bastianetto, S., Ménard, C., and Quirion, R. (2015). Neuroprotective action of resveratrol. Biochimica Biophysica Acta 1852 (6), 1195–1201. doi:10.1016/j.bbadis.2014.09.011
Beach, T. G., Adler, C. H., Sue, L. I., Vedders, L., Lue, L., White Iii, C. L., et al. (2010). Multi-organ distribution of phosphorylated alpha-synuclein histopathology in subjects with Lewy body disorders. Acta Neuropathol. 119 (6), 689–702. doi:10.1007/s00401-010-0664-3
Berrocal, R., Vasudevaraju, P., Indi, S. S., Sambasiva Rao, K. R. S., and Rao, K. S. (2014). In vitro evidence that an aqueous extract of Centella asiatica modulates α-synuclein aggregation dynamics. J. Alzheimer’s Dis. 39 (2), 457–465. doi:10.3233/JAD-131187
Bieschke, J., Russ, J., Friedrich, R. P., Ehrnhoefer, D. E., Wobst, H., Neugebauer, K., et al. (2010). EGCG remodels mature alpha-synuclein and amyloid-beta fibrils and reduces cellular toxicity. Proc. Natl. Acad. Sci. U. S. A. 107 (17), 7710–7715. doi:10.1073/pnas.0910723107
Billowria, K., Ali, R., Rangra, N. K., Kumar, R., and Chawla, A. (2024). Bioactive flavonoids: a comprehensive review on pharmacokinetics and analytical aspects. Crit. Rev. Anal. Chem. 54, 1002–1016. doi:10.1080/10408347.2022.2105641
Bloem, B. R., Okun, M. S., and Klein, C. (2021). Parkinson’s disease. Lancet London, Engl. 397 (10291), 2284–2303. doi:10.1016/S0140-6736(21)00218-X
Boh, B., Berovic, M., Zhang, J., and Zhi-Bin, L. (2007). Ganoderma lucidum and its pharmaceutically active compounds. Biotechnol. Annu. Rev. 13, 265–301. doi:10.1016/S1387-2656(07)13010-6
Bonifácio, M. J., Palma, P. N., Almeida, L., and Soares-da-Silva, P. (2007). Catechol-O-Methyltransferase and its inhibitors in Parkinson’s disease. CNS Drug Rev. 13 (3), 352–379. doi:10.1111/j.1527-3458.2007.00020.x
Brunel, J. M., Salmi, C., Loncle, C., Vidal, N., and Letourneux, Y. (2005). Squalamine: a polyvalent drug of the future? Curr. Cancer Drug Targets 5 (4), 267–272. doi:10.2174/1568009054064642
Burré, J., Sharma, M., and Südhof, T. C. (2018). Cell biology and pathophysiology of α-synuclein. Cold Spring Harb. Perspect. Med. 8 (3), a024091. doi:10.1101/cshperspect.a024091
Cai, C.-Z., Zhou, H.-F., Yuan, N.-N., Wu, M.-Y., Lee, S. M.-Y., Ren, J.-Y., et al. (2019). Natural alkaloid harmine promotes degradation of alpha-synuclein via PKA-mediated ubiquitin-proteasome system activation. Phytomedicine 61, 152842. doi:10.1016/j.phymed.2019.152842
Calamini, B., Ratia, K., Malkowski, M. G., Cuendet, M., Pezzuto, J. M., Santarsiero, B. D., et al. (2010). Pleiotropic mechanisms facilitated by resveratrol and its metabolites. Biochem. J. 429 (2), 273–282. doi:10.1042/BJ20091857
Cali, T., Ottolini, D., Negro, A., and Brini, M. (2012). α-Synuclein controls mitochondrial calcium homeostasis by enhancing endoplasmic reticulum-mitochondria interactions. J. Biol. Chem. 287 (22), 17914–17929. doi:10.1074/jbc.M111.302794
Carradori, S., Gidaro, M. C., Petzer, A., Costa, G., Guglielmi, P., Chimenti, P., et al. (2016). Inhibition of human monoamine oxidase: biological and molecular modeling studies on selected natural flavonoids. J. Agric. Food Chem. 64 (47), 9004–9011. doi:10.1021/acs.jafc.6b03529
Caruana, M., Högen, T., Levin, J., Hillmer, A., Giese, A., and Vassallo, N. (2011). Inhibition and disaggregation of α-synuclein oligomers by natural polyphenolic compounds. FEBS Lett. 585 (8), 1113–1120. doi:10.1016/j.febslet.2011.03.046
Chao, Y. X., Chew, L. M., Deng, X., and Tan, E.-K. (2015). Nonmotor symptoms in sporadic versus familial forms of Parkinson’s disease. Neurodegener. Dis. Manag. 5 (2), 147–153. doi:10.2217/nmt.14.57
Chau, E., Kim, H., Shin, J., Martinez, A., and Kim, J. R. (2021). Inhibition of alpha-synuclein aggregation by AM17, a synthetic resveratrol derivative. Biochem. Biophysical Res. Commun. 574, 85–90. doi:10.1016/j.bbrc.2021.08.049
Chen, J.-F., Xu, K., Petzer, J. P., Staal, R., Xu, Y.-H., Beilstein, M., et al. (2001). Neuroprotection by caffeine and A2A adenosine receptor inactivation in a model of Parkinson’s disease. J. Neurosci. 21 (10), RC143. doi:10.1523/JNEUROSCI.21-10-j0001.2001
Chen, L.-L., Song, J.-X., Lu, J.-H., Yuan, Z.-W., Liu, L.-F., Durairajan, S. S. K., et al. (2014). Corynoxine, a natural autophagy enhancer, promotes the clearance of alpha-synuclein via akt/mTOR pathway. J. Neuroimmune Pharmacol. 9 (3), 380–387. doi:10.1007/s11481-014-9528-2
Chen, M., Wang, T., Yue, F., Li, X., Wang, P., Li, Y., et al. (2015). Tea polyphenols alleviate motor impairments, dopaminergic neuronal injury, and cerebral α-synuclein aggregation in MPTP-intoxicated parkinsonian monkeys. Neuroscience 286, 383–392. doi:10.1016/j.neuroscience.2014.12.003
Chen, X., Oppenheim, J., and Howard, O. M. (2001). Shikonin, a component of antiinflammatory Chinese herbal medicine, selectively blocks chemokine binding to CC chemokine receptor-1. Int. Immunopharmacol. 1 (2), 229–236. doi:10.1016/s1567-5769(00)00033-3
Cheng, A. L., Hsu, C. H., Lin, J. K., Hsu, M. M., Ho, Y. F., Shen, T. S., et al. (2001). Phase I clinical trial of curcumin, a chemopreventive agent, in patients with high-risk or pre-malignant lesions. Anticancer Res. 21 (4B), 2895–2900.
Cho, J. (2006). Antioxidant and neuroprotective effects of hesperidin and its aglycone hesperetin. Archives Pharmacal Res. 29 (8), 699–706. doi:10.1007/BF02968255
Christmann, A., Gries, M., Scholz, P., Stahr, P. L., Law, J. K. Y., Schulte, S., et al. (2022). The antioxidant rutin counteracts the pathological impact of α-synuclein on the enteric nervous system in vitro. Biol. Chem. 403 (1), 103–122. doi:10.1515/hsz-2021-0259
Costa, H. N., Esteves, A. R., Empadinhas, N., and Cardoso, S. M. (2022). Parkinson’s disease: a multisystem disorder. Neurosci. Bull. 39 (1), 113–124. doi:10.1007/s12264-022-00934-6
Cuervo, A. M., Stefanis, L., Fredenburg, R., Lansbury, P. T., and Sulzer, D. (2004). Impaired degradation of mutant alpha-synuclein by chaperone-mediated autophagy. Sci. (New York, N.Y.) 305 (5688), 1292–1295. doi:10.1126/science.1101738
Danyu, L., Yanran, L., Xiuna, J., Ying, C., Sudan, P., Tianen, Z., et al. (2019). α-Synuclein induced mitochondrial dysfunction via cytochrome c oxidase subunit 2 in SH-SY5Y cells. Exp. Cell Res. 378 (1), 57–65. doi:10.1016/j.yexcr.2019.02.006
da Silva, F. L., Coelho Cerqueira, E., de Freitas, M. S., Gonçalves, D. L., Costa, L. T., and Follmer, C. (2013). Vitamins K interact with N-terminus α-synuclein and modulate the protein fibrillization in vitro. Exploring the interaction between quinones and α-synuclein. Neurochem. Int. 62 (1), 103–112. doi:10.1016/j.neuint.2012.10.001
Davidson, W. S., Jonas, A., Clayton, D. F., and George, J. M. (1998). Stabilization of alpha-synuclein secondary structure upon binding to synthetic membranes. J. Biol. Chem. 273 (16), 9443–9449. doi:10.1074/jbc.273.16.9443
de Araújo, F. F., de Paulo Farias, D., Neri-Numa, I. A., and Pastore, G. M. (2021). Polyphenols and their applications: an approach in food chemistry and innovation potential. Food Chem. 338, 127535. doi:10.1016/j.foodchem.2020.127535
Delgado-Minjares, K. M., Martinez-Fong, D., Martínez-Dávila, I. A., Bañuelos, C., Gutierrez-Castillo, M. E., Blanco-Alvarez, V. M., et al. (2021). Mechanistic insight from preclinical models of Parkinson’s disease could help redirect clinical trial efforts in GDNF therapy. Int. J. Mol. Sci. 22 (21), 11702. doi:10.3390/ijms222111702
Diachenko, A. I., Rodin, I. A., Krasnova, T. N., Klychnikov, O. I., and Nefedova, L. N. (2024). The role of vitamin K in the development of neurodegenerative diseases. Biochem. Biokhimiia 89 (Suppl. 1), S57–S70. doi:10.1134/S0006297924140049
Dickson, D. W. (2012). Parkinson’s disease and parkinsonism: neuropathology. Cold Spring Harb. Perspect. Med. 2 (8), a009258. doi:10.1101/cshperspect.a009258
DiFrancisco-Donoghue, J., Jung, M.-K., and Leder, A. (2019). Nicotine gum as a therapeutic approach for low blood pressure in Parkinson’s disease: a randomized pilot study. Nicotine and Tob. Res. 21 (2), 253–256. doi:10.1093/ntr/ntx263
Dikiy, I., and Eliezer, D. (2014). N-terminal acetylation stabilizes N-terminal helicity in lipid- and micelle-bound α-synuclein and increases its affinity for physiological membranes. J. Biol. Chem. 289 (6), 3652–3665. doi:10.1074/jbc.M113.512459
Dominguez-Meijide, A., Vasili, E., König, A., Cima-Omori, M.-S., Ibáñez de Opakua, A., Leonov, A., et al. (2020). Effects of pharmacological modulators of α-synuclein and tau aggregation and internalization. Sci. Rep. 10, 12827. doi:10.1038/s41598-020-69744-y
Donadio, V., Incensi, A., Rizzo, G., Fileccia, E., Ventruto, F., Riva, A., et al. (2022). The effect of curcumin on idiopathic Parkinson disease: a clinical and skin biopsy study. J. Neuropathology Exp. Neurology 81 (7), 545–552. doi:10.1093/jnen/nlac034
Dorsey, E. R., Sherer, T., Okun, M. S., and Bloem, B. R. (2018). The emerging evidence of the Parkinson pandemic. J. Parkinson’s Dis. 8 (Suppl. 1), S3–S8. doi:10.3233/JPD-181474
El-Agnaf, O. M. A., and Irvine, G. B. (2002). Aggregation and neurotoxicity of alpha-synuclein and related peptides. Biochem. Soc. Trans. 30, 559–565. doi:10.1042/bst0300559
Elkon, H., Don, J., Melamed, E., Ziv, I., Shirvan, A., and Offen, D. (2002). Mutant and wild-type alpha-synuclein interact with mitochondrial cytochrome C oxidase. J. Mol. Neurosci. MN 18 (3), 229–238. doi:10.1385/JMN:18:3:229
Ellis, C. E., Murphy, E. J., Mitchell, D. C., Golovko, M. Y., Scaglia, F., Barceló-Coblijn, G. C., et al. (2005). Mitochondrial lipid abnormality and electron transport chain impairment in mice lacking alpha-synuclein. Mol. Cell. Biol. 25 (22), 10190–10201. doi:10.1128/MCB.25.22.10190-10201.2005
Emekli-Alturfan, E., and Alturfan, A. A. (2023). The emerging relationship between vitamin K and neurodegenerative diseases: a review of current evidence. Mol. Biol. Rep. 50 (1), 815–828. doi:10.1007/s11033-022-07925-w
Enogieru, A. B., Haylett, W., Hiss, D. C., Bardien, S., and Ekpo, O. E. (2018). Rutin as a potent antioxidant: implications for neurodegenerative disorders. Oxidative Med. Cell. Longev. 2018, 6241017. doi:10.1155/2018/6241017
Evans, J. A., Mendonca, P., and Soliman, K. F. A. (2022). Neuroprotective effects and therapeutic potential of the citrus flavonoid hesperetin in neurodegenerative diseases. Nutrients 14 (11), 2228. doi:10.3390/nu14112228
Farrell, T. L., Gomez-Juaristi, M., Poquet, L., Redeuil, K., Nagy, K., Renouf, M., et al. (2012). Absorption of dimethoxycinnamic acid derivatives in vitro and pharmacokinetic profile in human plasma following coffee consumption. Mol. Nutr. and Food Res. 56 (9), 1413–1423. doi:10.1002/mnfr.201200021
Fox, S. H., Katzenschlager, R., Lim, S.-Y., Barton, B., de Bie, R. M. A., Seppi, K., et al. (2018). International Parkinson and movement disorder society evidence-based medicine review: update on treatments for the motor symptoms of Parkinson’s disease. Mov. Disord. 33 (8), 1248–1266. doi:10.1002/mds.27372
Fredholm, B. B., Bättig, K., Holmén, J., Nehlig, A., and Zvartau, E. E. (1999). Actions of caffeine in the brain with special reference to factors that contribute to its widespread use. Pharmacol. Rev. 51, 83–133.
Gadad, B. S., Subramanya, P. K., Pullabhatla, S., Shantharam, I. S., and Rao, K. S. (2012). Curcumin-glucoside, a novel synthetic derivative of curcumin, inhibits α-synuclein oligomer formation: relevance to Parkinson’s disease. Curr. Pharm. Des. 18 (1), 76–84. doi:10.2174/138161212798919093
Gadahad, M. R. K., Rao, M., and Rao, G. (2008). Enhancement of hippocampal CA3 neuronal dendritic arborization by Centella asiatica (linn) fresh leaf extract treatment in adult rats. J. Chin. Med. Assoc. JCMA 71 (1), 6–13. doi:10.1016/s1726-4901(08)70066-2
Ganeshpurkar, A., and Saluja, A. K. (2017). The pharmacological potential of rutin. Saudi Pharm. J. SPJ 25 (2), 149–164. doi:10.1016/j.jsps.2016.04.025
Ghanem, S. S., Fayed, H. S., Zhu, Q., Lu, J.-H., Vaikath, N. N., Ponraj, J., et al. (2021). Natural alkaloid compounds as inhibitors for alpha-synuclein seeded fibril formation and toxicity. Molecules 26 (12), 3736. doi:10.3390/molecules26123736
Ghio, S., Camilleri, A., Caruana, M., Ruf, V. C., Schmidt, F., Leonov, A., et al. (2019). Cardiolipin promotes pore-forming activity of alpha-synuclein oligomers in mitochondrial membranes. ACS Chem. Neurosci. 10 (8), 3815–3829. doi:10.1021/acschemneuro.9b00320
Ghodsi, H., Rahimi, H. R., Aghili, S. M., Saberi, A., and Shoeibi, A. (2022). Evaluation of curcumin as add-on therapy in patients with Parkinson’s disease: a pilot randomized, triple-blind, placebo-controlled trial. Clin. Neurology Neurosurg. 218, 107300. doi:10.1016/j.clineuro.2022.107300
Goel, A., Kunnumakkara, A. B., and Aggarwal, B. B. (2008). Curcumin as “curecumin”: from kitchen to clinic. Biochem. Pharmacol. 75 (4), 787–809. doi:10.1016/j.bcp.2007.08.016
Gong, P., Deng, F., Zhang, W., Ji, J., Liu, J., Sun, Y., et al. (2017). Tectorigenin attenuates the MPP+-Induced SH-SY5Y cell damage, indicating a potential beneficial role in Parkinson’s disease by oxidative stress inhibition. Exp. Ther. Med. 14 (5), 4431–4437. doi:10.3892/etm.2017.5049
Grey, M., Dunning, C. J., Gaspar, R., Grey, C., Brundin, P., Sparr, E., et al. (2015). Acceleration of α-synuclein aggregation by exosomes. J. Biol. Chem. 290 (5), 2969–2982. doi:10.1074/jbc.M114.585703
Guo, S.-S., Cui, X.-L., and Rausch, W.-D. (2016). Ganoderma lucidum polysaccharides protect against MPP(+) and rotenone-induced apoptosis in primary dopaminergic cell cultures through inhibiting oxidative stress. Am. J. Neurodegener. Dis. 5 (2), 131–144.
Guo, Y.-J., Dong, S.-Y., Cui, X.-X., Feng, Y., Liu, T., Yin, M., et al. (2016). Resveratrol alleviates MPTP-induced motor impairments and pathological changes by autophagic degradation of α-synuclein via SIRT1-deacetylated LC3. Mol. Nutr. and Food Res. 60 (10), 2161–2175. doi:10.1002/mnfr.201600111
Hajialuani, M., Farzaei, M. H., Echeverria, J., Nabavi, S. M., Uriarte, E., and Sobarzo-Sanchez, E. (2019). Hesperidin as a neuroprotective agent: a review of animal and clinical evidence. Mol. Basel, Switz. 24 (3), 648. doi:10.3390/molecules24030648
Halestrap, A. P. (2009). What is the mitochondrial permeability transition pore? J. Mol. Cell. Cardiol. 46 (6), 821–831. doi:10.1016/j.yjmcc.2009.02.021
Han, H., Weinreb, P. H., and Lansbury, P. T. (1995). The core alzheimer’s peptide NAC forms amyloid fibrils which seed and are seeded by beta-amyloid: is NAC a common trigger or target in neurodegenerative disease? Chem. and Biol. 2 (3), 163–169. doi:10.1016/1074-5521(95)90071-3
Hao, D., Hammond, L. A., Eckhardt, S. G., Patnaik, A., Takimoto, C. H., Schwartz, G. H., et al. (2003). A phase I and pharmacokinetic study of squalamine, an aminosterol angiogenesis inhibitor. Clin. Cancer Res. 9 (7), 2465–2471.
Hauser, R. A., Sutherland, D., Madrid, J. A., Rol, M. A., Frucht, S., Isaacson, S., et al. (2019). Targeting neurons in the gastrointestinal tract to treat Parkinson’s disease. Clin. Park. and Relat. Disord. 1, 2–7. doi:10.1016/j.prdoa.2019.06.001
He, Z., Hu, Y., Niu, Z., Zhong, K., Liu, T., Yang, M., et al. (2023). A review of pharmacokinetic and pharmacological properties of asiaticoside, a major active constituent of Centella asiatica (L.) Urb. J. Ethnopharmacol. 302, 115865. doi:10.1016/j.jep.2022.115865
Holmes, A. D., Copland, D. A., Silburn, P. A., and Chenery, H. J. (2011a). Acute nicotine enhances strategy-based semantic processing in Parkinson’s disease. Int. J. Neuropsychopharmacol. 14 (7), 877–885. doi:10.1017/S1461145710001665
Holmes, A. D., Copland, D. A., Silburn, P. A., and Chenery, H. J. (2011b). Nicotine effects on general semantic priming in Parkinson’s disease. Exp. Clin. Psychopharmacol. 19 (3), 215–223. doi:10.1037/a0023117
Holmqvist, S., Chutna, O., Bousset, L., Aldrin-Kirk, P., Li, W., Björklund, T., et al. (2014). Direct evidence of Parkinson pathology spread from the gastrointestinal tract to the brain in rats. Acta Neuropathol. 128 (6), 805–820. doi:10.1007/s00401-014-1343-6
Hong, D.-P., Fink, A. L., and Uversky, V. N. (2008). Structural characteristics of alpha-synuclein oligomers stabilized by the flavonoid baicalein. J. Mol. Biol. 383 (1), 214–223. doi:10.1016/j.jmb.2008.08.039
Hong, D.-P., Fink, A. L., and Uversky, V. N. (2009). Smoking and Parkinson’s disease: does nicotine affect α-synuclein fibrillation? Biochimica Biophysica Acta-Proteins Proteomics 1794 (2), 282–290. doi:10.1016/j.bbapap.2008.09.026
Hong, J., Shin, K. H., Lim, S. S., Kwak, J. H., Zee, O., Ishihara, K., et al. (2008). Lead compounds for anti-inflammatory drugs isolated from the plants of the traditional oriental medicine in korea. Inflamm. and Allergy Drug Targets 7 (3), 195–202. doi:10.2174/187152808785748100
Houser, M. C., and Tansey, M. G. (2017). The gut-brain Axis: is intestinal inflammation a silent driver of Parkinson’s disease pathogenesis? NPJ Parkinson’s Dis. 3 (3), 3. doi:10.1038/s41531-016-0002-0
Hu, Q., Uversky, V. N., Huang, M., Kang, H., Xu, F., Liu, X., et al. (2016). Baicalein inhibits α-synuclein oligomer formation and prevents progression of α-synuclein accumulation in a rotenone mouse model of Parkinson’s disease. Biochimica Biophysica Acta-Molecular Basis Dis. 1862 (10), 1883–1890. doi:10.1016/j.bbadis.2016.07.008
Huang, W., Zhang, H., Liu, W., and Li, C. (2012). Survey of antioxidant capacity and phenolic composition of blueberry, blackberry, and strawberry in nanjing. J. Zhejiang Univ. Sci. B 13 (2), 94–102. doi:10.1631/jzus.B1100137
Hui, K. Y. (2020). Investigating the role of alpha synuclein in mitochondrial dysfunction using IPSC-derived induced neurons, PQDT - global. Singapore: National University of Singapore.
Hukkanen, J., Jacob, P., and Benowitz, N. L. (2005). Metabolism and disposition kinetics of nicotine. Pharmacol. Rev. 57 (1), 79–115. doi:10.1124/pr.57.1.3
Hung, K.-C., Huang, H.-J., Wang, Y.-T., and Lin, A. M.-Y. (2016). Baicalein attenuates α-synuclein aggregation, inflammasome activation and autophagy in the MPP+-Treated nigrostriatal dopaminergic system in vivo. J. Ethnopharmacol. 194, 522–529. doi:10.1016/j.jep.2016.10.040
Ibarra-Gutiérrez, M. T., Serrano-García, N., and Orozco-Ibarra, M. (2023). Rotenone-induced model of Parkinson’s disease: beyond mitochondrial complex I inhibition. Mol. Neurobiol. 60 (4), 1929–1948. doi:10.1007/s12035-022-03193-8
Illes-Toth, E., Rempel, D. L., and Gross, M. L. (2024). Exploration of resveratrol as a potent modulator of α-synuclein fibril formation. ACS Chem. Neurosci. 15 (3), 503–516. doi:10.1021/acschemneuro.3c00571
Innos, J., and Hickey, M. A. (2021). Using rotenone to model Parkinson’s disease in mice: a review of the role of pharmacokinetics. Chem. Res. Toxicol. 34 (5), 1223–1239. doi:10.1021/acs.chemrestox.0c00522
Ishola, I. O., Afolayan, O., Odutola, I., Faniyan, O., and Adeyemi, O. (2021). Therapeutic potential of hesperidin in Parkinson’s disease with dementia: inhibition of alpha synuclein and amyloid beta in Drosophila melanogaster. Niger. J. Physiological Sci. 36 (1), 43–48.
Ishola, I. O., Jacinta, A. A., and Adeyemi, O. O. (2019). Cortico-hippocampal memory enhancing activity of hesperetin on scopolamine-induced amnesia in mice: role of antioxidant defense system, cholinergic neurotransmission and expression of BDNF. Metab. Brain Dis. 34 (4), 979–989. doi:10.1007/s11011-019-00409-0
Itti, E., Villafane, G., Malek, Z., Brugières, P., Capacchione, D., Itti, L., et al. (2009). Dopamine transporter imaging under high-dose transdermal nicotine therapy in Parkinson’s disease: an observational study. Nucl. Med. Commun. 30 (7), 513–518. doi:10.1097/MNM.0b013e32832cc204
Javed, H., Khan, M. M., Ahmad, A., Vaibhav, K., Ahmad, M. E., Khan, A., et al. (2012). Rutin prevents cognitive impairments by ameliorating oxidative stress and neuroinflammation in rat model of sporadic dementia of alzheimer type. Neuroscience 210, 340–352. doi:10.1016/j.neuroscience.2012.02.046
Jha, N. N., Ghosh, D., Das, S., Anoop, A., Jacob, R. S., Singh, P. K., et al. (2016). Effect of curcumin analogs onα-synuclein aggregation and cytotoxicity. Sci. Rep. 6, 28511. doi:10.1038/srep28511
Jha, N. N., Kumar, R., Panigrahi, R., Navalkar, A., Ghosh, D., Sahay, S., et al. (2017). Comparison of α-synuclein fibril inhibition by four different amyloid inhibitors. ACS Chem. Neurosci. 8 (12), 2722–2733. doi:10.1021/acschemneuro.7b00261
Ji, K., Zhao, Y., Yu, T., Wang, Z., Gong, H., Yang, X., et al. (2016). Inhibition effects of tanshinone on the aggregation of α-synuclein. Food and Funct. 7 (1), 409–416. doi:10.1039/C5FO00664C
Jia, L., Wang, Y., Sang, J., Cui, W., Zhao, W., Wei, W., et al. (2019). Dihydromyricetin inhibits α-synuclein aggregation, disrupts preformed fibrils, and protects neuronal cells in culture against amyloid-induced cytotoxicity. J. Agric. Food Chem. 67 (14), 3946–3955. doi:10.1021/acs.jafc.9b00922
Jiang, C.-P., Ding, H., Shi, D.-H., Wang, Y.-R., Li, E.-G., and Wu, J.-H. (2012). Pro-apoptotic effects of tectorigenin on human hepatocellular carcinoma HepG2 cells. World J. Gastroenterology WJG 18 (15), 1753–1764. doi:10.3748/wjg.v18.i15.1753
Jiang, M., Porat-Shliom, Y., Pei, Z., Cheng, Y., Xiang, L., Sommers, K., et al. (2010). Baicalein reduces E46K α-synuclein aggregation in vitro and protects cells against E46K α-synuclein toxicity in cell models of familiar parkinsonism. J. Neurochem. 114 (2), 419–429. doi:10.1111/j.1471-4159.2010.06752.x
Jin, F., Wu, Q., Lu, Y.-F., Gong, Q.-H., and Shi, J.-S. (2008). Neuroprotective effect of resveratrol on 6-OHDA-induced Parkinson’s disease in rats. Eur. J. Pharmacol. 600 (1–3), 78–82. doi:10.1016/j.ejphar.2008.10.005
Joo, Y.-E. (2014). Natural product-derived drugs for the treatment of inflammatory bowel diseases. Intestinal Res. 12 (2), 103–109. doi:10.5217/ir.2014.12.2.103
Jung, J.-C., Lim, E., Lee, Y., Kang, J.-M., Kim, H., Jang, S., et al. (2009). Synthesis of novel trans-stilbene derivatives and evaluation of their potent antioxidant and neuroprotective effects. Eur. J. Med. Chem. 44 (8), 3166–3174. doi:10.1016/j.ejmech.2009.03.011
Kalgaonkar, S., Gross, H. B., Yokoyama, W., and Keen, C. L. (2010). Effects of a flavonol-rich diet on select cardiovascular parameters in a golden Syrian hamster model. J. Med. Food 13 (1), 108–115. doi:10.1089/jmf.2008.0295
Kam, T.-I., Hinkle, J. T., Dawson, T. M., and Dawson, V. L. (2020). Microglia and astrocyte dysfunction in Parkinson’s disease. Neurobiol. Dis. 144, 105028. doi:10.1016/j.nbd.2020.105028
Kamalakkannan, N., and Prince, P. S. M. (2006). Antihyperglycaemic and antioxidant effect of rutin, a polyphenolic flavonoid, in streptozotocin-induced diabetic wistar rats. Basic and Clin. Pharmacol. and Toxicol. 98 (1), 97–103. doi:10.1111/j.1742-7843.2006.pto_241.x
Kanaze, F. I., Bounartzi, M. I., Georgarakis, M., and Niopas, I. (2007). Pharmacokinetics of the citrus flavanone aglycones hesperetin and naringenin after single oral administration in human subjects. Eur. J. Clin. Nutr. 61 (4), 472–477. doi:10.1038/sj.ejcn.1602543
Kang, K. S., Yamabe, N., Wen, Y., Fukui, M., and Zhu, B. T. (2013). Beneficial effects of natural phenolics on levodopa methylation and oxidative neurodegeneration. Brain Res. 1497, 1–14. doi:10.1016/j.brainres.2012.11.043
Kardani, J., and Roy, I. (2015). Understanding caffeine’s role in attenuating the toxicity of α-synuclein aggregates: implications for risk of Parkinson’s disease. ACS Chem. Neurosci. 6 (9), 1613–1625. doi:10.1021/acschemneuro.5b00158
Kardani, J., Sethi, R., and Roy, I. (2017). Nicotine slows down oligomerisation of α-synuclein and ameliorates cytotoxicity in a yeast model of Parkinson’s disease. Biochimica Biophysica Acta (BBA) - Mol. Basis Dis. 1863 (6), 1454–1463. doi:10.1016/j.bbadis.2017.02.002
Kaushik, S., and Cuervo, A. M. (2008). Chaperone-mediated autophagy. Methods Mol. Biol. Clift. N.J. 445, 227–244. doi:10.1007/978-1-59745-157-4_15
Keihanian, F., Saeidinia, A., Bagheri, R. K., Johnston, T. P., and Sahebkar, A. (2018). Curcumin, hemostasis, thrombosis, and coagulation. J. Cell. Physiology 233 (6), 4497–4511. doi:10.1002/jcp.26249
Kesh, S., Kannan, R. R., Sivaji, K., and Balakrishnan, A. (2021). Hesperidin downregulates kinases Lrrk2 and Gsk3β in a 6-OHDA induced Parkinson’s disease model. Neurosci. Lett. 740, 135426. doi:10.1016/j.neulet.2020.135426
Khan, M. M., Ahmad, A., Ishrat, T., Khuwaja, G., Srivastawa, P., Khan, M. B., et al. (2009). Rutin protects the neural damage induced by transient focal ischemia in rats. Brain Res. 1292, 123–135. doi:10.1016/j.brainres.2009.07.026
Kim, D. W., Hwang, I. K., Lim, S. S., Yoo, K.-Y., Li, H., Kim, Y. S., et al. (2009). Germinated buckwheat extract decreases blood pressure and nitrotyrosine immunoreactivity in aortic endothelial cells in spontaneously hypertensive rats. Phytotherapy Res. PTR 23 (7), 993–998. doi:10.1002/ptr.2739
Kim, J., Kobayashi, M., Fukuda, M., Ogasawara, D., Kobayashi, N., Han, S., et al. (2010). Pyrroloquinoline quinone inhibits the fibrillation of amyloid proteins. Prion 4 (1), 26–31. doi:10.4161/pri.4.1.10889
Kim, T. D., Paik, S. R., and Yang, C.-H. (2002). Structural and functional implications of C-terminal regions of alpha-synuclein. Biochemistry 41 (46), 13782–13790. doi:10.1021/bi026284c
Kitagawa, M., Houzen, H., and Tashiro, K. (2007). Effects of caffeine on the freezing of gait in Parkinson’s disease. Mov. Disord. Official J. Mov. Disord. Soc. 22 (5), 710–712. doi:10.1002/mds.21208
Kobayashi, M., Kim, J., Kobayashi, N., Han, S., Nakamura, C., Ikebukuro, K., et al. (2006). Pyrroloquinoline quinone (PQQ) prevents fibril formation of alpha-synuclein. Biochem. Biophysical Res. Commun. 349 (3), 1139–1144. doi:10.1016/j.bbrc.2006.08.144
Kreft, S., Knapp, M., and Kreft, I. (1999). Extraction of rutin from buckwheat (fagopyrum esculentumMoench) seeds and determination by capillary electrophoresis. J. Agric. Food Chem. 47 (11), 4649–4652. doi:10.1021/jf990186p
Krüger, R., Kuhn, W., Müller, T., Woitalla, D., Graeber, M., Kösel, S., et al. (1998). Ala30Pro mutation in the gene encoding alpha-synuclein in Parkinson's disease. Nat. Genet. 18 (2), 106–108. doi:10.1038/ng0298-106
Kulisevsky, J., Barbanoj, M., Gironell, A., Antonijoan, R., Casas, M., and Pascual-Sedano, B. (2002). A double-blind crossover, placebo-controlled study of the adenosine A2A antagonist theophylline in Parkinson’s disease. Clin. Neuropharmacol. 25 (1), 25–31. doi:10.1097/00002826-200201000-00005
Kyaw, W. T., Nagai, M., Kaneta, M., Kubo, M., Nishikawa, N., Tsujii, T., et al. (2013). Effect of nicotine on the pharmacokinetics of levodopa. Clin. Neuropharmacol. 36 (2), 46–51. doi:10.1097/WNF.0b013e31827fd9cd
Lashuel, H. A., Overk, C. R., Oueslati, A., and Masliah, E. (2013). The many faces of α-synuclein: from structure and toxicity to therapeutic target. Nat. Rev. Neurosci. 14 (1), 38–48. doi:10.1038/nrn3406
Lee, H.-J., Kim, C., and Lee, S.-J. (2010). Alpha-Synuclein stimulation of astrocytes: potential role for neuroinflammation and neuroprotection. Oxidative Med. Cell. Longev. 3 (4), 283–287. doi:10.4161/oxim.3.4.12809
Lee, H.-W., Choo, M.-K., Bae, E.-A., and Kim, D.-H. (2003). Beta-glucuronidase inhibitor tectorigenin isolated from the flower of Pueraria thunbergiana protects carbon tetrachloride-induced liver injury. Liver Int. 23 (4), 221–226. doi:10.1034/j.1600-0676.2003.00830.x
Lee, T. Y., Yang, W., Cha, D. S., and Han, Y. T. (2023). Synthesis of a natural quinoline alkaloid isolated from the deep-sea-derived fungus and its potential as a therapeutic for Parkinson’s disease. J. Asian Nat. Prod. Res. 25 (5), 446–455. doi:10.1080/10286020.2022.2104259
Lemay, S., Chouinard, S., Blanchet, P., Masson, H., Soland, V., Beuter, A., et al. (2004). Lack of efficacy of a nicotine transdermal treatment on motor and cognitive deficits in Parkinson’s disease. Prog. Neuro-Psychopharmacology and Biol. Psychiatry 28 (1), 31–39. doi:10.1016/S0278-5846(03)00172-6
Li, H., Gao, C., Liu, C., Liu, L., Zhuang, J., Yang, J., et al. (2021). A review of the biological activity and pharmacology of cryptotanshinone, an important active constituent in danshen. Biomed. and Pharmacother. 137, 111332. doi:10.1016/j.biopha.2021.111332
Li, H., Li, Q., Liu, Z., Yang, K., Chen, Z., Cheng, Q., et al. (2017). The versatile effects of dihydromyricetin in health. Evidence-Based Complementary Altern. Med. eCAM 2017, 1053617. doi:10.1155/2017/1053617
Li, L., Gao, H., Lou, K., Luo, H., Hao, S., Yuan, J., et al. (2021). Safety, tolerability, and pharmacokinetics of oral baicalein tablets in healthy Chinese subjects: a single-center, randomized, double-blind, placebo-controlled multiple-ascending-dose study. Clin. Transl. Sci. 14 (5), 2017–2024. doi:10.1111/cts.13063
Li, M., Shi, A., Pang, H., Xue, W., Li, Y., Cao, G., et al. (2014). Safety, tolerability, and pharmacokinetics of a single ascending dose of baicalein chewable tablets in healthy subjects. J. Ethnopharmacol. 156, 210–215. doi:10.1016/j.jep.2014.08.031
Li, S.-Y., Wang, X.-B., and Kong, L.-Y. (2014). Design, synthesis and biological evaluation of imine resveratrol derivatives as multi-targeted agents against alzheimer’s disease. Eur. J. Med. Chem. 71, 36–45. doi:10.1016/j.ejmech.2013.10.068
Li, X., Zhang, G., Nie, Q., Wu, T., Jiao, L., Zheng, M., et al. (2017b). Baicalein blocks α-synuclein secretion from SN4741 cells and facilitates α-synuclein polymerization to big complex. Neurosci. Lett. 655, 109–114. doi:10.1016/j.neulet.2017.06.031
Li, X., Zhang, Y., Wang, Y., Xu, J., Xin, P., Meng, Y., et al. (2017a). The mechanisms of traditional Chinese medicine underlying the prevention and treatment of Parkinson’s disease. Front. Pharmacol. 8, 634. doi:10.3389/fphar.2017.00634
Liddelow, S. A., Guttenplan, K. A., Clarke, L. E., Bennett, F. C., Bohlen, C. J., Schirmer, L., et al. (2017). Neurotoxic reactive astrocytes are induced by activated microglia. Nature 541 (7638), 481–487. doi:10.1038/nature21029
Lim, K.-L., and Ng, C.-H. (2009). Genetic models of Parkinson disease. Biochimica Biophysica Acta (BBA) - Mol. Basis Dis. 1792 (7), 604–615. doi:10.1016/j.bbadis.2008.10.005
Liu, D., Mao, Y., Ding, L., and Zeng, X.-A. (2019). Dihydromyricetin: a review on identification and quantification methods, biological activities, chemical stability, metabolism and approaches to enhance its bioavailability. Trends Food Sci. and Technol. 91, 586–597. doi:10.1016/j.tifs.2019.07.038
Liu, L., Shen, Q., Bao, Y., Xu, F., Zhang, D., Huang, H., et al. (2023). Association between dietary intake and risk of Parkinson’s disease: cross-sectional analysis of survey data from NHANES 2007-2016. Front. Nutr. 10, 1278128. doi:10.3389/fnut.2023.1278128
Liu, M., Guo, H., Li, Z., Zhang, C., Zhang, X., Cui, Q., et al. (2020). Molecular level insight into the benefit of myricetin and dihydromyricetin uptake in patients with alzheimer’s diseases. Front. Aging Neurosci. 12, 601603. doi:10.3389/fnagi.2020.601603
Liu, Q., Zhu, D., Jiang, P., Tang, X., Lang, Q., Yu, Q., et al. (2019). Resveratrol synergizes with low doses of L-DOPA to improve MPTP-induced Parkinson disease in mice. Behav. Brain Res. 367, 10–18. doi:10.1016/j.bbr.2019.03.043
Liu, W., Zhang, W., Xing, L.-Z., Zhao, Y.-D., Xu, J., Li, R.-J., et al. (2023). 4-Arylidene curcumin derivatives in vitro inhibit α-synuclein aggregation and disaggregate the preformed fibril. Bioorg Med. Chem. 96, 117529. doi:10.1016/j.bmc.2023.117529
Liu, Y., Ho, L. H., Carver, J. A., and Pukala, T. L. (2011). Ion mobility mass spectrometry studies of the inhibition of alpha synuclein amyloid fibril formation by (-)-Epigallocatechin-3-Gallate. Aust. J. Chem. 64 (1), 36–40. doi:10.1071/CH10334
Liu, Y., Zhao, X., Zhang, Q., Wang, L., Li, Y., and Li, Y. (2020). Characterization and evaluation of the solubility and oral bioavailability of rutin-ethanolate solvate. AAPS Pharm. Sci. Tech. 21 (7), 241. doi:10.1208/s12249-020-01779-w
Lobbens, E. S., Breydo, L., Skamris, T., Vestergaard, B., Jäger, A. K., Jorgensen, L., et al. (2016). Mechanistic study of the inhibitory activity of geum urbanum extract against α-synuclein fibrillation. Biochimica Biophysica Acta (BBA) - Proteins Proteomics 1864 (9), 1160–1169. doi:10.1016/j.bbapap.2016.06.009
Lorenzen, N., Nielsen, S. B., Yoshimura, Y., Vad, B. S., Andersen, C. B., Betzer, C., et al. (2014). How epigallocatechin gallate can inhibit α-synuclein oligomer toxicity in vitro. J. Biol. Chem. 289 (31), 21299–21310. doi:10.1074/jbc.M114.554667
Lu, J.-H., Ardah, M. T., Durairajan, S. S. K., Liu, L.-F., Xie, L.-X., Fong, W.-F. D., et al. (2011). Baicalein inhibits formation of α-synuclein oligomers within living cells and prevents Aβ peptide fibrillation and oligomerisation. Chembiochem 12 (4), 615–624. doi:10.1002/cbic.201000604
Lu, J.-H., Tan, J.-Q., Durairajan, S. S. K., Liu, L.-F., Zhang, Z.-H., Ma, L., et al. (2012). Isorhynchophylline, a natural alkaloid, promotes the degradation of alpha-synuclein in neuronal cells via inducing autophagy. Autophagy 8 (1), 98–108. doi:10.4161/auto.8.1.18313
Lu, K.-T., Ko, M.-C., Chen, B.-Y., Huang, J.-C., Hsieh, C.-W., Lee, M.-C., et al. (2008). Neuroprotective effects of resveratrol on MPTP-induced neuron loss mediated by free radical scavenging. J. Agric. Food Chem. 56 (16), 6910–6913. doi:10.1021/jf8007212
Lu, L., Zhang, C., Cai, Q., Lu, Q., Duan, C., Zhu, Y., et al. (2013). Voltage-dependent anion channel involved in the α-synuclein-induced dopaminergic neuron toxicity in rats. Acta Biochimica Biophysica Sinica 45 (3), 170–178. doi:10.1093/abbs/gms114
Luan, Y., Ren, X., Zheng, W., Zeng, Z., Guo, Y., Hou, Z., et al. (2018). Chronic caffeine treatment protects against α-synucleinopathy by reestablishing autophagy activity in the mouse striatum. Front. Neurosci. 12, 301. doi:10.3389/fnins.2018.00301
Lücking, C. B., and Brice, A. (2000). Alpha-Synuclein and Parkinson’s disease. Cell. Mol. Life Sci. CMLS 57 (13–14), 1894–1908. doi:10.1007/PL00000671
Ludtmann, M. H. R., Angelova, P. R., Horrocks, M. H., Choi, M. L., Rodrigues, M., Baev, A. Y., et al. (2018). α-Synuclein oligomers interact with ATP synthase and open the permeability transition pore in Parkinson’s disease. Nat. Commun. 9 (1), 2293. doi:10.1038/s41467-018-04422-2
Luth, E. S., Stavrovskaya, I. G., Bartels, T., Kristal, B. S., and Selkoe, D. J. (2014). Soluble, prefibrillar α-synuclein oligomers promote complex I-dependent, Ca2+-induced mitochondrial dysfunction. J. Biol. Chem. 289 (31), 21490–21507. doi:10.1074/jbc.M113.545749
Ma, C., Liu, Y., Neumann, S., and Gao, X. (2017). Nicotine from cigarette smoking and diet and Parkinson disease: a review. Transl. Neurodegener. 6, 18. doi:10.1186/s40035-017-0090-8
Mandel, S., Maor, G., and Youdim, M. B. H. (2004). Iron and alpha-synuclein in the substantia nigra of MPTP-treated mice: effect of neuroprotective drugs R-apomorphine and green tea polyphenol (-)-Epigallocatechin-3-Gallate. J. Mol. Neurosci. MN 24 (3), 401–416. doi:10.1385/JMN:24:3:401
Marier, J.-F., Vachon, P., Gritsas, A., Zhang, J., Moreau, J.-P., and Ducharme, M. P. (2002). Metabolism and disposition of resveratrol in rats: extent of absorption, glucuronidation, and enterohepatic recirculation evidenced by a linked-rat model. J. Pharmacol. Exp. Ther. 302 (1), 369–373. doi:10.1124/jpet.102.033340
Medvedeva, M., Barinova, K., Melnikova, A., Semenyuk, P., Kolmogorov, V., Gorelkin, P., et al. (2020). Naturally occurring cinnamic acid derivatives prevent amyloid transformation of alpha-synuclein. Biochimie 170, 128–139. doi:10.1016/j.biochi.2020.01.004
Medvedeva, M., Kitsilovskaya, N., Stroylova, Y., Sevostyanova, I., Saboury, A. A., and Muronetz, V. (2022). Hydroxycinnamic acid derivatives from coffee extracts prevent amyloid transformation of alpha-synuclein. Biomedicines 10 (9), 2255. doi:10.3390/biomedicines10092255
Mehmood, S., Maqsood, M., Mahtab, N., Khan, M. I., Sahar, A., Zaib, S., et al. (2022). Epigallocatechin gallate: phytochemistry, bioavailability, utilization challenges, and strategies. J. Food Biochem. 46 (8), e14189. doi:10.1111/jfbc.14189
Menon, V. P., and Sudheer, A. R. (2007). Antioxidant and anti-inflammatory properties of curcumin. Adv. Exp. Med. Biol. 595, 105–125. doi:10.1007/978-0-387-46401-5_3
Mishima, E., Wahida, A., Seibt, T., and Conrad, M. (2023). Diverse biological functions of vitamin K: from coagulation to ferroptosis. Nat. Metab. 5 (6), 924–932. doi:10.1038/s42255-023-00821-y
Miyazaki, I., and Asanuma, M. (2020). Neuron-astrocyte interactions in Parkinson’s disease. Cells 9 (12), 2623. doi:10.3390/cells9122623
Mladěnka, P., Macáková, K., Kujovská Krčmová, L., Javorská, L., Mrštná, K., Carazo, A., et al. (2021). Vitamin K – sources, physiological role, kinetics, deficiency, detection, therapeutic use, and toxicity. Nutr. Rev. 80 (4), 677–698. doi:10.1093/nutrit/nuab061
Moore, D. J., West, A. B., Dawson, V. L., and Dawson, T. M. (2005). Molecular pathophysiology of Parkinson’s disease. Annu. Rev. Neurosci. 28, 57–87. doi:10.1146/annurev.neuro.28.061604.135718
Moore, K. S., Wehrli, S., Roder, H., Rogers, M., Forrest, J. N., McCrimmon, D., et al. (1993). Squalamine: an aminosterol antibiotic from the shark. Proc. Natl. Acad. Sci. U. S. A. 90 (4), 1354–1358. doi:10.1073/pnas.90.4.1354
Musgrave, I. F., Farrington, R. L., Hoban, C., and Byard, R. W. (2016). Caffeine toxicity in forensic practice: possible effects and under-appreciated sources. Forensic Sci. Med. Pathology 12 (3), 299–303. doi:10.1007/s12024-016-9786-9
Musial, C., Kuban-Jankowska, A., and Gorska-Ponikowska, M. (2020). Beneficial properties of green tea catechins. Int. J. Mol. Sci. 21 (5), 1744. doi:10.3390/ijms21051744
Mustapha, M., and Taib, C. N. M. (2021). MPTP-induced mouse model of Parkinson’s disease: a promising direction for therapeutic strategies. Bosnian J. Basic Med. Sci. 21 (4), 422–433. doi:10.17305/bjbms.2020.5181
Nabavi, S. F., Thiagarajan, R., Rastrelli, L., Daglia, M., Sobarzo-Sánchez, E., Alinezhad, H., et al. (2015). Curcumin: a natural product for diabetes and its complications. Curr. Top. Med. Chem. 15 (23), 2445–2455. doi:10.2174/1568026615666150619142519
Nagy, K., Redeuil, K., Williamson, G., Rezzi, S., Dionisi, F., Longet, K., et al. (2011). First identification of dimethoxycinnamic acids in human plasma after coffee intake by liquid chromatography-mass spectrometry. J. Chromatogr. A 1218 (3), 491–497. doi:10.1016/j.chroma.2010.11.076
Neshati, V., Mollazadeh, S., Fazly Bazzaz, B. S., Iranshahi, M., Mojarrad, M., Naderi-Meshkin, H., et al. (2018). Cardiogenic effects of characterized geum urbanum extracts on adipose-derived human mesenchymal stem cells. Biochem. Cell Biol. 96 (5), 610–618. doi:10.1139/bcb-2017-0313
Noyce, A. J., Bestwick, J. P., Silveira-Moriyama, L., Hawkes, C. H., Giovannoni, G., Lees, A. J., et al. (2012). Meta-analysis of early nonmotor features and risk factors for Parkinson disease. Ann. Neurology 72 (6), 893–901. doi:10.1002/ana.23687
Oertel, W. H., Müller, H.-H., Unger, M. M., Schade-Brittinger, C., Balthasar, K., Articus, K., et al. (2023). Transdermal nicotine treatment and progression of early Parkinson’s disease. NEJM Evid. 2 (9), EVIDoa2200311. doi:10.1056/EVIDoa2200311
Okoro, N. O., Odiba, A. S., Han, J., Osadebe, P. O., Omeje, E. O., Liao, G., et al. (2024). Ganoderma lucidum methyl ganoderate E extends lifespan and modulates aging-related indicators in Caenorhabditis elegans. Food and Funct. 15 (2), 530–542. doi:10.1039/D3FO04166B
Olsen, A. L., Clemens, S. G., and Feany, M. B. (2023). Nicotine-mediated rescue of α-synuclein toxicity requires synaptic vesicle glycoprotein 2 in Drosophila. Mov. Disord. 38 (2), 244–255. doi:10.1002/mds.29283
Ono, K., Hirohata, M., and Yamada, M. (2007). Anti-fibrillogenic and fibril-destabilizing activity of nicotine in vitro: implications for the prevention and therapeutics of Lewy body diseases. Exp. Neurol. 205 (2), 414–424. doi:10.1016/j.expneurol.2007.03.002
Ono, K., and Yamada, M. (2006). Antioxidant compounds have potent anti-fibrillogenic and fibril-destabilizing effects for alpha-synuclein fibrils in vitro. J. Neurochem. 97 (1), 105–115. doi:10.1111/j.1471-4159.2006.03707.x
Pacheco, C. R., Morales, C. N., Ramirez, A. E., Munoz, F. J., Gallegos, S. S., Caviedes, P. A., et al. (2015). Extracellular α-synuclein alters synaptic transmission in brain neurons by perforating the neuronal plasma membrane. J. Neurochem. 132, 731–741. doi:10.1111/jnc.13060
Paillusson, S., Gomez-Suaga, P., Stoica, R., Little, D., Gissen, P., Devine, M. J., et al. (2017). α-Synuclein binds to the ER–mitochondria tethering protein VAPB to disrupt Ca2+ homeostasis and mitochondrial ATP production. Acta Neuropathol. 134 (1), 129–149. doi:10.1007/s00401-017-1704-z
Pan, C.-H., Kim, E. S., Jung, S. H., Nho, C. W., and Lee, J. K. (2008). Tectorigenin inhibits IFN-Gamma/LPS-Induced inflammatory responses in murine macrophage RAW 264.7 cells. Archives Pharmacal Res. 31 (11), 1447–1456. doi:10.1007/s12272-001-2129-7
Pandey, N., Strider, J., Nolan, W. C., Yan, S. X., and Galvin, J. E. (2008). Curcumin inhibits aggregation of alpha-synuclein. Acta Neuropathol. 115 (4), 479–489. doi:10.1007/s00401-007-0332-4
Pang, H., Xue, W., Shi, A., Li, M., Li, Y., Cao, G., et al. (2016). Multiple-ascending-dose pharmacokinetics and safety evaluation of baicalein chewable tablets in healthy Chinese volunteers. Clin. Drug Investig. 36 (9), 713–724. doi:10.1007/s40261-016-0418-7
Pangeni, R., Sharma, S., Mustafa, G., Ali, J., and Baboota, S. (2014). Vitamin E loaded resveratrol nanoemulsion for brain targeting for the treatment of Parkinson’s disease by reducing oxidative stress. Nanotechnology 25 (48), 485102. doi:10.1088/0957-4484/25/48/485102
Park, K.-Y., Jung, G.-O., Choi, J., Lee, K.-T., and Park, H.-J. (2002). Potent antimutagenic and their anti-lipid peroxidative effect of kaikasaponin iii and tectorigenin from the flower ofPueraria thunbergiana. Archives Pharmacal Res. 25 (3), 320–324. doi:10.1007/BF02976633
Park, S.-S., Do, H.-A., Park, H.-B., Choi, H.-S., and Baek, K.-H. (2023). Deubiquitinating enzyme YOD1 deubiquitinates and destabilizes α-synuclein. Biochem. Biophysical Res. Commun. 645, 124–131. doi:10.1016/j.bbrc.2023.01.030
Perni, M., Flagmeier, P., Limbocker, R., Cascella, R., Aprile, F. A., Galvagnion, C., et al. (2018). Multistep inhibition of α-synuclein aggregation and toxicity in vitro and in vivo by trodusquemine. ACS Chem. Biol. 13 (8), 2308–2319. doi:10.1021/acschembio.8b00466
Perni, M., Galvagnion, C., Maltsev, A., Meisl, G., Müller, M. B. D., Challa, P. K., et al. (2017). A natural product inhibits the initiation of α-synuclein aggregation and suppresses its toxicity. Proc. Natl. Acad. Sci. U. S. A. 114 (6), E1009–E1017. doi:10.1073/pnas.1610586114
Peter, S., Jama, S., Alven, S., and Aderibigbe, B. A. (2021). Artemisinin and derivatives-based hybrid compounds: promising therapeutics for the treatment of cancer and malaria. Mol. Basel, Switz. 26 (24), 7521. doi:10.3390/molecules26247521
Pingili, R., Vemulapalli, S., Mullapudi, S. S., Nuthakki, S., Pendyala, S., and Kilaru, N. (2016). Pharmacokinetic interaction study between flavanones (hesperetin, naringenin) and rasagiline mesylate in wistar rats. Drug Dev. Ind. Pharm. 42, 1110–1117. doi:10.3109/03639045.2015.1115868
Polymeropoulos, M. H., Lavedan, C., Leroy, E., Ide, S. E., Dehejia, A., Dutra, A., et al. (1997). Mutation in the alpha-synuclein gene identified in families with Parkinson’s disease. Sci. (New York, N.Y.) 276 (5321), 2045–2047. doi:10.1126/science.276.5321.2045
Postuma, R. B., Aarsland, D., Barone, P., Burn, D. J., Hawkes, C. H., Oertel, W., et al. (2012a). Identifying prodromal Parkinson’s disease: pre-motor disorders in Parkinson’s disease. Mov. Disord. 27 (5), 617–626. doi:10.1002/mds.24996
Postuma, R. B., Anang, J., Pelletier, A., Joseph, L., Moscovich, M., Grimes, D., et al. (2017). Caffeine as symptomatic treatment for Parkinson disease (Café-PD): a randomized trial. Neurology 89 (17), 1795–1803. doi:10.1212/WNL.0000000000004568
Postuma, R. B., Lang, A. E., Munhoz, R. P., Charland, K., Pelletier, A., Moscovich, M., et al. (2012b). Caffeine for treatment of Parkinson disease: a randomized controlled trial. Neurology 79 (7), 651–658. doi:10.1212/WNL.0b013e318263570d
Prajapati, R., Park, S. E., Seong, S. H., Paudel, P., Fauzi, F. M., Jung, H. A., et al. (2021). Monoamine oxidase inhibition by major tanshinones from salvia miltiorrhiza and selective muscarinic acetylcholine M4 receptor antagonism by tanshinone I. Biomolecules 11 (7), 1001. doi:10.3390/biom11071001
Price, D. L., Koike, M. A., Khan, A., Wrasidlo, W., Rockenstein, E., Masliah, E., et al. (2018). The small molecule alpha-synuclein misfolding inhibitor, NPT200-11, produces multiple benefits in an animal model of Parkinson’s disease. Sci. Rep. 8, 16165. doi:10.1038/s41598-018-34490-9
Qi, H., and Li, S. (2014). Dose-response meta-analysis on coffee, tea and caffeine consumption with risk of Parkinson’s disease. Geriatrics and Gerontology Int. 14 (2), 430–439. doi:10.1111/ggi.12123
Quik, M. (2004). Smoking, nicotine and Parkinson’s disease. Trends Neurosci. 27 (9), 561–568. doi:10.1016/j.tins.2004.06.008
Quik, M., Perez, X. A., and Bordia, T. (2012). Nicotine as a potential neuroprotective agent for Parkinson’s disease. Mov. Disord. Official J. Mov. Disord. Soc. 27 (8), 947–957. doi:10.1002/mds.25028
Ramakrishna, N., Meeker, H. C., and Brown, W. T. (2016). Novel epigenetic regulation of alpha-synuclein expression in down syndrome. Mol. Neurobiol. 53 (1), 155–162. doi:10.1007/s12035-014-8979-z
Rao, M. N., Shinnar, A. E., Noecker, L. A., Chao, T. L., Feibush, B., Snyder, B., et al. (2000). Aminosterols from the dogfish shark Squalus acanthias. J. Nat. Prod. 63 (5), 631–635. doi:10.1021/np990514f
Rappold, P. M., Cui, M., Chesser, A. S., Tibbett, J., Grima, J. C., Duan, L., et al. (2011). Paraquat neurotoxicity is mediated by the dopamine transporter and organic cation transporter-3. Proc. Natl. Acad. Sci. U. S. A. 108 (51), 20766–20771. doi:10.1073/pnas.1115141108
Ren, B., Liu, Y., Zhang, Y., Zhang, M., Sun, Y., Liang, G., et al. (2017). Tanshinones inhibit hIAPP aggregation, disaggregate preformed hIAPP fibrils, and protect cultured cells. J. Mater. Chem. B 6 (1), 56–67. doi:10.1039/C7TB02538F
Ren, B., Zhang, Y., Zhou, H., Sun, F., Zhang, Z., Wei, Z., et al. (2015). Tanshinone IIA prevents the loss of nigrostriatal dopaminergic neurons by inhibiting NADPH oxidase and iNOS in the MPTP model of Parkinson’s disease. J. Neurological Sci. 348 (1–2), 142–152. doi:10.1016/j.jns.2014.11.026
Ren, X., and Chen, J.-F. (2020). Caffeine and Parkinson’s disease: multiple benefits and emerging mechanisms. Front. Neurosci. 14, 602697. doi:10.3389/fnins.2020.602697
Ren, Z.-L., Wang, C.-D., Wang, T., Ding, H., Zhou, M., Yang, N., et al. (2019). Ganoderma lucidum extract ameliorates MPTP-induced parkinsonism and protects dopaminergic neurons from oxidative stress via regulating mitochondrial function, autophagy, and apoptosis. Acta Pharmacol. Sin. 40 (4), 441–450. doi:10.1038/s41401-018-0077-8
Rong, J., Fu, F., Han, C., Wu, Y., Xia, Q., and Du, D. (2023). Tectorigenin: a review of its sources, pharmacology, toxicity, and pharmacokinetics. Molecules 28 (15), 5904. doi:10.3390/molecules28155904
Ruwizhi, N., and Aderibigbe, B. A. (2020). Cinnamic acid derivatives and their biological efficacy. Int. J. Mol. Sci. 21 (16), 5712. doi:10.3390/ijms21165712
Sandeep, S., Sahu, M. R., Rani, L., Kharat, A. S., and Mondal, A. C. (2023). Could vitamins have a positive impact on the treatment of Parkinson’s disease? Brain Sci. 13 (2), 272. doi:10.3390/brainsci13020272
Santos, T. B., de Moraes, L. G. C., Pacheco, P. A. F., dos Santos, D. G., Ribeiro, R. M., dos Moreira, C. S., et al. (2023). Naphthoquinones as a promising class of compounds for facing the challenge of Parkinson’s disease. Pharmaceuticals 16 (11), 1577. doi:10.3390/ph16111577
Schapira, A. H. V., Chaudhuri, K. R., and Jenner, P. (2017). Non-motor features of Parkinson disease. Nat. Rev. Neurosci. 18 (7), 435–450. doi:10.1038/nrn.2017.62
Selinsky, B. S., Zhou, Z., Fojtik, K. G., Jones, S. R., Dollahon, N. R., and Shinnar, A. E. (1998). The aminosterol antibiotic squalamine permeabilizes large unilamellar phospholipid vesicles. Biochimica Biophysica Acta (BBA) - Biomembr. 1370 (2), 218–234. doi:10.1016/S0005-2736(97)00265-4
Senkevich, K., and Gan-Or, Z. (2020). Autophagy lysosomal pathway dysfunction in Parkinson’s disease; evidence from human genetics. Park. and Relat. Disord. 73, 60–71. doi:10.1016/j.parkreldis.2019.11.015
Setoguchi, Y., Oritani, Y., Ito, R., Inagaki, H., Maruki-Uchida, H., Ichiyanagi, T., et al. (2014). Absorption and metabolism of piceatannol in rats. J. Agric. Food Chem. 62 (12), 2541–2548. doi:10.1021/jf404694y
Shao, Z., Xie, J., Jiang, J., Shen, R., Gui, Z., Li, H., et al. (2024). Research on topological effect of natural small molecule and high-performance antibacterial air filtration application by electrospinning. Sci. Total Environ. 909, 168654. doi:10.1016/j.scitotenv.2023.168654
Sharma, K., Fallon, S. J., Davis, T., Ankrett, S., Munro, G., Christopher, G., et al. (2022). Caffeine and attentional control: improved and impaired performance in healthy older adults and Parkinson’s disease according to task demands. Psychopharmacology 239 (2), 605–619. doi:10.1007/s00213-021-06054-9
Sharma, N., and Nehru, B. (2018). Curcumin affords neuroprotection and inhibits α-synuclein aggregation in lipopolysaccharide-induced Parkinson’s disease model. Inflammopharmacology 26 (2), 349–360. doi:10.1007/s10787-017-0402-8
Sharma, R. A., Steward, W. P., and Gescher, A. J. (2007). Pharmacokinetics and pharmacodynamics of curcumin. Adv. Exp. Med. Biol. 595, 453–470. doi:10.1007/978-0-387-46401-5_20
Shi, L., Zhang, T., Liang, X., Hu, Q., Huang, J., Zhou, Y., et al. (2015a). Dihydromyricetin improves skeletal muscle insulin resistance by inducing autophagy via the AMPK signaling pathway. Mol. Cell. Endocrinol. 409, 92–102. doi:10.1016/j.mce.2015.03.009
Shi, L., Zhang, T., Zhou, Y., Zeng, X., Ran, L., Zhang, Q., et al. (2015b). Dihydromyricetin improves skeletal muscle insulin sensitivity by inducing autophagy via the AMPK-PGC-1α-sirt3 signaling pathway. Endocrine 50 (2), 378–389. doi:10.1007/s12020-015-0599-5
Siddique, Y. H., Naz, F., Jyoti, S., Fatima, A., Khanam, S., Ali, F., et al. (2014). Effect of Centella asiatica leaf extract on the dietary supplementation in transgenic Drosophila model of Parkinson’s disease. Parkinson’s Dis. 2014, 262058. doi:10.1155/2014/262058
Simola, N., Morelli, M., and Carta, A. R. (2007). The 6-hydroxydopamine model of Parkinson’s disease. Neurotox. Res. 11 (3–4), 151–167. doi:10.1007/BF03033565
Simon, D. K., Swearingen, C. J., Hauser, R. A., Trugman, J. M., Aminoff, M. J., Singer, C., et al. (2008). Caffeine and progression of Parkinson disease. Clin. Neuropharmacol. 31 (4), 189–196. doi:10.1097/WNF.0b013e31815a3f03
Singh, D., Gupta, R., and Saraf, S. A. (2012). Herbs–are they safe enough? An overview. Crit. Rev. Food Sci. Nutr. 52 (10), 876–898. doi:10.1080/10408398.2010.512426
Singh, P. K., Kotia, V., Ghosh, D., Mohite, G. M., Kumar, A., and Maji, S. K. (2012). Curcumin modulates α-synuclein aggregation and toxicity. ACS Chem. Neurosci. 4 (3), 393–407. doi:10.1021/cn3001203
Smit, J. W., Basile, P., Prato, M. K., Detalle, L., Mathy, F., Schmidt, A., et al. (2022). Phase 1/1b studies of UCB0599, an oral inhibitor of α-synuclein misfolding, including a randomized study in Parkinson’s disease. Mov. Disord. 37 (10), 2045–2056. doi:10.1002/mds.29170
Sohrabi, T., Mirzaei-Behbahani, B., Zadali, R., Pirhaghi, M., Morozova-Roche, L. A., and Meratan, A. A. (2023). Common mechanisms underlying α-synuclein-induced mitochondrial dysfunction in Parkinson’s disease. J. Mol. Biol. 435 (12), 167992. doi:10.1016/j.jmb.2023.167992
Srivastava, T., Tyagi, D., Fatima, S., Sathyan, M. T. V., Raj, R., Sharma, A., et al. (2023). A natural small molecule-mediated inhibition of alpha-synuclein aggregation leads to neuroprotection in Caenorhabditis elegans. J. Neurochem. 168 (8), 1640–1654. doi:10.1111/jnc.15907
Sternke-Hoffmann, R., Peduzzo, A., Bolakhrif, N., Haas, R., and Buell, A. K. (2020). The aggregation conditions define whether EGCG is an inhibitor or enhancer of α-synuclein amyloid fibril formation. Int. J. Mol. Sci. 21 (6), 1995. doi:10.3390/ijms21061995
Sun, Q., Gong, T., Liu, M., Ren, S., Yang, H., Zeng, S., et al. (2022). Shikonin, a naphthalene ingredient: therapeutic actions, pharmacokinetics, toxicology, clinical trials and pharmaceutical researches. Phytomedicine 94, 153805. doi:10.1016/j.phymed.2021.153805
Sun, Y., Liu, S., Yang, S., Chen, C., Yang, Y., Lin, M., et al. (2021). Mechanism of dihydromyricetin on inflammatory diseases. Front. Pharmacol. 12, 794563. doi:10.3389/fphar.2021.794563
Takao, K., Toda, K., Saito, T., and Sugita, Y. (2017). Synthesis of amide and ester derivatives of cinnamic acid and its analogs: evaluation of their free radical scavenging and monoamine oxidase and cholinesterase inhibitory activities. Chem. and Pharm. Bull. 65 (11), 1020–1027. doi:10.1248/cpb.c17-00416
Takeshima, M., Murata, M., Urasoe, N., Murakami, S., Miyazaki, I., Asanuma, M., et al. (2011). Protective effects of baicalein against excess L-DOPA-induced dopamine quinone neurotoxicity. Neurological Res. 33 (10), 1050–1056. doi:10.1179/1743132811Y.0000000032
Tan, A. H., Lim, S. Y., and Lang, A. E. (2022). The microbiome–gut–brain Axis in Parkinson disease — from basic research to the clinic. Nat. Rev. Neurol. 18 (8), 476–495. doi:10.1038/s41582-022-00681-2
Tavassoly, O., Kakish, J., Nokhrin, S., Dmitriev, O., and Lee, J. S. (2014). The use of nanopore analysis for discovering drugs which bind to α-synuclein for treatment of Parkinson’s disease. Eur. J. Med. Chem. 88, 42–54. doi:10.1016/j.ejmech.2014.07.090
Temsamani, H., Krisa, S., Decossas-Mendoza, M., Lambert, O., Merillon, J.-M., and Richard, T. (2016). Piceatannol and other wine stilbenes: a pool of inhibitors against α-synuclein aggregation and cytotoxicity. Nutrients 8 (6), 367. doi:10.3390/nu8060367
Teng, Y., Zhao, J., Ding, L., Ding, Y., and Zhou, P. (2019). Complex of EGCG with Cu(II) suppresses amyloid aggregation and Cu(II)-Induced cytotoxicity of α-synuclein. Mol. Basel, Switz. 24 (16), 2940. doi:10.3390/molecules24162940
Thanvi, B. R., and Lo, T. C. N. (2004). Long term motor complications of levodopa: clinical features, mechanisms, and management strategies. Postgrad. Med. J. 80 (946), 452–458. doi:10.1136/pgmj.2003.013912
That, Q. T., Nguyen Thien, T. V., Dang, H. P., Le Hoan, N., Vo, L. K. T., Nguyen, M. H. D., et al. (2018). Chemical constituents of geum urbanum L. Roots. Nat. Prod. Res. 32 (21), 2529–2534. doi:10.1080/14786419.2018.1425844
Thelen, P., Scharf, J.-G., Burfeind, P., Hemmerlein, B., Wuttke, W., Spengler, B., et al. (2005). Tectorigenin and other phytochemicals extracted from leopard lily belamcanda chinensis affect new and established targets for therapies in prostate cancer. Carcinogenesis 26 (8), 1360–1367. doi:10.1093/carcin/bgi092
Thorne, N. J., and Tumbarello, D. A. (2022). The relationship of alpha-synuclein to mitochondrial dynamics and quality control. Front. Mol. Neurosci. 15, 947191. doi:10.3389/fnmol.2022.947191
Torpey, J., Madine, J., Wood, A., and Lian, L.-Y. (2020). Cyclophilin D binds to the acidic C-terminus region of α-synuclein and affects its aggregation characteristics. Sci. Rep. 10 (1), 10159. doi:10.1038/s41598-020-66200-9
Tripathi, T. (2020). A master regulator of α-synuclein aggregation. ACS Chem. Neurosci. 11 (10), 1376–1378. doi:10.1021/acschemneuro.0c00216
Tsukakoshi, K., Yoshida, W., Kobayashi, M., Kobayashi, N., Kim, J., Kaku, T., et al. (2018). Esterification of PQQ enhances blood-brain barrier permeability and inhibitory activity against amyloidogenic protein fibril formation. ACS Chem. Neurosci. 9 (12), 2898–2903. doi:10.1021/acschemneuro.8b00355
Tu, W., Zheng, C., Zheng, Y., Feng, Z., Lin, H., Jiang, Y., et al. (2023). The investigation of interaction and chaperon-like activity of α-synuclein as a protein in pathophysiology of Parkinson’s disease upon direct interaction with tectorigenin. Int. J. Biol. Macromol. 249, 125702. doi:10.1016/j.ijbiomac.2023.125702
Uéda, K., Fukushima, H., Masliah, E., Xia, Y., Iwai, A., Yoshimoto, M., et al. (1993). Molecular cloning of cDNA encoding an unrecognized component of amyloid in alzheimer disease. Proc. Natl. Acad. Sci. U. S. A. 90 (23), 11282–11286. doi:10.1073/pnas.90.23.11282
Veerendra Kumar, M. H., and Gupta, Y. K. (2002). Effect of different extracts of Centella asiatica on cognition and markers of oxidative stress in rats. J. Ethnopharmacol. 79 (2), 253–260. doi:10.1016/s0378-8741(01)00394-4
Vesely, O., Baldovska, S., and Kolesarova, A. (2021). Enhancing bioavailability of nutraceutically used resveratrol and other stilbenoids. Nutrients 13 (9), 3095. doi:10.3390/nu13093095
Villafane, G., Cesaro, P., Rialland, A., Baloul, S., Azimi, S., Bourdet, C., et al. (2007). Chronic high dose transdermal nicotine in Parkinson’s disease: an open trial. Eur. J. Neurology 14 (12), 1313–1316. doi:10.1111/j.1468-1331.2007.01949.x
Villafane, G., Thiriez, C., Audureau, E., Straczek, C., Kerschen, P., Cormier-Dequaire, F., et al. (2018). High-dose transdermal nicotine in Parkinson’s disease patients: a randomized, open-label, blinded-endpoint evaluation phase 2 study. Eur. J. Neurology 25 (1), 120–127. doi:10.1111/ene.13474
Wagner, J., Ryazanov, S., Leonov, A., Levin, J., Shi, S., Schmidt, F., et al. (2013). Anle138b: a novel oligomer modulator for disease-modifying therapy of neurodegenerative diseases such as prion and Parkinson’s disease. Acta Neuropathol. 125 (6), 795–813. doi:10.1007/s00401-013-1114-9
Wang, D., Yu, W., Cao, L., Xu, C., Tan, G., Zhao, Z., et al. (2020). Comparative pharmacokinetics and tissue distribution of cryptotanshinone, tanshinone IIA, dihydrotanshinone I, and tanshinone I after oral administration of pure tanshinones and liposoluble extract of salvia miltiorrhiza to rats. Biopharm. Drug Dispos. 41, 54–63. doi:10.1002/bdd.2213
Wang, Q., Yao, S., Yang, Z.-X., Zhou, C., Zhang, Y., Zhang, Y., et al. (2023). Pharmacological characterization of the small molecule 03A10 as an inhibitor of α-synuclein aggregation for Parkinson’s disease treatment. Acta Pharmacol. Sin. 44 (6), 1122–1134. doi:10.1038/s41401-022-01039-6
Wang, Q., Yu, X., Patal, K., Hu, R., Chuang, S., Zhang, G., et al. (2013). Tanshinones inhibit amyloid aggregation by amyloid-β peptide, disaggregate amyloid fibrils, and protect cultured cells. ACS Chem. Neurosci. 4 (6), 1004–1015. doi:10.1021/cn400051e
Wang, W., Qu, L., Cui, Z., Lu, F., Li, L., and Liu, F. (2023). Citrus flavonoid hesperetin inhibits α-synuclein fibrillogenesis, disrupts mature fibrils, and reduces their cytotoxicity: in vitro and in vivo studies. J. Agric. Food Chem. 71 (43), 16174–16183. doi:10.1021/acs.jafc.3c06816
Wang, X., Yang, Y., Liu, X., and Gao, X. (2020). Pharmacological properties of tanshinones, the natural products from salvia miltiorrhiza. Adv. Pharmacol. 87, 43–70. doi:10.1016/bs.apha.2019.10.001
Wang, Y. J., Pan, M. H., Cheng, A. L., Lin, L. I., Ho, Y. S., Hsieh, C. Y., et al. (1997). Stability of curcumin in buffer solutions and characterization of its degradation products. J. Pharm. Biomed. Analysis 15 (12), 1867–1876. doi:10.1016/s0731-7085(96)02024-9
Williams, R. B., Gutekunst, W. R., Joyner, P. M., Duan, W., Li, Q., Ross, C. A., et al. (2007). Bioactivity profiling with parallel mass spectrometry reveals an assemblage of green tea metabolites affording protection against human huntingtin and alpha-synuclein toxicity. J. Agric. Food Chem. 55 (23), 9450–9456. doi:10.1021/jf072241x
Wills, A.-M. A., Eberly, S., Tennis, M., Lang, A. E., Messing, S., Togasaki, D., et al. (2013). Caffeine consumption and risk of dyskinesia in CALM-PD. Mov. Disord. 28 (3), 380–383. doi:10.1002/mds.25319
Wu, J.-Z., Ardah, M., Haikal, C., Svanbergsson, A., Diepenbroek, M., Vaikath, N. N., et al. (2019). Dihydromyricetin and salvianolic acid B inhibit alpha-synuclein aggregation and enhance chaperone-mediated autophagy. Transl. Neurodegener. 8, 18. doi:10.1186/s40035-019-0159-7
Xilouri, M., Brekk, O. R., Landeck, N., Pitychoutis, P. M., Papasilekas, T., Papadopoulou-Daifoti, Z., et al. (2013a). Boosting chaperone-mediated autophagy in vivo mitigates α-synuclein-induced neurodegeneration. Brain A J. Neurology 136 (Pt 7), 2130–2146. doi:10.1093/brain/awt131
Xilouri, M., Brekk, O. R., and Stefanis, L. (2013b). α-Synuclein and protein degradation systems: a reciprocal relationship. Mol. Neurobiol. 47 (2), 537–551. doi:10.1007/s12035-012-8341-2
Xing, L., Tan, Z.-R., Cheng, J.-L., Huang, W.-H., Zhang, W., Deng, W., et al. (2017). Bioavailability and pharmacokinetic comparison of tanshinones between two formulations of salvia miltiorrhiza in healthy volunteers. Sci. Rep. 7, 4709. doi:10.1038/s41598-017-02747-4
Xiong, L., Zhao, P., Guo, Z., Zhang, J., Li, D., and Mao, C. (2010). Alpha-Synuclein gene structure, evolution, and protein aggregation. Neural Regen. Res. 5 (18), 1423–1428. doi:10.3969/j.issn.1673-5374.2010.18.011
Xu, B., Chen, J., and Liu, Y. (2022). Curcumin interacts with α-synuclein condensates to inhibit amyloid aggregation under phase separation. ACS Omega 7 (34), 30281–30290. doi:10.1021/acsomega.2c03534
Yáñez, J. A., Remsberg, C. M., Miranda, N. D., Vega-Villa, K. R., Andrews, P. K., and Davies, N. M. (2008). Pharmacokinetics of selected chiral flavonoids: hesperetin, naringenin and eriodictyol in rats and their content in fruit juices. Biopharm. and Drug Dispos. 29 (2), 63–82. doi:10.1002/bdd.588
Yang, B., Yang, Z., and Hao, L. (2023). Dynamics of a model for the degradation mechanism of aggregated α-synuclein in Parkinson’s disease. Front. Comput. Neurosci. 17, 1068150. doi:10.3389/fncom.2023.1068150
Yang, Z., Yao, Y., Zhou, Y., Li, X., Tang, Y., and Wei, G. (2023). EGCG attenuates α-synuclein protofibril-membrane interactions and disrupts the protofibril. Int. J. Biol. Macromol. 230, 123194. doi:10.1016/j.ijbiomac.2023.123194
Yao, Y., Tang, Y., and Wei, G. (2020). Epigallocatechin gallate destabilizes α-synuclein fibril by disrupting the E46-K80 salt-bridge and inter-protofibril interface. ACS Chem. Neurosci. 11 (24), 4351–4361. doi:10.1021/acschemneuro.0c00598
Yao, Y., Tang, Y., Zhou, Y., Yang, Z., and Wei, G. (2022). Baicalein exhibits differential effects and mechanisms towards disruption of α-synuclein fibrils with different polymorphs. Int. J. Biol. Macromol. 220, 316–325. doi:10.1016/j.ijbiomac.2022.08.088
Yoshida, W., Kobayashi, N., Sasaki, Y., Ikebukuro, K., and Sode, K. (2013). Partial peptide of α-synuclein modified with small-molecule inhibitors specifically inhibits amyloid fibrillation of α-synuclein. Int. J. Mol. Sci. 14 (2), 2590–2600. doi:10.3390/ijms14022590
Youdim, K. A., Qaiser, M. Z., Begley, D. J., Rice-Evans, C. A., and Abbott, N. J. (2004). Flavonoid permeability across an in situ model of the blood–brain barrier. Free Radic. Biol. Med. 36 (5), 592–604. doi:10.1016/j.freeradbiomed.2003.11.023
Yu, Y.-X., Yu, X.-D., Cheng, Q.-Z., Tang, L., and Shen, M.-Q. (2020). The association of serum vitamin K2 levels with Parkinson’s disease: from basic case-control study to big data mining analysis. Aging 12 (16), 16410–16419. doi:10.18632/aging.103691
Zarranz, J. J., Alegre, J., Gómez-Esteban, J. C., Lezcano, E., Ros, R., Ampuero, I., et al. (2004). The new mutation, E46K, of alpha-synuclein causes Parkinson and Lewy body dementia. Ann. Neurology 55 (2), 164–173. doi:10.1002/ana.10795
Zhang, H., Caprioli, G., Hussain, H., Le, N. P. K., Farag, M. A., and Xiao, J. (2021). A multifaceted review on dihydromyricetin resources, extraction, bioavailability, biotransformation, bioactivities, and food applications with future perspectives to maximize its value. eFood 2 (4), 164–184. doi:10.53365/efood.k/143518
Zhang, R., Xu, S., Cai, Y., Zhou, M., Zuo, X., and Chan, P. (2011). Ganoderma lucidum protects dopaminergic neuron degeneration through inhibition of microglial activation. Evidence-Based Complementary Altern. Med. eCAM 2011, 156810. doi:10.1093/ecam/nep075
Zhang, X., Liu, J., Pang, X., Zhao, J., and Xu, S. (2020). Curcumin suppresses aldosterone-induced CRP generation in rat vascular smooth muscle cells via interfering with the ROS-ERK1/2 signaling pathway. Evidence-Based Complementary Altern. Med. eCAM 2020, 3245653. doi:10.1155/2020/3245653
Zhao, D. F., Fan, Y. F., Wang, F. Y., Hou, F. B., Gonzalez, F. J., Li, S. Y., et al. (2021). Discovery and characterization of naturally occurring potent inhibitors of catechol- O-methyltransferase from herbal medicines. RSC Adv. 11 (17), 10385–10392. doi:10.1039/d0ra10425f
Zhao, J., Liang, Q., Sun, Q., Chen, C., Xu, L., Ding, Y., et al. (2017). (-)-Epigallocatechin-3-Gallate (EGCG) inhibits fibrillation, disaggregates amyloid fibrils of α-synuclein, and protects PC12 cells against α-synuclein-induced toxicity. RSC Adv. 7 (52), 32508–32517. doi:10.1039/c7ra03752j
Zhao, X., Wang, J., Hu, S., Wang, R., Mao, Y., and Xie, J. (2017). Neuroprotective effect of resveratrol on rotenone-treated C57bl/6 mice. Neuroreport 28 (9), 498–505. doi:10.1097/WNR.0000000000000789
Zheng, C.-Q., Fan, H.-X., Li, X.-X., Li, J.-J., Sheng, S., and Zhang, F. (2021). Resveratrol alleviates levodopa-induced dyskinesia in rats. Front. Immunol. 12, 683577. doi:10.3389/fimmu.2021.683577
Zheng, Z. V., Cheung, C. Y., Lyu, H., Chan, H. Y., Li, Y., Bian, Z. X., et al. (2019). Baicalein enhances the effect of low dose levodopa on the gait deficits and protects dopaminergic neurons in experimental parkinsonism. J. Clin. Neurosci. 64, 242–251. doi:10.1016/j.jocn.2019.02.005
Zhu, M., Rajamani, S., Kaylor, J., Han, S., Zhou, F. M., and Fink, A. L. (2004). The flavonoid baicalein inhibits fibrillation of alpha-synuclein and disaggregates existing fibrils. J. Biol. Chem. 279 (26), 26846–26857. doi:10.1074/jbc.M403129200
Zhu, Q., Zhuang, X.-X., Chen, J.-Y., Yuan, N.-N., Chen, Y., Cai, C.-Z., et al. (2021). Lycorine, a natural alkaloid, promotes the degradation of alpha-synuclein via PKA-mediated UPS activation in transgenic Parkinson’s disease models. Phytomedicine Int. J. Phytotherapy Phytopharm. 87, 153578. doi:10.1016/j.phymed.2021.153578
Zhu, X., and Jia, Y.-H. (2014). Inhibition of catechol-o-methyltransferase (COMT) by myricetin, dihydromyricetin, and myricitrin. Die Pharm. 69 (3), 183–186.
Keywords: Parkinson’s disease, α-Synuclein, natural products, aggregation, misfolding
Citation: Yang K, Lv Z, Zhao W, Lai G, Zheng C, Qi F, Zhao C, Hu K, Chen X, Fu F, Li J, Xie G, Wang H, Wu X and Zheng W (2024) The potential of natural products to inhibit abnormal aggregation of α-Synuclein in the treatment of Parkinson’s disease. Front. Pharmacol. 15:1468850. doi: 10.3389/fphar.2024.1468850
Received: 26 July 2024; Accepted: 08 October 2024;
Published: 23 October 2024.
Edited by:
Irina Ielciu, University of Medicine and Pharmacy Iuliu Hatieganu, RomaniaReviewed by:
Ankit Srivastava, National Institutes of Health (NIH), United StatesRita Catarina Gonçalves Perfeito, University of Coimbra, Portugal
Sunil Dutt Shukla, Government Meera Girls College, India
Copyright © 2024 Yang, Lv, Zhao, Lai, Zheng, Qi, Zhao, Hu, Chen, Fu, Li, Xie, Wang, Wu and Zheng. This is an open-access article distributed under the terms of the Creative Commons Attribution License (CC BY). The use, distribution or reproduction in other forums is permitted, provided the original author(s) and the copyright owner(s) are credited and that the original publication in this journal is cited, in accordance with accepted academic practice. No use, distribution or reproduction is permitted which does not comply with these terms.
*Correspondence: Haifeng Wang, aGZoZjE5OTlAc2luYS5jb20=; Xiping Wu, d3hwZG91ZG91QDE2My5jb20=; Wu Zheng, emhlbmcxMjN3dUAxNjMuY29t
†These authors have contributed equally to this work and share first authorship